Abstract
Disturbances in cardiac rhythm, also termed cardiac arrhythmias, can arise from structural heart disease, adverse drug interactions, or inherited gene variants in ion channels or the proteins that regulate them. Cardiac arrhythmias range from relatively common, chronic arrhythmias such as atrial fibrillation, to the rarer but more acutely dangerous ventricular arrhythmias that can culminate in ventricular fibrillation, which is rapidly lethal unless defibrillation is administered. Because ion channels and their regulators conduct electrical currents in the heart that determine cardiac rhythm, most anti-arrhythmic drugs act, directly or indirectly, on cardiac ion channels. As different types of cells in specific regions of the heart each carry their own signature array of ion channels, anti-arrhythmic drugs can be chosen to target the specific regions in which the arrhythmia originates or is most critically manifested. In this chapter, after a brief historical perspective, we review anti-arrhythmic drugs, first discussing them in the context of the widely used Singh-Vaughan Williams classification system. Next, after a description of the major arrhythmogenic mechanisms, we discuss in detail clinical application of the various classes of anti-arrhythmics. In the final section, we cover inherited cardiac channelopathies and how they have guided our understanding of cardiac physiology, pathophysiology and its treatment, before discussing pharmacogenetics of adverse drug interactions and ideas about future gene therapy approaches. The chapter is intended to be a guide to the major classes of anti-arrhythmic drugs, their usage and mechanisms of action, and, where appropriate, mechanistic insights into the ion channels that they target.
Chapter Outline
Singh-Vaughan Williams Classification of Antiarrhythmic Drugs
Sodium Channels and Class I Antiarrhythmic Drugs
β Receptors and Class II Antiarrhythmics
Potassium Channels and Class III Antiarrhythmic Drugs
Molecular Genetics of Arrhythmias
Gene Therapy Guided by Molecular Genetics of Inherited Arrhythmias
Historical Perspective
The heart, and more specifically the heartbeat, has throughout history served as an indicator of well-being and disease, both to the physician and to the patient. Through one’s own heartbeat, one can feel the physiologic manifestations of joy, thrills, fear, and passion; the rigors of a sprint or long-distance run; the instantaneous effects of medications, recreational drugs, or toxins; the adrenaline of a rollercoaster ride or a penalty shootout in a World Cup final. Although the complexities of the heart continue to humble the scientists and physicians who study it, the heart is unique in that, despite the complexity of its physiology and the richness of both visceral and romantic imagery associated with it, its function can be distilled down to that of a simple pump, the function and dysfunction of which we now understand a great deal about (see Chapter 24 ). The history of development of pharmacologic agents to correct abnormalities in heart rhythm is, however, emblematic of drug development as a whole: major successes combined with paradoxes, damaging side effects, and the often frustrating intransigence of what would seem the most intuitive targets for antiarrhythmics—ion channels.
In ancient Greek, Egyptian, and Chinese cultures, the pulse was recognized as a means to assess health, and for millennia it was the only measure of cardiac physiology and pathophysiology. In second-century Rome, Galen’s work De Pulsibus became the first great treatise on the pulse as a window into human health and established Galen as the father of his discipline. It was not for another 1500 years that the great physician, scientist, and naturalist William Harvey, of Folkestone in England, published his landmark (and then controversial) work An Anatomical Exercise Concerning the Motion of the Heart and Blood in Animals . This introduced the theory that blood circulates throughout the body, a hypothesis tested rigorously by Harvey with experiments on animals and the cadavers of executed criminals. In the late 19th and early 20th centuries, Waller and Einthoven founded the field of electrocardiography, facilitating quantitative differential diagnoses of cardiac arrhythmias. This paved the way for modern cardiology and advances such as Wolff, Parkinson, and White’s neurocardiac theory, the discovery of atrial fibrillation (AF), and the increasingly sophisticated molecular genotype-phenotype correlations from which we benefit today.
These latter advances also owe much to the work of molecular geneticists in the 1990s whose Herculean efforts (before routine high-throughput sequencing) helped identify the mutant ion channel genes underlying inherited arrhythmias such as long QT syndrome, working hand in hand with cellular electrophysiologists and physicians to exemplify the bedside-to-bench model of modern molecular medicine. Having said this, β blockers, the first antiarrhythmics of the modern era, were developed in the later 1950s to early 1960s not with ion channels in mind, but were a by-product of Sir James W. Black’s desire to create improved therapies for angina and essentially “to stop the effects of adrenaline on the heart” following the death of his father after a myocardial infarction. The resulting class II antiarrhythmic drug, propranolol, ushered in a new era of drug development and proved effective in therapy of disorders including angina, arrhythmia, and hypertension.
Despite the vast array of imaging, molecular, and electrocardiographic diagnostic tools available to cardiologists and other physicians, measurement of the pulse will likely be central to routine examination of the cardiac and holistic health of individuals for the foreseeable future. Timely, rhythmic beating of the heart is essential for health. When the heart develops sustained nonrhythmic beating, generally termed arrhythmia, the consequences can range from mild (e.g., syncope) to lethal (sudden cardiac death). Cardiac arrhythmias are treated by surgical ablation, electronic pacemaker, cardioversion, pharmacologic agents, or a combination of these, depending on the location and nature of the causal factor. For example, in the case of ventricular fibrillation—a life-threatening, chaotic tachycardia—electrical defibrillation is required immediately to prevent sudden cardiac death. In contrast, in many cases of AF there is an underlying structural defect in the atria that can be successfully treated using radiofrequency catheter ablation—for example, to prevent the aberrantly conducting area from being an arrhythmogenic focus.
This chapter focuses on antiarrhythmic medications as frequently encountered in the perioperative and critical care settings. These are a broad class of drugs, incorporating numerous structural classes and modes of action, that are used based on the suspected or known molecular etiology of the arrhythmia to be treated. Recent advances in the understanding of the molecular basis for generation of electrical currents in the heart, and also the genetic basis of many cardiac arrhythmias, have contributed to the development and use of antiarrhythmic drugs. Furthermore, these advances have demonstrated the basis for the proarrhythmic action of some drugs—paradoxically, even some drugs that are classified and used as antiarrhythmics.
To fully understand the mechanism of action of antiarrhythmic drugs, one must understand both the underlying cause of the arrhythmia, and the molecular target of the antiarrhythmic; these may or may not be the same entity. Accordingly, this chapter includes not only a description of the basic and clinical pharmacology of the major classes of cardiac arrhythmias, but also a discussion of the mechanistic underpinnings of these arrhythmias.
Basic Pharmacology
Singh-Vaughan Williams Classification of Antiarrhythmic Drugs
Antiarrhythmic drugs include a wide range of structural and functional classes. Although not perfect, a highly useful framework for their classification is that proposed by Singh and Vaughan Williams, usually referred to as the Singh-Vaughan Williams (SVW) classification . The SVW classification categorizes antiarrhythmic drugs into four classes ( Table 27.1 ). Class I denotes sodium (Na + ) channel blocking activity, with resultant delay in phase 0 depolarization and/or altered action potential duration. Class II agents counteract the effects of endogenous catecholamines (in particular epinephrine and norepinephrine) by antagonism of β adrenergic receptors, and hence are known as β blockers or β antagonists. Class III antiarrhythmics prolong the action potential and refractory period, acting primarily by potassium (K + ) channel blockade. Class IV agents reduce heart rate, primarily by L-type calcium (Ca 2+ ) channel blockade, which slows conduction through the sinoatrial (SA) and atrioventricular (AV) nodes. (For a more detailed discussion of cardiac electrophysiology and myocyte depolarization, see Chapter 23 ).
Class | Mechanism of Action | Drugs |
---|---|---|
Ia | Na + channel blockade, prolonged repolarization | Procainamide, quinidine, disopyramide |
Ib | Na + channel blockade, shortened repolarization | Lidocaine, mexiletine, phenytoin, tocainide |
Ic | Na + channel blockade, repolarization unchanged | Encainide, flecainide, propafenone |
II | β-adrenoceptor antagonist | Esmolol, metoprolol, propranolol |
III | Marked prolongation of repolarization | Amiodarone, bretylium, ibutilide, sotalol |
IV | Calcium channel blockade | Diltiazem, verapamil |
The primary reason that the SVW classification is to some extent imperfect is that many, if not most, antiarrhythmic drugs exhibit physiologically significant actions in more than one of the four classes. Typically the classification is based on the action that was first described for each drug, which is generally but not necessarily the most therapeutically significant mechanism of action for all types of arrhythmia for which the drug is prescribed. This discrepancy can occur because of nonspecificity of the drug, or because major metabolites of the drug exhibit different activity than that of the drug itself. Furthermore, some important antiarrhythmic drugs, including digoxin and adenosine, do not fit primarily into any of the four classes. However, the SVW classification is still the most useful framework for describing antiarrhythmic drugs, especially if one considers the net effect of each drug rather than all its specific molecular targets. The clinical pharmacology of the major antiarrhythmic drugs is summarized in Table 27.2 .
Class | Mechanism | Specific Drugs | Clinical Uses | Adverse Effects |
---|---|---|---|---|
Ia | Na + channel block (intermediate kinetics); K + channel block; repolarization prolonged | Quinidine Procainamide Disopyramide | Ventricular arrhythmias Prevention of paroxysmal recurrent atrial fibrillation Conversion of atrial flutter and fibrillation (quinidine, procainamide) Maintenance of sinus rhythm after conversion of atrial flutter and fibrillation Wolff-Parkinson-White syndrome (procainamide) | QTP/torsades de pointes, nausea, diarrhea, hepatotoxicity, myelosuppression |
Lethal ventricular arrhythmias, QTP/torsades de pointes, hypotension Lupus-like syndrome, blood dyscrasias | ||||
Proarrhythmic, torsades de pointes, negative inotropy Parasympatholytic | ||||
Ib | Na + channel block (fast kinetics); repolarization shortened | Lidocaine | Ventricular tachycardia/fibrillation (lidocaine) | Seizure, tremor, confusion/delirium |
Tocainide | ||||
Mexiletine | Atrial fibrillation | Ataxia, tremor | ||
Ic | Na + channel block (slow kinetics); no effect on repolarization | Flecainide | Prevention of paroxysmal atrial fibrillation Recurrent tachyarrhythmias of abnormal conduction system Contraindicated immediately after myocardial infarction | Proarrhythmic, heart failure |
Propafenone | Proarrhythmic, nausea | |||
II | β blockade Propranolol also shows class I effect | Nonselective Propranolol Nadolol β 1 selective Esmolol Metoprolol Atenolol Bisoprolol Nonselective β/α blockade Carvedilol Labetalol | Reduce myocardial infarction mortality Prevention of recurrence of tachyarrhythmias Rate control | Bradycardia, hypotension, fatigue, depression |
III | K + channel block Sotalol is also a β blocker Amiodarone and dronedarone have class I, II, and III activity | Sotalol Ibutilide Dofetilide Amiodarone Dronedarone Bretylium (no longer available in U.S.) | Wolff-Parkinson-White syndrome Ventricular tachycardia/fibrillation (sotalol bretylium, amiodarone) Conversion of atrial flutter and fibrillation (ibutilide, sotalol, dofetilide, amiodarone) Maintenance of sinus rhythm after conversion of atrial flutter and fibrillation (sotalol, dofetilide, amiodarone, dronedarone) | Proarrhythmic, QTP/torsades de pointes, bradycardia, heart failure |
Proarrhythmic, QTP/torsades de pointes | ||||
Proarrhythmic, QTP/torsades de pointes | ||||
Hypotension, bradycardia Pulmonary, neurologic, hepatic, dermatologic, ophthalmic, thyroid | ||||
Increased mortality with heart failure Nausea, vomiting, diarrhea | ||||
Proarrhythmic, hypotension | ||||
IV | Ca 2+ channel block | Dihydropyridine (selective vasodilators) Nifedipine, nicardipine, amlodipine, isradipine | Hypertension | |
Benzothiapine (less vasodilation; sinoatrial and atrioventricular node block) Diltiazem | Prevention of recurrent paroxysmal supraventricular tachycardia Reduce ventricular rate in atrial fibrillation | Bradycardia, hypotension, ankle swelling | ||
Phenylalkylamine (sinoatrial and atrioventricular node block) Verapamil | Prevention of recurrent paroxysmal supraventricular tachycardia Reduce ventricular rate in atrial fibrillation | Bradycardia, hypotension, constipation | ||
Other | Adenosine receptor activation | Adenosine | Supraventricular arrhythmias | |
Sodium pump inhibition | Digoxin | Heart failure, atrial fibrillation | Bradycardia, nausea, vomiting, visual disturbances | |
Ca 2+ channel block and other effects | Magnesium | Torsades de pointes | Hypotension, weakness |
Sodium Channels and Class I Antiarrhythmic Drugs
Voltage-gated Na + (Na v ) channels open in response to cell membrane depolarization to permit influx of Na + , further depolarizing the cell. The predominant Na v channel in human cardiac electrophysiology is Na v 1.5, which is encoded by the SCN5A gene. In most cardiac myocytes, Na v 1.5 activation mediates the upstroke in phase 0 of the action potential, in which the membrane potential is depolarized from around −70 mV to +20 mV as the result of Na + influx ( Fig. 27.1A ). Na v 1.5, like other mammalian Na v channels, inactivates rapidly, which together with the transient outward K + current, I to , shapes the notch in phase 1 at the beginning of the human cardiac myocyte action potential.

Class I antiarrhythmics exhibit Na v channel blocking activity. These drugs are often referred to as “membrane stabilizing agents” because by blocking cardiac Na v channels, which mediate myocyte depolarization, they reduce cellular excitability. Na v channels are composed of a 24-transmembrane-segment pore-forming α subunit that consists of four homologous domains (DI-DIV) and also bears four voltage-sensing domains and one or more inactivation gates ( Fig. 27.2A ). The Na v 1.5 α subunits form cardiac ion channel complexes with single-transmembrane segment β subunits, encoded by the SCNxB genes, which modify Na v 1.5 function and pharmacology.

Class I antiarrhythmic drugs are thought to bind in the inner pore vestibule of Na v channels, with drugs from different structural classes, including lidocaine, flecainide, and quinidine, and the anticonvulsant phenytoin, binding to an overlapping but nonidentical site influenced experimentally by mutations in the S6 transmembrane segment of domain IV. Drugs in class I are subcategorized as Ia, Ib, or Ic depending on their effects on Na + channel conduction and resultant effects on action potentials in cardiac myocytes expressing the predominant cardiac form of Na v channel, Na v 1.5 ( Fig. 27.2B ).
Na v 1.5 is relatively insensitive to the canonical and lethal Na v channel antagonist tetrodotoxin (TTX), a nerve toxin present, among other animal sources, in the nerves, skin, and gonads of the Japanese puffer fish, Fugu rubripes . F. rubripes is saddled with the dual distinction of having the shortest known genome of any vertebrate organism and being a delicacy in Japan that must be prepared by certified chefs to decrease the chances of ingestion of lethal doses of TTX by adventurous diners. Compared with its effects on Na v 1.5, TTX acts with up to 10 3 -fold higher potency on the predominantly neuronal Na v channel subtypes Na v 1.1-1.3 (SCN1A-3A) and the skeletal muscle–expressed channel Na v 1.4 (SCN4A) . TTX, nicknamed “zombie powder” because its paralysis- and coma-inducing effects have led to its use in voodoo ceremonies (it does not cross the blood-brain barrier; those ingesting sublethal doses are potentially conscious while paralyzed), is therefore not a useful antiarrhythmic (owing to its inefficacy and lethality at low doses). However, TTX prolongs the local anesthetic effect of bupivacaine when the two are coadministered. A recent high-resolution x-ray crystallographic structure of an Na v channel from Arcobacter butzleri has provided a first look at this class of proteins essential to function of nerve and muscle, and will likely enhance future class I antiarrhythmic development (see Chapter 20 ).
β Receptors and Class II Antiarrhythmics
Class II antiarrhythmic drugs, also known as β blockers, antagonize the β-adrenergic receptor (β receptor). This β blockade prevents activation of adenylyl cyclase and the consequent increase in intracellular cyclic adenosine monophosphate (cAMP), and thus also activation of its principal downstream target cAMP-dependent protein kinase (PKA), and the promotion of maximal myocardial performance that normally results from the enhancement of sympathetic nervous tone ( Fig. 27.3 ; see Chapters 13 and 14 ).

β Blockers are selective, in that they do not block other receptors, and specific, in that they do not antagonize cardiac stimulation and vasodilatation elicited by agents other than β agonists. All β blockers share the basic structure of a β sympathomimetic side chain, which confers affinity for the receptor, along with an aromatic substituent, which determines potency; most are derivatives of the class-defining agent propranolol (see Fig. 27.3B ). β Blockers are effective as antiarrhythmic agents because, by blocking the action of the sympathetic nervous system on the heart, they depress SA and AV node function, decrease conduction and automaticity, and prolong atrial refractory periods. β Blockers also reduce blood pressure, probably arising from a combination of reduced cardiac output, renal renin release, and perhaps even effects within the central nervous system (see Chapter 26 ). Inasmuch as an exaggerated increase in sympathetic tone typifies the deleterious reaction to congestive heart failure, β blockers, particularly the cardioselective ones, are used successfully also in the treatment of this ailment. Propranolol is marketed as a D,L -racemic mixture (see Chapter 1 for a discussion of the pharmacologic implications of chirality), the reason being that the L -form is the β blocker while the D -form is a “membrane stabilizer,” which adds antiarrhythmic effect to the β-blocking properties of the L -enantiomer.
Potassium Channels and Class III Antiarrhythmic Drugs
Class III antiarrhythmic drugs are defined by their ability to block K + channels. This activity increases action potential duration in cardiac myocytes and prolongs the refractory period—that is, it extends the period during which the heart is refractory to premature electrical stimuli. Cardiac K + channels exhibit a much wider variety than other cardiac ion channels. The two most physiologically and therapeutically important families of K + channels in the heart, based on current understanding, are the voltage-gated K + (K v ) channels and inward rectifier potassium (K ir ) channels. Channels in both these families are predominantly involved in the repolarization phases of the cardiac myocyte action potential, because both K v and K ir channels pass inward K + currents only when the cell membrane potential is negative to the K + equilibrium potential, which is around −80 mV under physiologic conditions.
K v channels are each composed of several subunit types. Similar to Na v channels, the pore-forming α subunits of K v channels are arranged in a 24-transmembrane-segment array forming an aqueous central pore with external voltage-sensing modules ( Fig. 27.4A ). However, in K v channels, this is composed of a tetramer of noncovalently linked α subunits (each subunit having six transmembrane segments) rather than one contiguous α subunit with four homologous six-segment domains as in Na v and Ca v channels. In K v channel α subunits, the fourth transmembrane segment (S4) bears the basic residues that confer voltage sensitivity, while S6 lines the pore ( Fig. 27.4A ). High-resolution x-ray crystallographic structures of bacterial and eukaryotic K v channels have revolutionized the study of these ubiquitous and essential proteins, including current understanding of drug binding sites.

The most important K v α subunits in human ventricular repolarization are the ether-à-go-go related gene product (hERG; also named KCNH2 ) and KCNQ1 . Tetramers of each of these coassemble with multiple single-transmembrane-domain ancillary or β subunits from the KCNE gene family. KCNQ1-KCNE1 complexes primarily generate the slowly activating I Ks current; hERG- KCNE2 complexes generate I Kr ; and each of the five KCNE proteins probably regulates these and other α subunits in the heart too. I Kr and I Ks are crucial to phase 3 repolarization (see Fig. 27.1A ); therefore blocking these currents delays ventricular repolarization, which can be proarrhythmic or antiarrhythmic depending on the disease state and other genetic and environmental factors. hERG is particularly sensitive to drug block by a wide range of drugs, owing to its bearing an unusual array of hydrophobic residues, not conserved among other K v channels, in an internal pore cavity also predicted to be wider than in other K v channels ( Fig. 27.4B ).
Inhibitors of K v channels tend to bind in one of three distinct sites: the outer vestibule, the inner vestibule (drugs in these classes would be considered pore blockers), or on the voltage sensor, which is rarer among small molecules but is seen with some toxins from venomous animals, such as hanatoxin and SGTx from tarantula spiders. The canonical inhibitors of hERG and KCNQ1 are E-4031 and chromanol 293B, respectively, both of which bind in the inner vestibule and show relatively high specificity for their targets. Additionally, both drugs are sensitive to the presence of KCNE subunits in complex with the α subunits. E-4031 will not be used in the future for antiarrhythmic therapy because pure hERG antagonists such as this cause dangerous repolarization delays. I Ks inhibitors are still being considered because it might be therapeutically useful to counteract the upregulation of I Ks during periods of high sympathetic activity associated with arrhythmia triggering. Other important K v channel targets of class III antiarrhythmic drugs include the K v 4 α subunits that generate human ventricular I to (particularly active during phase 1 of the ventricular action potential), and K v 1.5, which generates the ultrarapidly activating K + current, I Kur , important in atrial myocyte repolarization. K v 4 and K v 1.5 α subunits are also regulated by the KCNE subunits. In addition, all the K v channels discussed herein are regulated by a host of cytoplasmic β subunits and other regulatory proteins ( Fig. 27.4C ), each of which can affect channel pharmacology either directly or indirectly, because changes in gating alter drug binding kinetics.
K ir channels do not possess a voltage sensor but exhibit inward rectification because they are inhibited at more positive membrane potentials by intracellular constituents, including magnesium (Mg 2+ ) and polyamines such as spermine. These channels are composed of a tetramer of α subunits, each with only two transmembrane segments, around a central, aqueous, K + -selective pore ( Fig. 27.4D ). The ventricular inward rectifier current I K1 is generated by K ir 2 family α subunits and contributes to stabilizing the cardiac potential, passing current at either end but less so when the myocyte is strongly depolarized (see Fig. 27.1A ). The K ATP channel, which also probably contributes to cardiac excitability, is an octamer of four K ir 6 α subunits and four membrane-spanning sulfonylurea receptor subunits that render it sensitive to adenosine triphosphate (ATP). A host of class III drugs, including amiodarone and dofetilide, inhibit K ir channels by direct pore block within the inner vestibule.
Calcium Channels and Class IV Antiarrhythmics
Class IV agents slow AV nodal conduction, primarily by L-type Ca 2+ channel (LTCC) antagonism. LTCCs mediate the upstroke of nodal cell action potentials, unlike true atrial and ventricular myocyte action potentials in which the upstroke is mediated primarily by the faster activating and inactivating Na v channel, Na v 1.5. Hence, nodal action potentials exhibit a much slower upstroke than that of typical atrial and ventricular myocytes (see Chapter 23 ). The transmembrane topology of voltage-gated Ca 2+ channel (Ca v ) α subunits mirrors that of the Na v channel α subunits: 24 transmembrane segments around an aqueous pore. LTCCs also incorporate a requisite array of ancillary subunits: the cytoplasmic β subunit, transmembrane δ and γ subunits, and extracellular α 2 subunit ( Fig. 27.5A ). In cardiac muscle, the LTCC Ca v 1.2 α subunit is located in the T-tubules and is activated by cellular depolarization, via its voltage-sensing apparatus, which moves upon membrane depolarization and opens the discrete but connected pore. Ca 2+ influx through Ca v 1.2, down the Ca 2+ concentration gradient, helps depolarize the cell and increase cytosolic [Ca 2+ ] directly, but it also works indirectly by activating the sarcoplasmic reticulum–located ryanodine receptor (RyR2 in cardiac muscle) through Ca 2+ -activated Ca 2+ release. In skeletal muscle, the mechanism is somewhat different: Ca v 1.1 is mechanically linked to RyR1 and acts as the latter’s voltage sensor. Thus in skeletal muscle, RyR1 is activated primarily by membrane depolarization, with the Ca v 1.1 S4 domains acting as the RyR1 voltage sensors (see Fig. 27.5B ).

Most clinically relevant Ca v channel blockers are in one of three chemical classes: the dihydropyridines, which are not generally indicated for arrhythmias; the phenylalkylamines, exemplified by verapamil; and the benzothiazepines, exemplified by diltiazem. Diltiazem and verapamil (see Fig. 27.5C ) are thought to bind overlapping but distinct sites within the S6 segments of repeats III and IV, and the Ca 2+ selectivity filter of the α 1 subunit of the cardiac LTCC Ca v 1.2.
Clinical Pharmacology
Categories of Arrhythmogenic Mechanisms
The mechanistic bases for most, if not all, arrhythmias can be placed in one of three categories. These are discussed with the most common modes of treatment.
Automaticity
Arrhythmias in this category arise from changes to the normal process of automaticity essential for cardiac rhythm (see Fig. 27.1 ). They can be further separated into two subcategories:
Normal automaticity arrhythmias are those that elicit speeding or slowing of the heartbeat, initially at least maintaining regular beating, although this is lost at some point—for example, at extremely high heart rates, owing to an intrinsic inability of ion channels to function rapidly enough in concert. Arrhythmias in this category include sinus tachycardia and ventricular tachycardia (both being an increased heart rate). The most common pharmacologic treatment of sinus tachycardia and ventricular tachycardia is with β blockers (class II antiarrhythmics).
Abnormal automaticity arrhythmias are those in which regular activity is lost immediately and involve spontaneous impulse formation in partially depolarized cells (membrane potentials in the range of −40 to −60 mV). Examples of arrhythmias that can fall into this category include ventricular tachycardias and ectopic atrial tachycardias in the subacute phase (within 48 hours) following myocardial infarction, exercise-induced idiopathic ventricular tachycardias, and catecholamine-sensitive idiopathic ventricular tachycardias. The most common pharmacologic treatment of idiopathic rhythms and ectopic atrial tachycardias is with Ca 2+ channel blockers (class IV antiarrhythmics).
Sinus rhythm is regulated by pacemaker channels (i.e., hyperpolarization-activated, cyclic nucleotide-gated monovalent cation-nonselective channels known as HCN ), and to a greater or lesser extent, Ca 2+ oscillations. Hence, human HCN4 mutation is associated with sinus-mediated pathologic slowing of the heart rate (sinus bradycardia).
Triggered
Triggered arrhythmias are those in which a mistimed beat occurs before the previous beat is complete (see Fig. 27.1B ). The ion channels involved in orchestrating each cardiac myocyte action potential must work in concert to generate rhythmic contraction of the heart. Because the various classes of ion channels each have distinct gating kinetics and refractory periods, if one type of ion channel dysfunctions, it can act asynchronously with the others, potentially causing triggered arrhythmias. Triggered arrhythmias can be separated into two main classes:
Early after-depolarizations (EADs) occur when myocardial repolarization is delayed sufficiently that the next action potential in a given cardiac myocyte begins before that myocyte is fully repolarized. A common clinical consequence is the arrhythmia referred to as torsades de pointes (TdP). TdP is so named because it appears on the electrocardiogram (ECG) as a twisted ribbon owing to the variance in magnitude of the voltages associated with each heartbeat (see Fig. 27.1C ). TdP most often occurs because of pharmacologic inhibition of specific ventricular myocyte K + channels, which results in a delay in ventricular myocyte repolarization and consequent prolongation of the QT interval on the ECG. A number of drugs inhibit these channels and can lead to TdP, including several drugs commonly used in anesthesia; some of the major QT interval prolonging drugs are summarized in Table 27.3 . The QT interval represents the time from the onset of ventricular depolarization to the end of ventricular repolarization; prolongation of this interval can indicate long QT syndrome, of which there are now many well-defined subtypes with distinct molecular etiologies. When sufficiently long delays in repolarization occur, Na v channels can recover from their refractory period and open before repolarization is complete, leading to an EAD in phase 2, 3, or 4 of the ventricular or atrial action potential (see Fig. 27.1B ). The most common pharmacologic treatment of TdP is with magnesium sulfate, β blockers (class II antiarrhythmics), and/or Ca 2+ channel blockers (class IV antiarrhythmics).
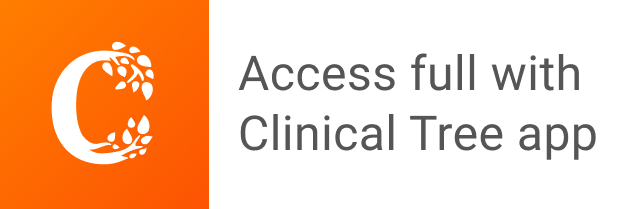