KEY POINTS
1. Three important channels characteristic of the circulation in utero allow preferential shunting of blood to sustain fetal viability. These include the ductus venosus, foramen ovale, and ductus arteriosus. A thorough understanding of the fetal circulation and transition to the adult circulation is an important aspect in understanding congenital heart disease (CHD).
2. There are significant structural differences in the infant’s heart compared to an adult heart that impact anesthetic management.
3. Most congenital cardiac defects can be assigned to one of three groups: Those resulting in increased pulmonary blood flow, decreased pulmonary blood flow, or obstruction to blood flow.
4. Cyanosis (associated with decreased pulmonary blood flow lesions) and congestive heart failure (CHF) (associated with increased pulmonary blood flow lesions and ventricular obstructive lesions) are the two major manifestations of CHD.
5. Neurologic sequelae remain one of the most common and potentially devastating complications of CHD and its repair.
6. All intravenous and monitoring tubing must be bubble-free whenever a potential shunt is present regardless of shunt direction. Intracardiac shunts can be bidirectional and shunt direction can be changed by anesthetic maneuvers such as positive-pressure ventilation.
7. For anesthetic induction and maintenance in children with CHD, the choice of drug(s) is not as important as an understanding of the lesion’s pathophysiology. Of help is the development of hemodynamic goals for each patient in terms of heart rate, contractility, preload, SVR, and PVR.
8. There are two methods regarding carbon dioxide management during cardiopulmonary bypass (CPB) (alpha-stat and pH-stat) that have significant implications for pediatric cardiac surgery.
9. Many cardiac lesions can be categorized based on the manifestations of cyanosis or CHF. However, this division is somewhat arbitrary as some lesions may have elements of both manifestations (e.g., transposition of the great arteries).
I. Introduction
The care of children undergoing cardiovascular surgery provides a remarkable challenge to the anesthesiologist. In the last decade, improvements in diagnostic capability, CPB techniques, monitoring, and perioperative care have permitted more complicated procedures to be performed on smaller, sicker children with remarkable success. The environment is dynamic, because operative procedures and technology are being modified constantly in an effort to further improve the safety and outcome for these special children.
Each child presents a unique set of circumstances and pathophysiologic concerns. Much of the knowledge regarding appropriate management for adults undergoing cardiac surgery will not apply to these children. Anesthesiologists caring for these patients must be flexible and innovative. Rigid protocols are rarely appropriate; instead, an individualized plan for each patient is mandatory. Team effort and good communication are essential to the success of a pediatric cardiovascular surgical program. Being a part of a successful team effort and caring for these patients are among the most exciting and rewarding experiences in medicine today.
1
A. Incidence. CHD is relatively uncommon. It is estimated to occur in less than 1% of all live births (Table 14.1). The true incidence is probably quite a bit higher. Much fetal wastage is thought to occur because of the presence of congenital heart defects that are incompatible with life. Also, some heart lesions (e.g., bicuspid aortic valve and patent ductus arteriosus [PDA]) may be relatively asymptomatic early in life; thus, the true incidence of CHD is unknown.
Table 14.1 Reported estimate of prevalence per 1,000 live births for specific congenital heart defects
Certain lesions are more likely to become manifest early in life than others. With these caveats in mind, the lesions most likely to be encountered in the first year of life are ventricular septal defect (VSD), transposition of the great vessels (TGV), tetralogy of Fallot (TOF), coarctation of the aorta, and hypoplastic left heart syndrome.
Different reference populations may demonstrate different patterns of CHD. For instance, infants who are premature and small for their gestational ages have an increased prevalence of CHD (especially VSD and PDA) compared to full-term newborns. Congenital heart defects are more common among infants of diabetic mothers than those of nondiabetic mothers. Infants with abnormal chromosomes have an increased frequency of congenital heart defects. About 23% to 56% of children with trisomy 21 have CHD [1]. The most common defects in children with Down syndrome appear to be VSD, atrial septal defect (ASD), PDA, and atrioventricular (AV) canal defects.
B. Prognosis. The outlook for these children today has improved considerably over that of previous years. A better understanding of the pathophysiology of individual lesions allows development of a rational treatment care plan. Earlier complete repairs are being performed successfully, often resulting in the avoidance of the long-term sequelae of unrepaired CHD. Cardiac transplantation also has become a viable option for some children whose lesions cannot be surgically repaired. For any of these options to be successful, the patient’s care must be thoughtfully individualized with vigilance, anticipation, and meticulous attention to detail. Close communication with the surgeon preoperatively and intraoperatively will result in better anticipation and, hopefully, avoidance of potential problems or management of real issues in the perioperative period.
II. Physiologic considerations
A. What is the difference between fetal and adult circulation?
To develop an understanding of the clinical and anesthetic implications of CHD, one must be familiar with the fetal and the adult circulations. Three important channels characteristic of the circulation in utero allow preferential shunting of blood: Ductus venosus, foramen ovale, and ductus arteriosus (Fig. 14.1).
Figure 14.1 Course of fetal circulation. See text for description. Ao, aorta; DA, ductus arteriosus; DV, ductus venosus; LA, left atrium; PT, pulmonary trunk; RA, right atrium; RV, right ventricle. (From Hoffman JIE. The circulatory system. In: Rudolph AM, ed. Pediatrics. 17th ed. Norwalk, CT: Appleton & Lange; 1982:1232, with permission.)
1. Ductus venosus. Well-oxygenated blood from the placenta, with a partial pressure of oxygen of about 33 mm Hg, travels through the umbilical vein to enter the liver. The ductus venosus allows about one-half of this blood to be shunted from the liver directly into the inferior vena cava.
2. Foramen ovale. About one-third of the blood entering the right atrium from the inferior vena cava is preferentially shunted across the foramen ovale into the left atrium. On the other hand, superior vena cava blood (which is poorly oxygenated) primarily enters the right ventricle (RV), with 2% to 3% crossing the foramen ovale.
3. Ductus arteriosus. RV blood is largely shunted across the ductus arteriosus into the descending aorta (rather than perfusing the high-resistance pulmonary circulation).
4. Implications. The structure of the fetal circulation allows the well-oxygenated blood (which has a high glucose content) from the inferior vena cava to preferentially perfuse the brain, coronary circulation, and upper extremities. The lower portion of the body receives blood with a low oxygen content from the ductus arteriosus. Hence, the systemic and pulmonary circulations in the fetus function in parallel, with each ventricle receiving only a portion of the systemic cardiac output (CO). The adult situation, in contrast, requires the two circulations to work in series, each processing the entire CO.
B. What is the transitional circulation?
At birth, remarkable changes occur rapidly in the circulation that allow the infant to adapt to the stresses of extrauterine life. A period of transition in the neonatal circulation occurs before permanent adaptation to the normal adult pattern takes place. This transitional stage is unstable and may exist for a few hours or for many weeks, depending on the stresses imposed. Factors contributing to the instability of the transitional circulation are the state of the ductus arteriosus, foramen ovale, and pulmonary vascular bed, as well as the immaturity of the neonatal heart. Conditions that may prolong the transitional circulation include hypoxia, hypothermia, acidosis, hypercarbia, sepsis, prematurity, and CHD.
1. Closure of the ductus arteriosus. Functional closure of the ductus arteriosus usually occurs within a few hours of birth, but anatomic closure may not occur for several weeks. During this period, the resistance to ductus arteriosus blood flow is responsive to changes in arterial PO2 (PaO2); that is, increased PaO2 increases resistance, and decreased PaO2 decreases resistance. Prostaglandin E1 (PGE1) infusion relaxes the ductal musculature and increases ductal flow, which may be left-to-right, right-to-left, or bidirectional. Maintenance of ductal patency may be important for the infant with cyanotic heart disease until repair or palliative surgery can be performed.
2. Closure of the foramen ovale and ductus venosus. The foramen ovale functionally closes when the left atrial pressure exceeds right atrial pressure; this usually occurs within a few hours after birth. Anatomic closure does not occur for many months, and about 30% of adults demonstrate probe patency of the foramen ovale. Right-to-left intracardiac shunting may occur across this area with coughing or the Valsalva maneuver or if pulmonary hypertension develops. Umbilical arteries and veins close shortly after birth, as does the ductus venosus. The latter forms the ligamentum venosum.
3. Pulmonary vascular resistance. Pulmonary vascular resistance (PVR) is high in utero but declines rapidly after birth in the term infant. Usually, it is lower than systemic levels within 24 h of birth. Thereafter, it falls at a moderate rate for 5 to 6 wks and then more gradually for the next 2 to 3 yrs. During this period, a child’s pulmonary vascular bed is more reactive than that of an adult, and a rise in pulmonary artery (PA) pressure can easily be produced by hypoxemia, hypercarbia, acidosis, or bronchospasm. If this reaction occurs shortly after birth, it may result in shunting across the ductus arteriosus or foramen ovale or other cardiac defects. Later in life, only a patent foramen ovale or cardiac defect remains as a possible shunt site.
2
C. How is a child’s heart structurally different from that of an adult?
1. Size. At birth, both ventricles are approximately equal in size and wall thickness. With the changeover from the fetal circulation, the left ventricle (LV) must accommodate a greater pressure and volume workload; conversely, the pressure load of the RV is reduced, and its volume work is only slightly increased. The LV hypertrophies in response to the increased workload and becomes roughly twice as heavy as the RV by about age 6 mos.
2. Ultrastructure. The neonate’s heart is ultrastructurally immature. Myofibrils are arranged in a disorderly fashion and have a smaller percentage of contractile proteins than those in the adult (30% vs. 60%). Autonomic innervation is incomplete at birth. The sympathetic innervation to the heart is decreased, as are cardiac catecholamine stores. In contrast, the parasympathetic innervation of the neonatal heart is comparable to that of the adult heart. These observations often are cited to explain the frequent vagal predominance that occurs in infants compared to adults. Sympathetic innervation also is immature in the peripheral vasculature. Therefore, control of vascular tone and myocardial contractility in infants depends largely on adrenal function and circulating or exogenously administered catecholamines. There are differences in myocardial calcium metabolism. In the mature myocardium, the sarcoplasmic reticulum is the predominant source of calcium ion for excitation–contraction coupling, but the sarcoplasmic reticulum is poorly developed in the immature heart. As the neonatal myocardial cell is deficient in T tubules that, in the mature myocardium, provide electrical coupling between the cell membrane and the sarcoplasmic reticulum, it is incapable of internal release and reuptake of calcium for contraction and instead depends on transmembrane calcium transport for the development of tension. These differences in calcium handling by the cell provide some explanation for the clinical observation that newborns require greater serum-ionized calcium levels for optimal myocardial contractility.
3. Compliance. The immature heart has a functionally decreased compliance compared to the adult heart. This difference reflects, in part, the ultrastructure of the heart and the increased volume load that each ventricle must handle with the transition from a parallel fetal to an adult series circulation. The RV and LV are more intimately inter-related as a result of this decreased compliance and similarity in size. Dysfunction of one ventricle quickly leads to biventricular failure. Reduced compliance also means that the immature heart is more sensitive to volume overload. A neonate’s ventricular function curve is shifted to the right and downward compared to that of an adult. Over the physiologic range of ventricular filling pressures, stroke volume changes are, in fact, small.
This relatively fixed stroke volume makes a neonate highly dependent on heart rate and sinus rhythm for optimal CO. In comparison, the adult heart is much more responsive to changes in preload to effect a change in stroke volume and thereby change CO. Increases in pressure work are poorly tolerated by both the right and left sides of the immature heart. The neonate, therefore, responds poorly to either volume or pressure loading, because resting cardiac function is on or near the plateau of the cardiac function curve.
D. How are congenital cardiac lesions meaningfully characterized?
3
1. Flow pattern. Patients with congenital heart defects are a diverse group. Rather than memorize an approach to each lesion, one should group the many anatomic varieties into a few understandable categories (Table 14.2). Most defects can be assigned to one of the three groups: (i) Those resulting in increased pulmonary blood flow, (ii) those resulting in decreased pulmonary blood flow, and (iii) those resulting in obstruction to blood flow. The first two groups feature an abnormal shunt pathway, whereas the third group has no shunting of blood. A fourth group could include lesions in which no pulmonary–systemic exchange of blood occurs (e.g., TGV). However, infants with TGV have either naturally occurring or artificially induced mixing of systemic and pulmonary venous returns and often can be classified into one of the first two groups, depending on whether obstruction to pulmonary blood flow is present.
Table 14.2 Flow characteristics of various congenital cardiac lesions
4
2. Clinical status: Cyanosis versus heart failure. Cyanosis and CHF are the major manifestations of CHD. Thus, the pathophysiologic classification must be related to the clinical status. Cyanosis occurs most commonly with lesions in which pulmonary blood flow is anatomically decreased or functionally decreased as mixing of systemic and pulmonary venous blood occurs.
CHF occurs most commonly in shunt lesions with excessively increased pulmonary blood flow or obstructive lesions that stress the ventricle beyond its capacity to pump effectively. Note that a child can be cyanotic but still fall into the category of having a lesion with increased pulmonary blood flow and may even manifest CHF. Two examples of such a situation are an infant with TGV and a large VSD or a child with truncus arteriosus. Even the most complex lesions usually fall into one of the three categories, even if they are characterized by mixed features (Table 14.2).
E. When and why do patients become symptomatic?
Many types of CHD may not be detected immediately after birth. The age at which heart defects become manifest depends on the type of lesion, its severity, and the state of the infant’s transitional circulation. Increased pulmonary blood flow lesions typically become symptomatic as PVR decreases and shunt flow to the lungs increases. These changes may take days to weeks to occur. Also, if the defect is small, it may remain asymptomatic.
Decreased pulmonary blood flow lesions often are detected earlier, usually because they result in significant cyanosis. If obstruction to pulmonary blood flow is severe, patients with such lesions may be dependent on left-to-right flow across their PDA. As the PDA closes in the first few days of life, hypoxemia becomes even more pronounced and may be incompatible with survival.
Infants with TGV and inadequate intracardiac communication become extremely cyanotic as their PDA closes. On the other hand, if a large VSD or PDA is present, these patients may develop excessive pulmonary blood flow as PVR decreases during the first few weeks of extrauterine life. Cyanosis will persist, however. Left untreated, hypertrophic vascular changes and pulmonary hypertension will occur.
Left-sided obstructive lesions cause pulmonary congestion without pulmonary volume overload. They impede flow from the pulmonary venous system to the systemic arterial system and can precipitate CHF. The symptomatology and age at presentation depend on the severity of the lesion. If the ductus arteriosus is patent, it allows right-to-left shunting of blood around the lesion, improving systemic perfusion but causing cyanosis.
III. Preoperative assessment
A. What should the anesthesiologist look for?
In developing an anesthetic plan for these children, one must understand the pathophysiology of the individual lesion and appreciate the degree of clinical symptomatology. As in any other assessment, taking a careful history and the physical examination probably are the most important parts of the preoperative evaluation. One must remember that the infant cannot relate the symptoms experienced, and the parents often fail to understand the significance of some of their observations.
1. Age at presentation. The age at presentation often provides a clue to the severity of the lesion. Infants with decreased pulmonary blood flow or inadequate mixing may be persistently cyanotic or may have intermittent episodes that often are associated with agitation, crying, or exercise. If a child is older, cyanotic episodes may be associated with “squatting” (which increases systemic vascular resistance [SVR] and promotes increased pulmonary blood flow). This change in pulmonary blood flow dynamics may partially alleviate the cyanosis. However, particularly severe episodes can result in loss of consciousness or seizures.
2. Frequency of episodes. The frequency of episodes suggests the severity of the lesion. Knowledge that cyanotic episodes are intermittent confirms the dynamic nature of the shunt and should alert one to the fact that the same scenario is probable during anesthesia and surgical manipulations. Alterations in SVR and PVR may result in profound changes in the magnitude of the right-to-left shunt.
a. Cyanosis. During the physical examination, an important consideration is that clinical cyanosis depends on the absolute concentration of deoxygenated hemoglobin in the blood rather than on the oxygen saturation. More than 3 g/dL of deoxygenated arterial blood hemoglobin should make central cyanosis recognizable. The oxyhemoglobin saturation at which central cyanosis becomes clinically apparent varies from about 62% when the hemoglobin level is 8 g/dL to about 88% in the polycythemic infant whose hemoglobin level is 24 g/dL. Therefore, cyanosis is detected more easily when the infant’s hematocrit (Hct) is elevated. However, recognition of cyanosis is more difficult if a newborn has a significant proportion of fetal hemoglobin because it is more highly saturated at a given PO2 (Fig. 14.2). Therefore, the newborn with a high proportion of fetal hemoglobin may have a large reduction in PO2 before central cyanosis is clinically apparent.
Figure 14.2 Hemoglobin–oxygen dissociation curves for fetal and adult hemoglobin. Note that an infant with a high proportion of fetal hemoglobin will have a low PaO2 (33 to 42 mm Hg) before cyanosis is observed. (From Lees MH, King DH. Heart disease in the newborn. In: Adams FH, Emmanouilides GC, Riemenschneider TA, eds. Heart Disease in Infants, Children and Adolescents. Baltimore, MD: Williams & Wilkins; 1989:844, with permission.)
Infants with a preductal coarctation of the aorta may demonstrate cyanosis that is restricted to the lower half of the body, because the RV supplies the descending aorta with deoxygenated blood via the PDA.
3. Respiration. Infants with cyanotic heart disease often have an increased tidal volume. Clubbing of the fingers may occur but may not be evident early in life. Children with decreased pulmonary blood flow usually have exercise intolerance. These patients also have a blunted ventilatory response to hypoxia.
4. CHF. Infants with too much pulmonary blood flow present with CHF early in infancy when PVR decreases. A history of feeding difficulties and failure to thrive are characteristics of CHF in infancy. Other features include tachypnea, tachycardia, irritability, inappropriate sweating (often with feeding), nasal flaring, sternal and intercostal retractions, cardiomegaly, and hepatomegaly. A history of wheezing, frequent respiratory infections, and pneumonia is common. The distinction between left-sided and right-sided heart failure in the newborn is less obvious than in the adult. Peripheral edema and rales are rarely present in the young infant. Systemic perfusion may be compromised, as evidenced by decreased pulses, pallor, and poor capillary refill. A severely compromised infant may be apathetic and have a weak cry and little spontaneous movement.
Left-sided obstructive lesions may cause CHF with clinical manifestations that are similar to those of pulmonary volume overload. Note, however, that the symptoms are a result of pulmonary venous congestion without abnormal shunting. If the lesion is located so that LV outflow is obstructed, LV hypertrophy will develop. If the site of obstruction involves the inflow to the LV, LV hypertrophy does not occur, and LV end-diastolic pressure is normal.
5. Associated anomalies. Look carefully for associated congenital anomalies, because they are common in newborns with cardiac disease. Other problems peculiar to the newborn or premature infant include difficulty with temperature regulation, impaired nutrition, susceptibility to dehydration and hypoglycemia, respiratory difficulties, coagulation abnormalities, and central nervous system disorders.
B. Which preoperative laboratory studies are helpful?
Laboratory studies of particular interest include Hct, white blood cell count, coagulation profile, and electrolyte and serum glucose determinations. Sickle-cell screening and measurement of digoxin level should be included when applicable.
1. Hct. The Hct progressively rises as hypoxemia becomes more profound. In fact, periodic checks of the Hct provide a simple method to follow the patient’s level of hypoxemia. Increasing Hct may serve as a cue for the appropriate timing of surgery for patients with complex cyanotic lesions. However, poor nutrition and iron deficiency in a hypoxemic child can prevent this increase in Hct and may mislead the clinician into thinking that the hypoxemia is less severe than it really is. A high Hct can result in increased blood viscosity, which can lead to spontaneous thrombosis and resultant cerebral, renal, or pulmonary infarctions. This process may be aggravated by the relative dehydration produced by a long period without oral intake. If the polycythemia is sufficiently severe (i.e., Hct is greater than 60% to 65%), phlebotomy may be required.
2. White blood cell count. Elevations in white blood cell count and a white blood cell shift in the differential should raise the suspicion of a systemic infection. Fever and upper respiratory infection must be ruled out. Children with elevated white blood cell counts should not be electively anesthetized, because immunologic function is compromised by CPB. Also, prosthetic material is frequently used in the surgical repair; if this material is inadvertently seeded by a bacteremia, the consequences may be disastrous.
3. Coagulation studies. Results of coagulation studies must be normal before CPB can be performed. A family history of bleeding tendencies should be sought. Unsuspected factor deficiencies have manifested and caused uncontrollable bleeding after surgery, when it may be difficult to identify the source of the problem. Patients with cyanotic CHD are prone to develop coagulopathies because of platelet dysfunction and hypofibrinogenemia.
4. Electrolytes. Electrolyte problems may be present in the newborn, especially if the child is receiving medication or total parenteral nutrition. Hypokalemia, hypomagnesemia, hypocalcemia, and hypoglycemia should be ruled out. Hypocalcemia is common in children with DiGeorge syndrome (a congenital disorder of the third and fourth branchial arches that is associated with thymic hypoplasia and congenital heart defects, especially aortic arch abnormalities or left-sided obstructive lesions).
5. Glucose. Hypoglycemia is especially common in infants with hypoplastic left-sided heart syndrome. The newborn’s myocardium has an increased glucose dependence compared to the adult myocardium; thus, hypoglycemia may aggravate myocardial failure. Hypoglycemia can occur because of reduced synthetic function, decreased glycogen stores, or reduced systemic perfusion, resulting in compromised hepatic function. Under anesthesia, hypoglycemia will be masked and may be missed unless the clinician looks for it. Conversely, these children often come to the operating room with concentrated dextrose in their hyperalimentation solution. Steroids are commonly administered on CPB, and this combination can result in significant hyperglycemia. Substantial literature exists, showing the detrimental effect of hyperglycemia during complete, incomplete, and focal cerebral ischemia in animals and adult humans. Specific data are lacking in children, although Steward et al. [2] suggested a worse neurologic outcome with hyperglycemia in a retrospective review of 34 children undergoing deep hypothermic circulatory arrest (DHCA). However, the Boston Circulatory Arrest Study suggested that normal blood glucose levels during reperfusion were associated with poorer neurologic outcome, whereas hyperglycemic levels appeared associated with better outcome [3]. It has been speculated that substrate deficiency in the immature brain may be an issue and that normal blood glucose levels during the reperfusion period after cerebral ischemia in infants may be insufficient for complete cerebral recovery.
6. Digoxin. Many children scheduled for heart surgery are receiving digoxin. After CPB, both a rebound increase in digoxin level and an increased sensitivity to the drug have been reported. Perioperative dysrhythmias are common and may be related to digoxin toxicity. Other factors may play a role in this enhanced toxicity, including hypokalemia, calcium fluxes, hypomagnesemia, and decreased creatinine clearance. Therefore, verify that the digoxin level is within the normal range and withhold digoxin preoperatively.
7. Sickling test. A sickling test should be performed in appropriate children. Hypothermia, acidosis, and anemia, as induced by CPB, decreased perfusion, and the bypass circuit itself enhance sickling if hemoglobin S is present. If the sickling test result is positive, hemoglobin electrophoresis should be performed to delineate the type of hemoglobinopathy. Depending on the type of defect, exchange transfusion may be indicated before or at the initiation of CPB.
8. Electrocardiography. The electrocardiogram shows great variability, especially during the first 24 h of life. In some instances, the electrocardiogram is diagnostically helpful. For example, extreme left- or right-axis deviation with counterclockwise frontal vector and RV hypertrophy suggests a form of endocardial cushion defect.
9. Echocardiography and cardiac catheterization. Two-dimensional echocardiography with quantitative Doppler and color flow mapping has revolutionized the diagnosis of CHD. In many institutions, the technology has become so refined that most surgical procedures are performed on the basis of this study alone. Cardiac catheterization may be used to confirm the diagnosis and to provide information concerning vascular resistance, the magnitude of shunts, and coronary anatomy. Cardiac catheterization may also be performed in conjunction with interventions such as device closures of septal defects.
10. Chest radiography. Chest radiography serves to evaluate the type and severity of the heart disease. It is also used to identify simulators of heart disease (e.g., meconium aspiration, mediastinal masses, pneumothorax, hyaline membrane disease, and diaphragmatic hernia) and to rule out significant pulmonary pathology.
C. What should the anesthesiologist tell the family about risk?
5
1. Neurologic sequelae. Morbidity and mortality vary with the lesion being repaired or palliated and with the institution involved. Ironically, as mortality has decreased, important morbidities have become more prominent. Neurologic sequelae remain one of the most common and potentially devastating complications of CHD and its repair. Early postoperative neurologic dysfunction may occur in as many as 25% of these children, with seizures occurring in approximately 20% of neonates after CPB. The seizures are generally self-limited, and some early series reported no long-term adverse sequelae. However, the group from Boston was the first to prospectively study a relatively homogeneous group of infants with TGV undergoing repair using either a predominantly low-flow CPB or DHCA strategy. They demonstrated that seizures are an important prognostic indicator of neurodevelopmental outcome [4]. The study also showed that there was a significantly higher incidence of seizures among infants randomized to circulatory arrest compared to those randomized to low-flow bypass. Follow-up of these children has shown a continuing important association between postoperative seizures and outcome, with an important decrement in cognitive and verbal skill assessments as well as motor skills [5]. Avoidance of circulatory arrest is not the entire solution to the problem, however, because the Boston group showed that there was a risk of seizures and suboptimal neurodevelopmental outcome even when continuous bypass was used.
Multiple factors contribute to the risk for neurodevelopmental sequelae. A complex interaction of preoperative, perioperative, and postoperative events can conspire to produce brain injury. Many children with CHD have pre-existing brain malformations. Multiple chromosomal anomalies with combined cardiac and neurologic features have been described, the best known being Down syndrome (trisomy 21) and the catch-22 spectrum of conditions associated with microdeletions in the 22q11 region of chromosome 22. Neurologic manifestations of the catch-22 spectrum may be subtle early in life but become more apparent over time. The potential impact of this chromosomal anomaly is enormous, because monosomy 22q11 has an estimated prevalence of 5% to 10% in the population of children with CHD. Brain injury also may be acquired in the preoperative period. Abnormal cardiovascular function may be associated with poor brain growth, embolic infarction, cerebrovascular thrombosis, and abscess formation. Hopefully, earlier repair of children with CHD will limit this mechanism of brain injury. Hypoxic–ischemic/reperfusion or embolic injury is thought to be the principal mechanism of brain injury occurring in the intraoperative period [6]. Injury also can occur in the postoperative period, during which unstable hemodynamics and increased cerebral energy needs may result in a mismatch of oxygen supply and demand to the brain. Intensive research is ongoing in this area of brain injury to try to prevent this devastating problem.
D. When should oral intake stop?
Nil per os (NPO) guidelines used for other infants and children generally can be used in patients with CHD. Recent evidence suggests that clear liquids can be continued until 2 to 4 h before surgery. In children with cyanotic heart disease, meticulous attention must be paid to the patient’s state of hydration. Specifically, orders must be written to awaken the child 2 h before surgery to offer clear liquids. If uncertainty exists concerning the precise time of surgery, place an intravenous catheter and begin an infusion to prevent dehydration in patients with cyanotic heart disease.
E. Which sedation is appropriate?
The need for sedation must be individualized, but certain guidelines can be offered. A thorough explanation to the patient and family is in order, because the parents’ anxiety often is transmitted to the child. Neonates and infants younger than 6 mos rarely require any sedation, because separation anxiety is not an issue. In older children, if intravenous access is already established and the child’s parents are allowed to accompany him or her to the preoperative holding area, additional sedation may be unnecessary, because incremental intravenous agents can be titrated before transfer to the operating room.
Children between the ages of 1 and 5 yrs benefit most from judicious sedation. A variety of agents and routes can be used. The author prefers to avoid intramuscular injections and uses either intravenous or oral midazolam. If given intravenously, titrate in 0.1 to 0.25 mg increments; if given orally, the author gives 0.5 mg/kg. Patient acceptance is improved if the drug is offered in sweetened apple juice. An oral dose of 0.5 mg/kg typically results in easy separation from the parents at 15 to 30 min. If given intranasally, 0.2 mg/kg will be effective at about 10 to 15 min. These patients must be monitored when sedation is given. Pulse oximetry and careful observation are mandatory, because the hemodynamic status may be adversely and unpredictably affected if hypercarbia or hypoxemia occurs.
Some physicians routinely administer anticholinergics preoperatively. Others give atropine in the operating room only if clinically necessary. Keep in mind that slow heart rates often are not tolerated in infants whose stroke volume is relatively fixed.
F. When does the patient need intravenous infusions?
Children who require vasoactive infusions preoperatively come to the operating room with such access already available. For others, the timing of intravenous access is strictly up to the anesthesiologist involved. For many cases, a gentle inhalation induction with subsequent expeditious venous catheter placement before intubation is appropriate. Again, if the timing of surgery is uncertain, preoperative intravenous catheter placement is desirable to avoid dehydration, especially in children with cyanotic heart disease.
G. When is prostaglandin E1 indicated?
PGE1 is indicated whenever it is thought that maintaining, reopening, or enlarging an existing ductus arteriosus will benefit the neonate. Common situations in which it is used include the presence of (i) lesions with decreased pulmonary blood flow, (ii) TGV, and (iii) left-sided heart outflow obstruction.
With TGV, the response to PGE1 is variable, but in some infants, mixing of systemic and pulmonary circulation improves sufficiently to reduce hypoxemia slightly and to relieve acidosis. With left-sided heart outflow obstruction (e.g., hypoplastic left-sided heart syndrome, coarctation), PGE1 will open the ductus and allow right-to-left flow across it, improving systemic perfusion and perhaps even coronary blood flow. It may also dilate the pulmonary vascular bed.
Stabilization of the infant before surgical intervention and improved outcome often result from PGE1 infusion. Side effects include apneic spells, seizures, systemic hypotension, inhibition of platelet aggregation, peripheral edema, and unexplained fever. Cortical proliferation in long bones can occur with chronic use. Because PGE1 is metabolized rapidly, it must be infused continuously, usually at a dose of 0.05 to 0.1 μg/kg/min. As much as 80% of circulating PGE1 is metabolized in one pass through the lungs; thus, the ductal response diminishes within minutes after its discontinuation.
IV. Equipment and infusions
A. What is required?
Care for an infant undergoing heart surgery demands meticulous attention to detail and extreme vigilance. A well-thought-out plan should be developed before induction so that all equipment needed is available and in working order (Table 14.3).
Table 14.3 Equipment used during pediatric cardiac surgery
1. Anesthetic and surgical considerations. The anesthesia machine and circuit should be checked as for all procedures. Multiple sizes of endotracheal tubes, masks, and laryngoscope blades must be available. Appropriate equipment to keep the infant warm may be needed, including a heating/cooling blanket, radiant warming lights, a fluid warmer, and a heated humidifier. A working operating room table that allows optimal positioning to facilitate surgical exposure is required. A defibrillator with both nonsterile (external) and sterile (internal) paddles, a dual-chamber pacemaker generator, a CO computer, and a coagulation analyzer must be operational. A fibrillator is frequently needed intraoperatively to induce ventricular fibrillation during open-chamber procedures.
2. Monitoring. Equipment needed to monitor the patient includes a pulse oximeter, a hemodynamic monitor, appropriate catheters for arterial and venous cannulation, transducers (zeroed and calibrated to mercury), a blood pressure cuff, a stethoscope, thermistors, and a mixed venous oxygen saturation monitor. Equipment used to monitor central nervous system function may include electroencephalography (EEG), transcranial Doppler (TCD), jugular venous saturation monitoring, and near-infrared spectroscopy. Transesophageal echocardiography has become an extraordinarily valuable tool for diagnostic purposes (confirmation and delineation of anatomy, detection of unsuspected defects or residual defects after repair) and for ventricular function and volume monitoring.
6
3. Intravenous fluids. Two intravenous fluid sets should be prepared, and all air bubbles should be removed from the tubing. This task is made easier if one begins with warm fluid; microbubble formation seems to occur less frequently than if cold fluid is allowed to warm when the room temperature is raised. All intravenous and monitoring tubing must be bubble-free whenever a potential shunt is present, because intracardiac shunts can be bidirectional and may become right-to-left during surgery. Air filters can be used, but the same amount of effort must be expended to remove air from intravenous tubing, because air filters cannot be relied on to trap all air. Another drawback of air filters is that they slow down intravenous infusions significantly and may make it difficult to keep up with volume replacement.
A method to carefully control and limit intravenous fluid intake is important, because many patients have barely compensated excess pulmonary blood flow and volume. Infusion using a limited amount in a buret chamber and a minidripper, use of infusion pumps with set volumes, or administration of fluid via syringe in bolus increments are methods that can be used to limit intake. At least three infusion pumps that allow accurate titration of vasoactive drugs should be available.
4. Preparation of infusions. Appropriate intravenous solutions for mixing infusions (e.g., normal saline and 5% dextrose in water) and cassettes for the pumps should be on hand. Common infusions to be available on short notice include sodium nitroprusside, epinephrine, isoproterenol, dopamine, and milrinone. Some thought should be given to the appropriate concentrations for the patient’s body size so that fluid overload can be minimized. Table 14.4 lists commonly used drugs and bolus doses or initial infusion rates.
Table 14.4 Nonanesthetic drugs and dosagesa
Children do not routinely require multiple vasoactive infusions postoperatively. I find it helpful to prepare commonly needed drugs in syringes so that a small bolus can be given if required. If needed repetitively, an infusion can be mixed. Table 14.5 lists drugs that should be available in syringes at the beginning of each pediatric cardiac surgical case. A narcotic, a benzodiazepine, and a muscle relaxant also should be on hand for ready use.
Table 14.5 Bolus drugs available in syringes
5. Blood and blood products. Blood products appropriate to the particular procedure and patient size should be readied in advance. Preparation may range from typing and screening for simple procedures to typing and cross-matching of multiple units of blood or platelets (or both) and fresh frozen plasma for complex pump cases. At the University of Florida, infants younger than 4 mos undergo typing and screening and then preferentially receive type O blood without a cross-match because the risk of transfusion reaction is low.
Transfusion-acquired cytomegalovirus infection is generally a benign entity in immunocompetent patients who receive blood. However, immunologically immature patients (especially low-birth-weight infants) can become symptomatic if infected. Therefore, our routine is to use cytomegalovirus-negative blood products in children younger than 4 mos. For infants with aortic arch abnormalities, blood products should be irradiated, because these cardiac lesions may be associated with DiGeorge syndrome. Such patients may have an absent thymus and increased susceptibility to graft-versus-host disease after transfusion.
V. Anesthetic induction and maintenance
A. Which monitors are needed before induction?
No absolute rule exists for determining the amount of monitoring necessary before induction. In some patients, particularly if a “steal” induction is ideal, anesthesia can be started with just a pulse oximeter and a precordial stethoscope. Then, as the patient is induced, electrocardiography and blood pressure monitoring can be quickly established. In others, it may be preferable to begin with all monitors (even invasive ones) in place. Generally, arterial and central venous catheters are placed after induction, although occasionally they may need to be placed before the procedure.
B. When does a patient need intravenous access before induction?
The timing of intravenous access, as noted previously, often is a matter of personal preference. However, polycythemic patients must be well hydrated either by mouth or intravenously. Children with extremely poor cardiac function who require inotropes may not tolerate an inhalation induction; thus, an intravenous induction is preferred. Most other pediatric patients tolerate a judicious inhalation induction with subsequent placement of intravenous catheters.
If myocardial reserve is impaired, a high-dose inhalation technique cannot be used for long; once catheters are placed, a transition is made either to a completely intravenous narcotic technique or to a combination of intravenous and inhalation techniques. If the anesthesiologist is uncertain about his or her ability to place an intravenous catheter rapidly during an inhalation induction, it should be inserted before induction.
C. How does cardiac disease affect the rate of induction?
1. Inhalation agents. Intracardiac shunting can alter the speed of induction with an inhalation anesthetic. The final effect on rate of induction depends on the size and direction of the shunt and the patient’s CO. A right-to-left intracardiac shunt prolongs induction, because of a slower rate of rise in systemic arterial blood anesthetic partial pressure. This rate of rise is slowed because of the dilution of the pulmonary blood flow containing anesthetic by the blood passing through the right-to-left shunt to which no anesthetic has been added. If high concentrations of agents are used to speed induction and a relative anesthetic overdose occurs, it is difficult to remedy, because the inhalation agents are slow to be eliminated. A left-to-right shunt, generally, has a negligible effect on the speed of induction if the systemic perfusion is preserved at a normal level.
2. Intravenous agents. Response to intravenously administered drugs is faster with a right-to-left shunt because the dilution effect and the pulmonary transit time are reduced in proportion to the magnitude of the shunt. A left-to-right shunt has a minimal effect on the response to intravenous drugs if systemic perfusion is preserved.
D. What problems are likely to occur during induction?
Any number of untoward events can occur during induction of anesthesia in pediatric patients with heart disease.
1. Airway obstruction. Airway obstruction is poorly tolerated in these patients, especially small infants or those with cyanotic heart disease. The margin for error is extremely small and minor problems can quickly become life threatening. Airway obstruction that causes hypoxemia or hypercarbia increases PVR. A reversal of a left-to-right intracardiac shunt or aggravation of a right-to-left shunt may result, exacerbating the problem and creating a vicious cycle.
2. Dysrhythmias. The patient may become bradycardic or develop a nodal rhythm with induction. As stroke volume is relatively fixed, CO suffers in this context. Acidosis can occur quickly when perfusion is marginal; this further depresses myocardial contractility, increasing PVR and decreasing SVR.
Dysrhythmias can result from many causes, including light anesthesia, hypoxemia, hypercarbia, drugs, and electrolyte abnormalities.
Significant potential problems may occur during central venous access acquisition. The drapes or patient position may serve to kink the endotracheal tube as it warms to body temperature. Dysrhythmias during this phase are generally induced mechanically from the catheter or guidewire. Familiarity with the lengths of the catheter kit components makes insertion to excessive depth less likely. Mechanically induced dysrhythmias respond better to removal of the stimulus than to pharmacologic therapy.
7
E. Which anesthetic technique should the anesthesiologist use?
The choice of drug(s) is not as important as an understanding of the lesion’s pathophysiology. Of help is the development of hemodynamic goals for each patient in terms of heart rate, contractility, preload, SVR, and PVR (Table 14.6). In several lesions, over-riding considerations dominate. An appropriate approach for a patient with aortic insufficiency may be completely different than that for a patient with TOF. Once the goals are defined, appropriate agents, dosages, and routes of administration can be selected. Many agents can be used so long as they are administered in a thoughtful fashion.
Table 14.6 Cardiac grid for common congenital heart diseases (desired hemodynamic changes)
F. How should the patient be positioned?
Data are scarce regarding the safest way to position a patient for heart surgery.
Access to the patient’s head is crucial for visual inspection to rule out superior vena cava syndrome, for pupil evaluation, and for airway manipulation. A piece of eggcrate foam can be placed under the patient’s head to minimize the chance of pressure necrosis; many heart surgery cases are lengthy, and low perfusion pressure occurs during CPB. However, because infants have such large occiputs, elevation of the head in this way occasionally may result in encroachment on the surgical field.
VI. Monitoring
Anesthetic induction generally proceeds with noninvasive monitors. A five-lead electrocardiograph is used to facilitate detection of rhythm disturbances and myocardial ischemia. An esophageal lead also can be used to more easily diagnose dysrhythmias, especially when tachycardia is present. Lead V5 can be placed in its normal position and covered with an adhesive drape to protect it from the surgical scrub solutions. The monitor mode of the electrocardiograph will minimize baseline drift, whereas the diagnostic mode allows better resolution of the P and T waves.
A. When is an arterial catheter indicated?
An indwelling arterial catheter is required whenever continuous monitoring of arterial pressure or frequent blood sampling is necessary. All procedures using CPB require placement of an arterial catheter, because noninvasive methods are not useful if no pulsatile flow is present. Most closed heart procedures also benefit from beat-to-beat monitoring of arterial pressure.
The arterial catheter is placed after intubation, preferably percutaneously in the nondominant radial artery. Other sites commonly used include the dorsalis pedis, posterior tibial, femoral, and, occasionally, temporal arteries. A surgical cutdown is used only as a last resort because of the disproportionately high incidence of thrombosis and infection. For coarctation repairs, the arterial catheter must be placed in the right radial artery; if this is unsuccessful, the catheter can be placed in a temporal artery. In patients with a Blalock–Taussig (BT) shunt, the catheter must be placed on the side opposite to the BT shunt or in a lower-extremity artery. A 22- or 24-gauge catheter is used, depending on the child’s size.
B. When is central venous access needed?
1. Central venous catheters. Central venous access is established routinely when knowledge of right-sided heart filling pressure trends or the need to administer vasoactive drugs rapidly is desirable. Access to the central circulation also allows placement of PA or pacing catheters. The decision as to which type and size of catheter should be used depends on the type of operation performed and on the size and clinical status of the patient.
A central venous catheter is used routinely in patients undergoing CPB. Monitoring superior vena caval pressure during extracorporeal circulation is useful for assessing adequacy of venous drainage, particularly when two venous cannulas are used. A central catheter with at least two lumens allows drug and fluid delivery via one lumen and uninterrupted central venous pressure (CVP) measurements via the other. Many centers avoid the use of SVC catheters in neonates because of the concern for venous thrombosis and SVC occlusion.
My guidelines for catheter length and size are listed in Table 14.7. Follow-up verification of appropriate catheter position occurs on review of the postoperative chest film.
Table 14.7 Guidelines for central venous pressure catheter size and length in relationship to patient size
2. PA catheters. Monitoring of PA pressure is helpful when PVR is problematic. Placement of the PA catheter can be accomplished percutaneously or by the surgeon using a direct transthoracic approach. If the patient’s size is too small to accept a balloon flotation catheter, a combined percutaneous and direct intraoperative approach can be used. In this scenario, the catheter is placed through a sterility sheath into the introducer and advanced into the superior vena cava. When the chest and heart are open, the surgeon can advance the catheter into the proximal PA. In complex cardiac anomalies with shunts, the surgeon generally will thread the percutaneously placed PA catheter into position at the end of the surgical procedure, because the catheter often is in the field of repair.
Continuous mixed venous oximetry is available with selected PA catheters and may be helpful in titrating vasoactive infusions, in providing an early warning of deteriorating CO, and in assessing residual shunts. The patient’s size must be large enough to accommodate a 5 to 6 Fr introducer to facilitate percutaneous placement of a PA catheter.
Smaller infants requiring PA pressure monitoring will have transthoracic PA catheters placed at the close of surgery. The PA catheter is also useful postoperatively to assess pressure gradients by carefully measuring pullback pressures upon its removal. Some PA catheters allow measurement of CO by thermodilution; however, this method is not used often in small children because of the significant fluid load it imposes on the patient.
3. Left atrial catheters. Left atrial pressure monitoring is commonly used in patients with CHD because disparities in left- and right-sided heart function often are present. This catheter is inserted by the surgeon at the end of repair, usually via the right superior pulmonary vein. One must be careful with its use; the risk of systemic embolization of clot or air is real because the catheter is in the left side of the heart. The risk of bleeding and cardiac tamponade is present when the catheter is removed.
C. Where should temperature be measured?
The optimal site for temperature monitoring during cardiac cases is controversial; remember that gradients exist between various sites (Fig. 14.3). Commonly used are the esophagus, nasopharynx, rectum, tympanic membrane, blood, and bladder. Temperature monitoring is important because the rate of cooling and rewarming appears to be important in the production of brain injury. Monitoring in at least two sites is advisable to ensure that the temperature gradient between inflow (blood temperature) and bladder or rectal temperature does not exceed 10°C and that uniform cooling and warming have occurred.
Figure 14.3 Average temperature (±SEM) of arterial cannula, myocardium, cerebral cortex, nasopharynx, and rectum during 40 min of cooling and 90 min of rewarming during CPB in six pigs. (From Stefaniszyn HJ, Novick RJ, Keith FM, et al. Is the brain adequately cooled during deep hypothermic cardiopulmonary bypass? Curr Surg. 1983;40:294, with permission.)
D. How do end-tidal and arterial carbon dioxide pressures correlate?
Monitoring of end-tidal carbon dioxide partial pressure (PETCO2) is useful to corroborate tracheal intubation. The arterial to end-tidal carbon dioxide partial pressure difference (P(a–ET)CO2) can be increased in patients with cardiopulmonary disease. It may also be increased in small children, depending on the sampling site and ventilatory pattern. Patients with CHD have altered ventilation/perfusion ratios; this produces abnormalities of both the physiologic dead space to tidal volume ratio (VDS/VT) and venous admixture (Qs/Qt).
In patients with cyanotic heart disease, in which Qs/Qt can be large, PETCO2 significantly underestimates PaCO2. As intracardiac shunting often is dynamic, the P(a–ET)CO2 is ever changing; thus, even PETCO2 trends are not reliable in these patients. Periodic measurement of PaCO2 is necessary to document adequate ventilation. PETCO2 monitoring during PA banding reflects the decrease in pulmonary blood flow. As the band is tightened, the P(a–ET)CO2 gradient increases.
8
E. How should blood gases be managed on cardiopulmonary bypass?
The strategy of management of the pH has received considerable attention over the past few years. Blood gas management strategy may be more important in children because greater degrees of hypothermia are often used, resulting in more profound differences in blood carbon dioxide levels. Briefly, there are two schools of thought regarding carbon dioxide management. In the alpha-stat strategy, no carbon dioxide is added to the circuit, and electrochemical neutrality is maintained with the blood gas measurement not corrected to temperature (i.e., reported at 37°C). Enzymatic function is well maintained in this milieu. In contrast, with the pH-stat strategy, carbon dioxide is added to the system to maintain a constant pH over varying temperatures. Blood gases are temperature corrected and reported at actual body temperature. In this scenario, hydrogen ions accumulate, total carbon dioxide stores are elevated, and the microcirculatory pH becomes increasingly acidotic at deep hypothermic temperatures. It was initially believed that intracellular pH also became increasingly acidotic, but more recent data have shown that the intracellular pH changes only slightly [7].
Evidence in adults suggests that either carbon dioxide management on CPB does not matter or that an alpha-stat strategy is advantageous. Recently, randomized prospective studies of adults using moderate hypothermia showed that postoperative neurologic or neuropsychologic outcome is slightly, but consistently, better with alpha-stat management [8].
Although acid–base management is probably not so important when moderate hypothermic temperatures are used, it may be critical in the setting of deep hypothermia. Investigations have been performed to try to understand the correct acid–base management approach in children, but controversy remains. Proponents of the alpha-stat method suggest that the luxuriously high blood flows seen with pH-stat management may put the brain at risk for damage because of microemboli, cerebral edema, or high intracranial pressure, or it may predispose to an adverse redistribution of blood flow (“steal”) away from marginally perfused areas in patients with cerebrovascular disease. On the other hand, proponents of the pH-stat strategy suggest that enhanced CBF may be helpful in improving cerebral cooling before the initiation of circulatory arrest. Total CBF is increased, global cerebral cooling is enhanced, and a redistribution of brain blood flow occurs during pH-stat management. An increased proportion of CBF is distributed to deep brain structures (thalamus, brainstem, and cerebellum) when pH-stat management is used [9]. However, other data suggest that cerebral metabolic recovery after circulatory arrest may be better with the alpha-stat method than with the pH-stat mode. This variation in results has led some authors to advocate a crossover strategy, that is, using a pH-stat approach during the first 10 min of cooling to provide maximal cerebral metabolic suppression followed by a change to alpha-stat strategy to remove the severe acidosis that accumulates during profound hypothermia with the use of pH-stat. This approach appears to offer maximal metabolic recovery in animals (Fig. 14.4) [10]. Choice of acid–base management may be particularly important in the subgroup of patients with aortopulmonary collaterals where cerebral cooling is problematic. It appears that addition of carbon dioxide during cooling enhances cerebral perfusion and improves cerebral metabolic recovery [11]. A randomized, single-center trial in human infants younger than 9 mos found that those managed with a pH-stat strategy generally had better outcomes than those in the alpha-stat group [12]. The pH-stat infants had a significantly shorter recovery time to first EEG activity and a tendency to fewer EEG-manifested seizures. In the subset of transposition babies, those assigned to pH-stat tended to have a higher cardiac index despite a lower requirement for inotropic agents, less-frequent acidosis and hypotension, and a shorter duration of mechanical ventilation and intensive care unit stay. The data suggest that pH-stat management may enhance systemic and cerebral protection in this group of patients.
Figure 14.4 Effects of three different cooling strategies: alpha-stat, pH-stat, and a cross-over of pH-stat followed by alpha-stat on cerebral metabolic suppression before DHCA and recovery of cerebral metabolism after DHCA. The pH-stat strategy provides better metabolic suppression before DHCA than alpha-stat, but cerebral metabolic recovery after DHCA is poor. Initial cooling with a pH-stat strategy followed by conversion to alpha-stat before DHCA results in the greatest cerebral metabolic recovery after DHCA. Yellow bar, 37°C; Pink bar, 13°C; Blue bar, after DHCA. **Percent metabolic recovery significantly better for crossover strategy than either the alpha- or pH-stat strategy alone. (From Kern FH, Greeley WJ. pH-stat management of blood gases is not preferable to alpha-stat in patients undergoing brain cooling for cardiac surgery. J Cardiothorac Vasc Anesth. 1995;9:215, with permission.)
Why the apparent difference in outcome between adults and children relative to pH management? It may be related to differences in the mechanism of brain injury on CPB. In adults, emboli appear to play a prominent role in adverse neurologic outcome. It is postulated that the decrease in CBF associated with alpha-stat management might be protective by limiting cerebral microemboli. On the other hand, the mechanism of injury in children may relate more to hypoperfusion or activation of excitotoxic pathways [13]. If a pH-stat strategy is used, the increase in CBF may be beneficial in ensuring complete brain cooling and slowing oxygen consumption, thus increasing the brain’s tolerance for DHCA.
F. How should the central nervous system be monitored?
Central nervous system insults associated with cardiac surgery remain an unsolved problem. Brain damage can occur as a result of global hypoxia–ischemia or focal emboli. EEG has been used to try to provide a measure of cerebral electrical activity and function during cardiac surgery. Unfortunately, the EEG may not be reliable in predicting or preventing brain ischemia during CPB because of the effects of hypothermia and anesthetic agents and because of the likelihood of focal embolic injury. Newer, computerized, processed EEG monitors are less cumbersome and allow easier recognition of trends and abrupt changes. The advantage of EEG is that it can be obtained in patients of any age or size with no risk and may be effective in detecting catastrophic events causing global ischemia. EEG can also be useful for assessing adequacy of cerebral cooling by ensuring electrocerebral silence before circulatory arrest.
CBF has been measured using the Fick principle and xenon (Xe) 133 clearance. Unfortunately, this technique does not provide continuous measurement of CBF and is more applicable to the research setting rather than clinical care. TCD sonography uses the Doppler principle to detect shifts in frequency of reflected signals from blood in motion to calculate flow velocity. As it is thought that the diameters of the large cerebral arteries insonated are relatively constant, trends in flow velocity should pattern those of CBF. Thus, even though quantitative measurement of CBF cannot be made from TCD, qualitative inferences may be appropriate. TCD can provide an indirect measure of cerebral vascular resistance (which is increased with elevated intracranial pressure or markedly elevated CVP). TCD can be helpful in detecting suboptimally placed cannulae, because distortion of the superior vena cava can result in an impediment to cerebral venous drainage. TCD is also useful for detecting cerebral emboli. At present, the technology does not allow determination of emboli type (air vs. particulate) or size.
Cerebral metabolism has been estimated by monitoring jugular venous bulb saturation, the cerebral equivalent of “mixed venous” blood. A low saturation suggests an elevation in cerebral metabolism outstripping the cerebral oxygen provided. However, this blood is the effluent from many areas of the brain, and regional areas of cerebral hypoperfusion can easily be missed.
Challenges in monitoring the neonatal cardiac surgical patient while in the operating room are the result of an interplay between several ongoing variables. Metrics employed to assess CO, adequacy of CPB, changes in metabolic demand associated with temperature changes, and ongoing inflammatory responses all create significant difficulty in determining how to best monitor a patient undergoing surgical intervention. Many institutions and programs have begun to employ near infrared spectroscopy (NIRS) as a tool to best monitor cerebral and peripheral oxygenation [14]. Assessment of perfusion in the operating room setting has historically relied upon an indirect measurement of CO using parameters such as blood pressure, pulses, capillary refill, and urine output. While on CPB, perfusion pressure and the ability to control the patient’s core temperature are often times the only sources of information regarding adequate CPB pump functionality. We have found that continuous monitoring of the regional oxygen saturation (rSO2) via NIRS allows for identification of acute changes in cerebral and systemic oxygen delivery and frequently precedes other indicators of decreased CO. Sensors are often placed on the forehead to look at cerebral oxygenation as well as over the kidney for a measure of somatic perfusion. This is helpful not only prior to and following the use of CPB, but also while the child is on CPB as a method of ensuring adequate cerebral perfusion as well as drainage. In addition, the postoperative patient can be expected to have lability with regard to CO, and the need for acute interventions should be anticipated because critical low-output syndrome may develop. Strategies for postoperative care are predicated upon optimizing rSO2 and CO. As such, an algorithmic and reproducible approach to initial medical management in the perioperative and immediate postoperative periods minimizes the potential for these predictable and necessary interventions to result in morbidity or mortality. Neurologic dysfunction can be a problem in patients with CHD. NIRS may provide data on cerebral oxygenation, and enthusiasm for this technology has increased with hopes of reducing neurologic dysfunction. Many centers have adopted NIRS as standard of care. Available data suggest that multimodality monitoring, including NIRS, may be a useful adjunct. However, the current literature on the use of NIRS alone does not conclusively demonstrate improvement in neurologic outcome. Data correlating NIRS findings with indirect measures of neurologic outcome or mortality are limited. Although NIRS has promise for measuring regional tissue oxygen saturation, the lack of data demonstrating improved outcomes limits the support for wide-spread implementation [15].
As neurologic morbidity is such an issue, I believe that neurologic monitoring will become more prevalent and techniques will be refined over the next decade. Anesthesiologists will become more skilled in “pattern recognition” of scenarios that require intervention.
G. What is the role of echocardiography?
Perioperative echocardiography is increasingly used in many centers for pediatric cardiac surgery. Both epicardial and, more recently, transesophageal studies have been performed to better define cardiac anatomy and assess surgical repair. Technologic improvements allow better imaging, smaller probe size, and multiplane capability, thereby substantially increasing the information provided by this modality. Several studies have demonstrated that two-dimensional and Doppler color-flow imaging can demonstrate previously unappreciated anatomic details and allow assessment of quality of repair and ventricular function after bypass. The ultimate role of echocardiography in the operating room and intensive care unit is still evolving and will hinge on demonstration of improvement in outcome in these patients.
VII. Cardiopulmonary bypass
A. How does it differ in children?
Although the physiology of extracorporeal circulation is similar in adults and children, significant differences exist in technique and physiologic sequelae (Table 14.8). Smaller cannulas are placed in children; however, they may still obstruct venous drainage into the heart or impede arterial outflow from the CPB circuit before institution of bypass or after its discontinuation. Almost all cardiac repairs in children necessitate the use of dual venous cannulas so that all venous blood can be diverted to the bypass circuit and the heart can be opened to allow repair of the intracardiac defect.
Table 14.8 Differences in adult versus pediatric cardiopulmonary bypass
1. Profound hypothermia and total circulatory arrest. An alternative method used in very small children with complex heart disease is profound hypothermia and total circulatory arrest. This technique uses a single venous drainage cannula during the period of cooling. When a core temperature of about 18 to 20°C is reached, the pump is stopped and the venous cannula removed. The major advantage of this technique is that it provides excellent exposure without cannulas or blood in the operative field and is especially of benefit in the small neonate. Deep hypothermia also enhances myocardial protection, decreases CPB time, and decreases blood trauma. The Boston Circulatory Arrest Study showed that the use of circulatory arrest is associated with a higher risk of seizures. There was a strong correlation between duration of circulatory arrest and the occurrence of seizures. Seizures in the perioperative period significantly increased the risk of both lower IQ scores and neurologic abnormalities [16]. On the basis of this study, most centers have minimized the use of DHCA; when it must be used, every effort is made to limit its duration to less than 35 to 40 min. As centers have become more advanced in their perfusion and protection techniques, many have begun to employ a strategy of low-flow cerebral perfusion, directed toward the innominate artery. This technique provides cerebral flow and protection while concurrently ceasing all pump flow to the remainder of the body.
2. Venous drainage. Venous drainage problems are more common in children. The inferior vena cava is quite short, and inadvertent cannulation of the hepatic veins is possible, resulting in marked engorgement of the splanchnic vessels and subsequent mesenteric ischemia. Problems with superior vena cava drainage are possible, especially if a left superior vena cava is present. Occlusion of this vessel may cause significant cerebral venous hypertension, and cerebral ischemia may result. Careful attention should be paid to superior vena cava pressure by frequent inspection of the head and monitoring of the CVP via an SVC catheter.
3. Systemic-to-PA–shunt occlusion. When CPB is first initiated, the surgeon must quickly occlude any systemic-to-PA shunts (e.g., PDA or BT shunt). These shunts are often constructed as palliative first-stage interventions for creation of a source of pulmonary blood flow. Lack of occlusion of these shunts with initiation of CPB can lead to underperfusion of the systemic circulation, possible hemorrhagic edema of the lungs, and continued pulmonary venous return with possible overdistention of the left side of the heart.
4. Perfusion flow and pressure. CPB flow rates are proportionately higher in infants and children than in adults, ranging from 80 to 150 mL/(kg min). Adult rates usually range from about 1.8 to 2.2 L/(min m2) or about 50 mL/(kg min). Perfusion pressures tend to be lower in children (30 to 50 mm Hg) when adequate oxygenation and perfusion are apparent. The optimal pressure or flow is unclear, and significant interinstitutional variation exists. The use of NIRS has greatly enhanced the intraoperative monitoring and adjustments of perfusion flows and pressures.
5. Moderate hypothermia and ventricular fibrillation. Moderate hypothermia combined with ventricular fibrillation is occasionally employed in pediatric cardiac repair. With this technique, the patient is cooled to about 28 to 30°C, and the heart is fibrillated but continues to be perfused because an aortic cross-clamp is not placed. The surgeon can open the cardiac chambers without risking entrainment of air into the left side of the heart and subsequent ejection into the arterial circulation.
Deliberate fibrillation is often used during work on the right side of the heart or for relatively simple repairs such as ASD closure. The advantages of deliberate fibrillation with moderate hypothermia include a favorable myocardial supply/demand ratio, decreased risk of air embolus to the brain, and avoidance of aortic cross-clamping and cardioplegia. However, surgical exposure is limited because of intracardiac blood and continued motion of the heart. Therefore, aortic cross-clamping and cardioplegic protection of the heart are necessary for more complex intracardiac repairs, especially in small children.
6. Bypass circuit volume. The bypass circuit volume is large relative to the blood volume in infants. In pediatric CPB circuits, the priming volume may be as much as 700 mL, whereas the estimated blood volume of a 3 kg neonate is 250 to 300 mL. Accordingly, the Hct is reduced by approximately 70%. In contrast, an adult CPB circuit is primed with 1,500 mL for a patient with an estimated blood volume of 5 L; a drop in Hct of less than 25% results. Small infants undergoing complex repairs often require transfusion of red blood cells, platelets, and fresh frozen plasma to offset the dilutional reduction of Hct and clotting factors. More recently, technologic improvements have allowed miniaturization of the circuit with priming volumes as low as 300 to 350 mL. The prime in these instances often consists of reconstituted whole blood and allows for the employment of a blood cardioplegia system. Improvements in air filters have allowed for even miniaturized circuits to maintain a high level of safety despite the changes in the arteriovenous loop diameters. However, there may be significant tradeoffs in using these miniaturized systems. They often entail use of a closed system and may lack some of the usual safety features many practitioners consider standard like an air filter and blood cardioplegia system.
B. When should blood be added to the bypass circuit?
The optimal Hct during CPB is unknown. In general, moderate hemodilution during bypass is well tolerated, because microcirculatory perfusion is improved and metabolic needs are reduced by hypothermia. However, if the Hct is lowered too far, oxygen-carrying capacity is diminished and anaerobic metabolism results. Variation exists among surgical centers, but for most complex repairs, an Hct of 25% to 30% during CPB is used.
The CPB circuit must be primed. Each circuit has an obligatory volume that is required to fill the tubing, filters, pumps, and oxygenator. Therefore, it may be necessary to add red blood cells to the priming solution to reach the desired Hct. Calculation of the Hct on bypass is as follows.
1. Determine the patient’s estimated blood volume.
2. Multiply the estimated blood volume by the measured Hct to yield the patient’s red blood cell mass (RBCM).
3. Ask the perfusionist what the circuit priming volume is.
4. Add the estimated blood volume to the priming volume to obtain the total volume on bypass (CPBV).
5. Predicted Hct on bypass = RBCM/CPBV.
6. If the predicted Hct on bypass is less than desired, the quantity of red blood cells that must be added is calculated as follows:
CPBV × Desired hematocrit = Required RBCM
Required RBCM – Patient’s RBCM = RBCM to be added
C. How is anticoagulation managed?
Heparin is given to prevent initiation of the coagulation cascade by contact of blood with the bypass circuit. A dose of 300 units/kg is generally sufficient, although 400 units/kg is sometimes recommended for neonates. This dose is given through a central catheter after aspiration to verify blood return.
1. Activated clotting time. Measuring activated clotting time about 3 to 5 min after heparin administration can allow documentation of its effect. A value of about 400 s appears adequate to prevent clotting on bypass. Activated clotting time is relatively simple to determine and is reasonable to monitor, because occasionally a patient does exhibit marked heparin resistance. The activated clotting time also can signal a potentially catastrophic drug administration error when a substance other than heparin is injected. If the patient remains normothermic, the activated clotting time is generally rechecked every 20 to 30 min. With significant hypothermia, heparin effect is prolonged. Many centers advocate measuring actual heparin concentrations, especially during hypothermic CPB.
D. Should antifibrinolytics be used?
In an effort to minimize transfusion requirements, many groups have focused on preservation of platelet function and prevention of fibrinolysis, using drugs such as ε-aminocaproic acid (amicar®), tranexamic acid, and aprotinin. Although the data supporting the decreased transfusion requirement in redo operations in adults are fairly convincing, the data are not as clear as in infants. Some groups have reported dramatic decreases in blood loss, whereas others have shown no difference in donor exposures to banked blood [17,18]. The use of aprotinin in the pediatric population has been avoided since October of 2007 due to concerns raised by the Food and Drug Administration regarding its association with increased mortality in the cardiac surgical population. As such, most centers employ the use of amicar or tranexamic acid exclusively.
E. TEG and TEM
CPB induces derangements in the coagulation cascade and this often leads to challenges with immediate postoperative bleeding and the need for significant transfusion of blood and clotting products [19]. This is especially true and of significant challenge in the neonatal cyanotic patient undergoing surgical intervention [20]. Concerns regarding transfusions have historically been well documented and, in particular, can be of challenge in the congenital heart surgical patient population. Proactive detection of hemostatic abnormalities increases the ability to adequately plan treatment strategies and, as a result, minimizes the overall need for transfusion therapies. Due to issues of heparinization and dilution, routine coagulation assays may indeed not be appropriate for adequate assessment during operative intervention [21].
The use of TEM and TEG has gained favor as a tool for measuring coagulation profiles and parameters in the intra- and immediate postoperative management for congenital heart surgery. Both methods measure the viscoelastic properties of the fibrin clot but with somewhat different technology and terminology. The TEG analyzer examines whole blood as the clot forms, to measure the time it takes to reach certain levels of clot formation. It also measures the strength and contribution of key elements in the hemostasis system [21]. It is important to note that levels are not measured; rather, the functional contribution of these various components is assessed.
Several groups have described a reduction in the overall transfusion prevalence in pediatric cardiac surgical patients with use of TEM/TEG [22]. More specifically, red blood cell and plasma transfusion rates have decreased when intraoperative decisions have been guided by TEM or TEG data [21]. These findings are in concert with already well-described similar findings in the adult population.
F. How are patients weaned?
Success in weaning from bypass is critically dependent on the surgeon’s ability to completely repair the defect. Residual shunts, obstruction, or valvular dysfunction are tolerated poorly after bypass. In addition, close communication between surgeon, anesthesiologist, and perfusionist is essential for a planned approach toward separation from the CPB circuit.
1. Preparation. Be certain that the patient is optimally prepared before attempting discontinuation of bypass (Table 14.9). Near the end of the surgical repair, gradual rewarming is begun. Temperature gradients are common; be sure that the patient is thoroughly and evenly rewarmed. The speed and method of rewarming may be critical. Postischemia hyperthermia is particularly deleterious in the setting of altered cerebral energy metabolism. In addition, it is known to be associated with an induction of tachyarrhythmias. On the other hand, mild degrees of hypothermia have been shown to be protective. Infusion of afterload-reducing agents, such as sodium nitroprusside, given during rewarming may be helpful to dilate the vascular bed and promote uniform rewarming. Allowing time for reperfusion of the heart after the cross-clamp is removed makes possible dissipation of “evil humors” (cardioplegia) and re-establishment of aerobic metabolism.
Table 14.9 Checklist for discontinuation of bypass
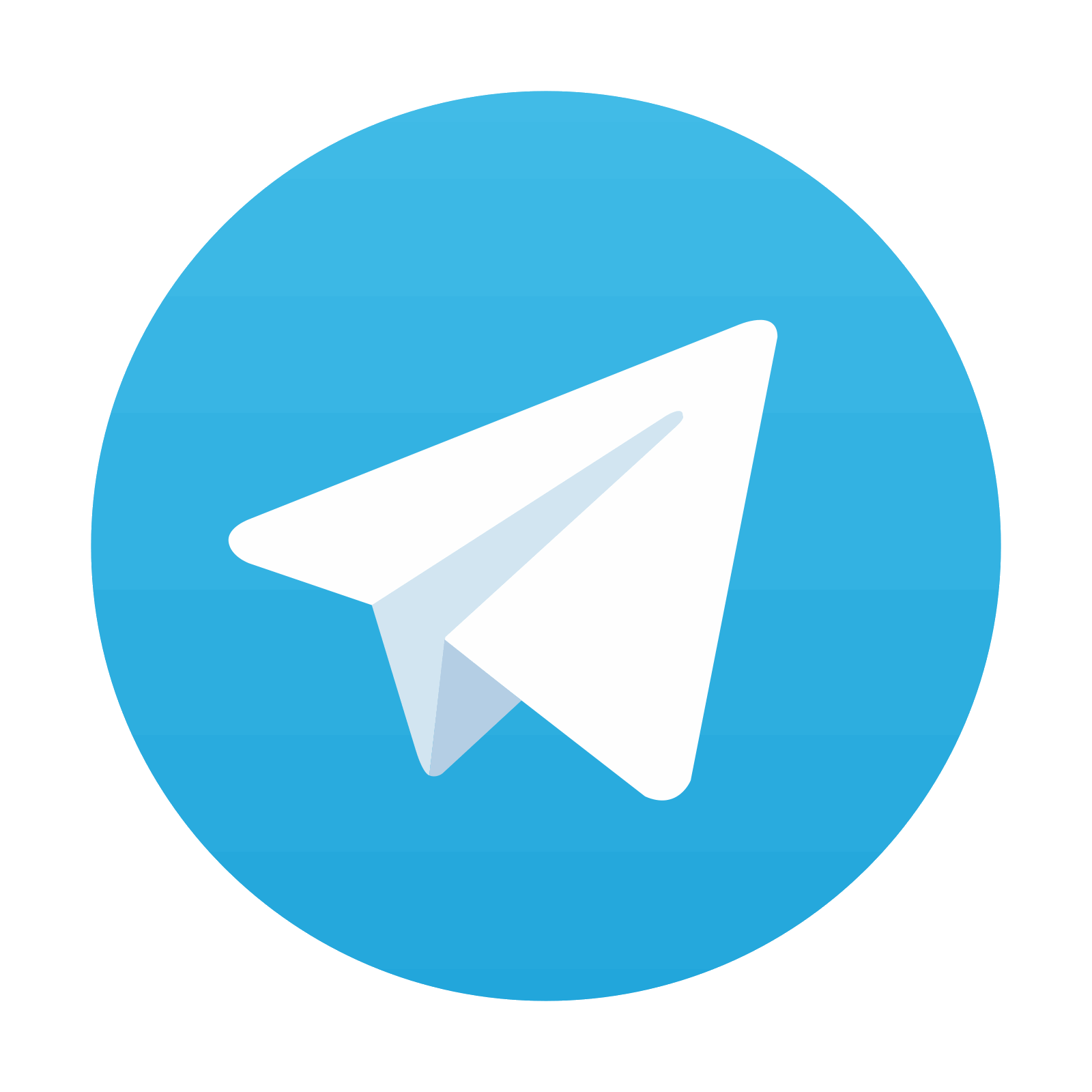
Stay updated, free articles. Join our Telegram channel
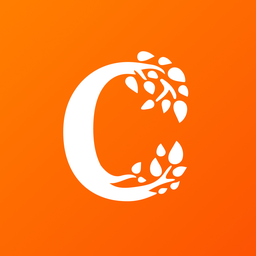
Full access? Get Clinical Tree
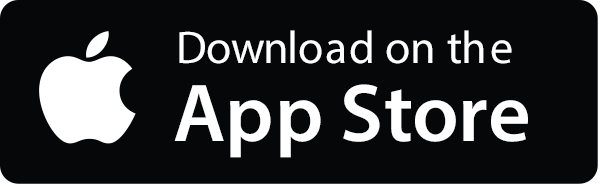
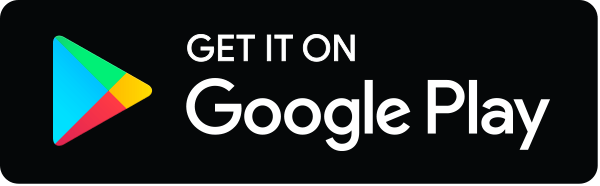