Chapter 121 Anesthesia Principles and Operating Room Anesthesia Regimens
Intraoperative anesthetic can be provided by one of five techniques, which include local anesthesia (sometimes referred to as “local only,” monitored anesthesia care (MAC), peripheral nerve blockade, neuraxial techniques (spinal or epidural anesthesia), and general anesthesia. Local anesthesia involves the infiltration of a surgical site with a local anesthetic agent to render the site insensitive to pain. This may be done solely by the surgeon without the involvement of an anesthesia provider. MAC involves monitoring a patient with standard monitors in accord with guidelines of the American Society of Anesthesiologists (ASA monitors, see below) and administering a sedative and/or analgesic agent intravenously to provide anxiolysis, sedation, and analgesia. MAC is frequently combined with either infiltration of the surgical site with a local anesthetic agent or a regional anesthetic technique. MAC is provided using a combination of a drug with amnestic properties (midazolam or propofol) combined with a drug to provide analgesia (an opioid such as fentanyl). During MAC, spontaneous ventilation is maintained during the procedure, thereby eliminating the need for endotracheal intubation and controlled ventilation. The depth of sedation may range from a state in which the patient is awake and relaxed with the ability to respond to verbal stimuli to a state of deep sedation where a painful stimulus is required to elicit a response. Peripheral nerve blockade and neuraxial anesthesia are frequently considered together under the title of regional anesthesia. A peripheral nerve block involves the placement of a local anesthetic agent around a nerve or group of nerves (plexus) to render specific dermatomes insensitive to pain. Examples of plexus blockade include cervical plexus blockade for superficial and deep neck surgery, brachial plexus blockade for upper extremity or shoulder procedures, or lumbar plexus blockade for hip or leg surgery.1–3 Intravenous regional anesthesia, the Bier block, is another example of a peripheral nerve block that can be used to provide surgical anesthesia. A Bier block involves the intravenous injection of a dilute local anesthetic into a vein of an extremity after that extremity has been exsanguinated by wrapping it with a bandage and then occlusion with a tourniquet. Although the latter technique is generally successful and easy to accomplish, it may not be feasible in younger children except when combined with deep sedation. Given the use of an occlusive tourniquet, its duration is limited to 60 to 70 minutes due to tourniquet pain. The major concern with the Bier block is that the dose of local anesthetic used approaches the toxic limits and should the tourniquet fail, cardiovascular or central nervous system (CNS) consequences from the local anesthetic agent may occur. Neuraxial anesthesia involves the injection of a local anesthetic agent into either the subarachnoid or epidural space. This reasults in blockade of the spinal cord and its accompanying nerve roots to render an entire region of the body (lower abdomen, pelvis, perineum, or lower extremities) insensitive to pain. Examples of neuraxial anesthesia include spinal, epidural, and caudal anesthesia.4,5 In infants and children, a regional anesthetic technique such as a peripheral nerve block or epidural anesthesia can be used instead of general anesthesia in patients with comorbid diseases that significantly increase the risk of anesthesia. More frequently, the regional anesthetic technique is combined with a general anesthetic as part of a balanced anesthetic technique and continued into the postoperative period by use of a continuous infusion via the catheter to provide postoperative analgesia.
When a general anesthesia is used, it should include the four requisites of amnesia, analgesia, muscle relaxation, and attenuation of the sympathetic nervous system’s response to surgical trauma. The phases of general anesthesia include induction, maintenance, and emergence. The induction of anesthesia can be carried out with the intravenous administration of an anesthetic agent (thiopental, propofol, ketamine, or etomidate) or via the inhalation route with an inhalational anesthetic agent such as sevoflurane. Advantages of an intravenous induction include the rapid onset of anesthesia and avoidance of issues associated with inhalation induction including claustrophobia from anesthesia mask placement and the odor of the inhalational anesthetic agent. In pediatric patients, the inhalation induction of anesthesia is frequently chosen to avoid the need for obtaining intravenous access on an awake child. However, when inhalation induction is carried out without intravneous access, airway and cardiovascular issues may arise which may mandate immediate treatment without intravenous access. In such cases, if intravenous access cannot be rapidly obtained, it may be feasible to use the intramuscular (IM) route for a select number of medications (atropine, succinylcholine). However, more aggressive resuscitation such as the administration of epinephrine for hemodynamic compromise may require the use of intraosseous access (IO).6 Fortunately, the majority of problems during inhalation induction can be easily reversed with appropriate airway techniques or the administration of IM medications. Hemodynamic compromise including cardiac arrest was more common with the use of halothane, given its negative inotropic and chronotropic properties.7 However, halothane is no longer used in the practice of anesthesia, having been replaced by sevoflurane. Even if intravenous access is present, the inhalational induction of anesthesia may be chosen as it allows the maintenance of spontaneous ventilation even during deep planes of anesthesia (deep enough to allow for direct laryngoscopy and endotracheal intubation). Such a technique may be used if there is a question regarding the ability to bag-valve-mask ventilate the patient, such as for patients with compromised airways from infection, tumor, or anatomic abnormalities. Following anesthetic induction, one progresses into the maintenance phase of general anesthesia. This can be achieved by the administration of intravenous agents, inhalational agents, or, most often, a combination of the two (balanced anesthesia). An example of a balanced technique includes some combination of inhalational (nitrous oxide and an inhalational anesthetic agent), a continuous infusion of an intravenous anesthetic (propofol), a nondepolarizing neuromuscular blocking agent (NMBA), and an opioid. In most circumstances, the choice of maintenance anesthesia is based on the presence of comorbid features and the preferences of the anesthesiologists.
Preoperative Evaluation
The preoperative evaluation includes a history of present illness, past medical problems including drug allergies, a past surgical and anesthetic history, and a review of the patient’s current and perhaps past medical record and medication list. For elective surgical procedures, the status of comorbid conditions should be optimized prior to the surgical procedure. The latter may not be feasible for urgent or emergent cases. The physical examination is directed primarily at the central nervous system, cardiovascular system, and respiratory system, including an examination of the airway. The preoperative evaluation can identify many of the patients with a difficult airway which may preclude successful endotracheal intubation using standard techniques of direct laryngoscopy. An airway history should be obtained seeking medical, surgical, and anesthetic factors that may indicate a difficult airway. Examination of previous anesthesia records is helpful, although a patient’s airway may change with growth or the development of comorbid conditions. A physical examination of the airway is performed to detect physical characteristics associated with a difficult airway such as a large tongue, small mouth, short neck (shortened thyromental distance), recessed mandible, limited extension or flexion of the neck, limited mouth opening, and difficulty visualizing the uvula and tonsillar pillars when the patient opens their mouth. Risk of airway access is assessed using the Mallampati grading system. Visualization of the entire uvula and tonsillar pillars (Mallampati grade I) suggests that endotracheal intubation will be uncomplicated while failure to visual the tonsillar pillars and the soft palate (Mallampati grade IV) suggests that endotracheal intubation will be difficult. Based on the preoperative evaluation, an ASA Physical Status classification is assigned to the patient based on his or her comorbid features and associated medical conditions (Table 121-1).8 The physical classification is based on the physical condition of the patient and does not include the planned surgical procedure. Laboratory tests and additional investigations are ordered based on the positive findings obtained during the history and physical examination and on the complexity of the surgical procedure.9 The routine preoperative testing of all patients for elective surgery has been shown to be unjustified and expensive. In the absence of comorbid conditions, for surgical procedures with limited chance of significant blood loss, no laboratory or radiologic evaluation is necessary. Although commonly performed, routine testing of coagulation function has been shown to be of limited value without an antecedent history of bleeding problems.10 The most common coagulation disorder that may cause problems intraoperatively is von Willebrand disease; however, it cannot be identified on routine coagulation screening, which is limited to a prothrombin time (PT), partial thromboplastin time (PTT), and an international normalized ratio (INR). Another area of ongoing controversy is the need for routine preoperative pregnancy testing in postmenarchal patients. Given the theoretical potential for anesthetic agents to be teratogenic and the risks of spontaneous abortion, the history should include specific questioning about the potential for pregnancy including the patient’s last menstrual cycle. In addition, there is increasing use of a point-of-care urine pregnancy testing in many centers. Further testing such as pulmonary function tests, electrocardiography, and echocardiography are based solely on the presence of comorbid conditions following the preoperative visit, including the history and physical examination. The planned management of anesthesia is discussed with each patient, and risks and possible complications are reviewed. Options and plans for postoperative pain management are discussed. The history and informed consent complete the preoperative evaluation.
Table 121–1 ASA Physical Status Classification
Classification | Description | Example |
---|---|---|
1 | Normal healthy patient | — |
2 | Mild systemic disease with no functional limitation | Mild asthma, acyanotic congenital heart disease (atrial septal defect) |
3 | Severe systemic disease with functional limitation | Sickle cell disease, cystic fibrosis, palliated cyanotic congenital heart disease |
4 | Severe systemic disease that is a constant threat to life | Advanced stages of muscular dystrophy, cyanotic congenital heart disease with pulmonary hypertension |
5 | Moribund patient not expected to survive without operation | Perforated bowel with sepsis and shock |
6 | Brain-dead patient; organs are being removed for donor purposes | — |
E | Emergency operation | — |
NPO Guidelines
Although the aspiration of gastric contents is an uncommon event, the consequences may be severe, including pneumonitis, respiratory failure, or even death. Classical teaching states that the severity of the aspiration injury relates to the volume aspirated as well as its pH, with severe complications occurring with the aspiration of a volume 0.4 mL/kg or greater or a pH of 2.5 or less. Although aspiration may occur in any setting, patients at risk include parturients, obese patients, diabetics, patients who have received opioids, patients with gastrointestinal disease (reflux, obstruction), patients with altered mental status, patients with intraabdominal pathology (acute abdominal emergencies including appendicitis) and patients in whom difficult airway management is anticipated. These factors may predispose to aspiration by limiting the patient’s ability to protect their own airway, decreasing the normal barrier to aspiration (lower esophageal sphincter tone), increasing gastric volume, or delaying gastric emptying.11,12 Patients who have the highest incidence of perioperative aspiration are those with a high ASA physical status classification (III, IV, or V) and those having emergency surgery. The majority of aspirations occur during the induction of anesthesia or following tracheal extubation when the patient has lost his or her protective airway reflexes.
Classically, keeping patients nil per os (NPO) has been the mainstay of therapy to prevent acid aspiration. In the past, patients were fasted for 8 to 12 hours before surgery to reduce the volume of gastric contents at the time of induction of anesthesia and to decrease the risk of aspiration pneumonitis. This preoperative fast does not take into account differences in gastric emptying of clear liquids and solids. More recently, based on several investigations, there has been a significant revision in the perioperative fasting rules, especially for infants and children. It has been demonstrated that clear liquids have a gastric emptying time of 1 to 2 hours, while solids have an unpredictable gastric emptying time that may be greater than 6 hours.13–16 The ingestion of clear liquids up to 2 hours before surgery does not increase gastric fluid volume or acidity.13–16 As a result, the liberalization of guidelines for ingestion of clear liquids for elective surgery of otherwise healthy patients has been recommended.17,18 A survey of anesthesiologists in the United States has shown that 69% have either changed their NPO policy or are flexible in their practice in allowing clear liquids before elective operations in children.19 Suggested guidelines as recommended by the American Society of Anesthesiologists for patients with no known risk factors include no solid food for at least 6 hours before surgery and unrestricted clear liquids until 2 hours before surgery. Oral medications may be given 1 to 2 hours before surgery with a small sip of water.
Preoperative Medication
There are several categories and uses of preoperative medications (Table 121-2). The most common use of a preoperative medication is to provide sedation and anxiolysis prior to transport to the operating room. Preparing the patient for surgery includes psychological preparation and, frequently, pharmacological premedication. Psychological preparation includes the preoperative visit and an interview by the anesthesiologist. Pharmacologic premedication may be given orally or, rarely, intramuscularly 1 to 2 hours before the induction of anesthesia or intravenously in the immediate preoperative period. Popular choices include benzodiazepines such as midazolam or occasionally, α2-adrenergic agonists such as clonidine or dexmedetomidine. A frequently used agent and route of administration is the oral administration of the benzodiazepine midazolam to ease separation from parents and improve mask acceptance for inhalation induction. This is generally necessary when children are at least 9 to 18 months of age and begin to manifest stranger anxiety. Given alterations in bioavailability when administered by the oral route, doses of 0.3 to 0.5 mg/kg are required.
Table 121–2 Types and Uses of Premedications
Type of Medication | Purpose |
---|---|
Benzodiazepine | Sedation, anxiolysis, amnesia—eases parental separation |
α2-Adrenergic agonists (clonidine, dexmedetomidine) | Sedation, anxiolysis—decrease intraoperative anesthetic needs |
Opioids | Analgesia during invasive procedures |
Anticholinergic agent (atropine, glycopyrrolate) | To prevent bradycardia, blunt airway reflexes, dry secretions |
Inhaled β-adrenergic agonists (albuterol) and anticholinergic agents (ipratroprium) | Prevention or relief of bronchospasm |
Inhaled lidocaine | Prevent airway reflexes during awake fiberoptic intubation, direct laryngoscopy or bronchoscopy |
H2-antagonists, proton pump inhibitors | Decrease pH of stomach contents |
Promotility agents such as metoclopramide | Decrease volume of gastric secretions |
Antiemetic agents (scopolamine patch, neurokinin-1 inhibitors) | Prevention of perioperative nausea and vomiting |
Additional preoperative medications may be used in patients with certain comorbid features, such as the use of H2-antagonists, proton pump inhibitors, or motility agents to increase gastric pH and decrease gastric volume in patients at risk for acid aspiration; inhaled β-adrenergic agonists (albuterol) or anticholinergic agents (ipratropium) may be administered to patients with reactive airway diseases (asthma, recent upper respiratory infection, or chronic obstructive pulmonary diseases). Anticholinergic agents may be used to dry airway secretions in patients requiring fiberoptic intubation.
Monitoring
The standards for intraoperative anesthetic monitoring have been outlined by the American Society of Anesthesiologists. Monitoring standards are the same regardless of whether the case entails a general anesthetic, regional anesthetic (peripheral nerve block, spinal or epidural), or monitored anesthesia care. The standards according to the ASA include an oxygen analyzer, noninvasive blood pressure cuff, continuous ECG, pulse oximeter, end-tidal carbon dioxide analyzer, precordial or esophageal stethoscope, temperature probe, and a ventilator disconnect alarm. Based on the medical condition of the patient and the surgical procedure, more elaborate and invasive monitoring may be added to these standard monitors, such as a urinary catheter; catheters for measuring intraarterial, central venous, and pulmonary artery pressures; and transesophageal echocardiography. Although there are no strict guidelines dictating which patients should have invasive monitors placed, there have been recommendations set forth for the adult population. These recommendations must be taken in context of the limited data available comparing outcomes, for instance, in patients managed perioperatively with or without pulmonary artery (PA) catheters.20,21 The ASA recommends considering three variables including disease severity, magnitude of the surgical procedure, and practice setting when assessing benefit versus risk of PA catheters.22 Additional information regarding structural and functional issues of the myocardium may be obtained by the use of transesophageal echocardiography (TEE). TEE is used with increasing frequency in the adult population. There are now specific curriculae in cardiac anesthesia fellowships to teach the skills necessary for performance of TEE. The practice has been encouraged by the American Board of Anesthesiology, which recognizes such training and provides the opportunity for credentialing through the completion of a written examination. The strongest indications for perioperative transesophageal echocardiography that are supported by evidence-based medicine include cardiac surgery procedures such as repair of valvular lesions (insufficiency or stenosis) or congenital lesions, assessments and repairs of thoracic aortic aneurysms and dissections, pericardial window procedures, and the repair of hypertrophic obstructive cardiomyopathy.23 For noncardiac surgery, intraoperative transesophageal echocardiography is indicated to evaluate acute, persistent, and life-threatening hemodynamic disturbances in which ventricular function and its determinants are uncertain and which have not responded to treatment, especially when placement of a PA catheter is not feasible.
In addition to standard ASA monitors, there is growing interest in the development and potential use of “depth of anesthesia” monitors. Although controversial, the potential impact of such monitors is highlighted by the results of several trials which demonstrate that intraoperative awareness may occur in anywhere from 0.1% to 0.2% of all patients, with even higher incidences in specific procedures including trauma, cardiac, obstetrical, and emergency surgery. Several manufacturers have marketed or are developing monitors which provide the anesthesia provider with a numerical value against which anesthetic agents are titrated. There are currently five such monitors, including the Bispectral Index (BIS monitor, Aspect Medical, Newton, MA); the Narcotrend (MonitorTechnik, Bad Bramstedt, Germany), which is currently available only in Europe; Patient State Analyzer (PSA 4000, Baxter Healthcare, Deerfield, IL); SNAP (Everest Medical, Minneapolis, MN); and Auditory Evoked Potential Monitor (AEP Monitor, Danmetter Medical). To date, the one that has received the most clinical use is the BIS monitor. The BIS is a modified electroencephalographic monitor that uses a preset algorithm based on intraoperative data obtained from adults to evaluate the electroencephalogram. The BIS number is determined from three primary factors, including the frequency of the electroencephalographic waves, the synchronization of low and high frequency information, and the percentage of time in burst suppression. Part of the simplicity and attraction of the BIS monitor is that the depth of sedation/anesthesia is displayed numerically, ranging from 0 to 100, with 40 to 60 being a suitable level of anesthesia to ensure amnesia and lack of recall. With the use of BIS monitoring, a decreased incidence of awareness has been demonstrated, as well as a decrease in the total amount of anesthetic agent used.24–26 Additional studies have suggested faster recovery times and faster discharge times from the postanesthesia care unit; all of which may translate into reduced perioperative costs.26,27 Although not considered the standard of care as of yet for intraoperative anesthesia care, the ASA does recommend the availability of such monitors whenever general anesthesia is provided. Given the success of such monitors in the perioperative arena, there is ongoing interest in the application of such technology in the ICU and the procedural sedation arena.28–30
The Pharmacology of Anesthetic Agents
Local Anesthetic Agents
The mechanism of action for the majority of local anesthetic agents involves blockade of sodium channels in the nerve membrane, thereby preventing depolarization. The non-ionized portion of the local anesthetic agent penetrates the lipid membrane, while the ionized portion reversibly blocks the inner aspect of the sodium channel. Local anesthetic agents differ in intrinsic potency, onset of action, duration of action, and their ability to produce differential sensory and motor blockade. Potency is determined primarily by lipid solubility (high lipid solubility is directly related to potency).31 Bupivacaine and tetracaine are examples of local anesthetic agents with high lipid solubility and hence high potency. The onset of action is determined primarily by the pKa with onset being most rapid in those agents with a pKa closest to physiologic pH.32,33 With a pKa close to physiologic pH, the percentage of the un-ionized form is greater, thereby increasing passage through the nerve membrane. Lidocaine has a pKa of 7.7 and at a pH of 7.4, 35% exists in the un-ionized base form yielding a relatively rapid onset of blockade. In contrast, tetracaine has a pKa of 8.6 with only 5% in the un-ionized form at a tissue pH of 7.4, resulting in a slower onset of blockade than lidocaine. Duration of action is determined primarily by the degree of protein binding to receptors in the sodium channel.34 Local anesthetic agents bind to protein receptors in the sodium channels. High protein binding and therefore a prolonged duration of action are characteristic of bupivacaine, levobupivacaine, tetracaine, and ropivacaine. Duration of action is also influenced by the degree of vasodilation produced by the local anesthetic.35 Vasodilatation results in increased blood flow to the area and therefore an increased removal of the agent from the depot in the tissues. The local anesthetic agents also differ in their differential effects on sensory versus motor nerves. Bupivacaine and ropivacaine demonstrate this property, which is very beneficial for postoperative analgesia administered through an epidural catheter so that patients are able to ambulate with minimal discomfort.
When performing regional anesthesia, the goal is to place the local anesthetic agent as close as possible to the nerve or plexus that needs to be anesthetized. A recent addition to the armamentarium of the anesthesiologist has been the use of ultrasound to visualize the individual nerve roots or the plexus that is to be anesthetized.37–40 This technology is also being used for neuraxial techniques including spinal and epidural anesthesia. The advantages of this technology are not only an increased success rate of various regional anesthetic techniques, but also the ability to provide blockade with a decreased dose of the local anesthetic agent. Additional factors that must be considered when using these agents are the maximal allowable dose of the agent, the impact when a vasoconstrictor such as epinephrine is added to the solution, and the effect of the site of administration. Increasing the dose of a local anesthetic (increased concentration or volume) yields a faster onset of effect, a longer duration of action, and a greater depth of blockade.41 However, higher plasma concentrations of the local anesthetic agent will also be achieved, thereby increasing the risks of toxicity (discussed later).
Given the catastrophic effects of local anesthetic toxicity, mechanisms to avoid it and prevent its occurrence are mandatory during the performance of regional anesthetic techniques in infants and children. Epinephrine (5 μg/mL or a concentration of 1:200,000) is added to the local anesthetic solution during performance of a regional anesthetic technique to cause local vasoconstriction, thereby decreasing the vascular absorption of the drug and also to serve as a marker of inadvertent systemic injection.41–43 However, the ability of epinephrine to prolong the duration of action depends on the local anesthetic used and the site of administration. More importantly, epinephrine is used as a marker for inadvertent systemic injection. Even with negative aspiration for blood, there is the potential for inadvertent intravascular administration, thereby suggesting the use of a test dose. The test dose entails the administration of 3 mL of the 5 μg/mL epinephrine solution or a total epinephrine dose of 15 μg. If this amount of epinephrine is injected intravascularly, it can generally be detected by changes in heart rate, blood pressure, or the ST-T wave segments of the electrocardiogram and will thereby alert the practitioner that inadvertent intravascular injection is occurring.44
The site of injection of the local anesthetic agent also has a significant impact on the clinical effects including duration of action and vascular uptake (plasma concentrations). The shortest durations of action occur with either intrathecal injection for spinal anesthesia or subcutaneous administration. The longest duration of action and onset of blockade are seen with major peripheral nerve blocks (brachial or lumbar plexus blockade).45,46 The vascular absorption of the local anesthetic agent and its plasma concentration are also dependent on the site of administration. The highest plasma concentration occurs following an intercostal nerve block or interpleural analgesia followed in order by caudal epidural, lumbar or thoracic epidural, brachial plexus, peripheral nerve blockade, subarachnoid, and subcutaneous infiltration.47
During performance of regional anesthesia, the greatest risk of morbidity and mortality results from the achievement of toxic plasma concentrations of the drug. Local anesthetic-induced systemic toxicity affects the CNS and the cardiovascular (CV) system. The differential effects on these two organ systems and the plasma concentration at which toxic effects are noted vary according to the agent. With most local anesthetic agents, CNS toxicity occurs at doses and blood levels below those that produce cardiovascular toxicity. The latter provides some degree of safety, as the CNS symptoms (seizures) are generally more amenable to treatment than the cardiovascular effects (arrhythmias and conduction blockade). Death from local anesthetic toxicity is most commonly the result of the cardiovascular effects of these agents with adverse effects on cardiac electrical and mechanical activity.48 Bupivacaine produces cardiac arrhythmias by inhibiting the fast sodium channels and the slow calcium channels in the cardiac membrane. Hypercarbia, acidosis, and hypoxia potentiate the negative chronotropic and inotropic effects of high plasma concentrations of local anesthetic agents. These effects are so profound that resuscitative measures for ventricular tachycardia/fibrillation including standard ACLS protocols may be ineffective. Anecdotal case reports have suggested the potential role of various agents such as amiodarone for refractory ventricular arrhythmias. More recently, anecdotal human data and animal studies have suggested that intralipid solutions may be used to bind the local anesthetic agent, thereby resulting in return of spontaneous circulation. Current recommendations from the ASA include easy and ready access to 20% intralipid solutions whenever large doses of local anesthetic agents are used for regional anesthetic techniques. Given the risks of morbidity and mortality from local anesthetic toxicity, avoidance of toxicity is the goal through careful calculation of the dose, use of the lowest necessary dose (concentration and volume), use of a test dose with epinephrine to identify inadvertent intravascular injection, intermittent aspiration to identify vascular penetration, and slow incremental injection of the dose.
Intravenous Anesthetic Agents
Thiopental, propofol, and etomidate mediate their anesthetic properties through interactions with the γ-aminobutyric acid (GABA)A receptor complex. These interactions lead to enhanced activity of the inhibitory neurotransmitter system (GABA).49–52 Activation of the GABAA receptor increases the transmembrane movement of chloride, resulting in hyperpolarization of the postsynaptic cell membranes. Ketamine’s analgesic and anesthetic effects are the result of its interactions with the N-methyl-D-aspartate (NMDA) system, which is activated by glutamate, an excitatory transmitter, as well as by other sites within the CNS, including those involved with opioid and cholinergic transmission.53–55 The intravenous anesthetic agents result in somewhat varying end-organ effects. The barbiturates, propofol, and etomidate reduce cerebral metabolism (CMRO2), cerebral blood flow (CBF), and intracranial pressure (ICP). As such, they are valuable agents in the practice of neuroanesthesia or in critically ill patients with increased ICP. When compared with propofol or the barbiturates, etomidate may be preferred in patients with abnormal cardiovascular function as it provides greater hemodynamic stability. As a result, etomidate maintains cerebral perfusion pressure (CPP = MAP – ICP), whereas propofol and thiopental may decrease MAP through their effects on systemic vascular resistance (vasodilatation) as well as direct negative inotropic properties. Thiopental, and perhaps etomidate and propofol, may possess “neuroprotective” properties secondary to reducing CMRO2, which improves the ability of the brain to tolerate incomplete ischemia during procedures such as carotid endarterectomy or the temporary occlusion of cerebral arteries during an aneurysm repair.56,57 Ketamine’s direct effects on ICP remain controversial, with the older literature suggesting that ketamine may directly increase cerebral blood flow and ICP. However, recent studies suggest that ketamine has limited effects on CBF and ICP, especially when given in combination with other anesthetic agents including midazolam.72–74
Most intravenous anesthetics have anticonvulsant properties. Various of the barbiturates and propofol have been incorporated into algorithms for the treatment of refractory status epilepticus.61,62 Opposite effects are generally seen with etomidate, which can produce involuntary myoclonic movements from an imbalance of inhibitory and excitatory influences in the thalamocortical tract. Etomidate also stimulates the EEG resulting in increased amplitude and frequency.63 Myoclonic movements and opisthotonic posturing have also been reported following the administration of propofol. These movements are attributed to propofol’s antagonism at glycine receptors in subcortical structures.
The intravenous anesthetic agents also have dose-dependent effects on ventilatory function. Thiopental, propofol, etomidate, and midazolam result in a decrease of tidal volume and minute ventilation as well as a rightward shift of the CO2 response curve. As with many of the end-organ effects of the anesthetic agents, the respiratory depressant effects may be magnified in patients with comorbid conditions (chronic respiratory or cardiovascular disease) and when coadministered with other medications that are respiratory depressants (inhalational anesthetic agents, opioids, phenothiazines). Given these effects on central control of ventilation, a transient period of apnea generally occurs following an anesthetic induction dose of any of these agents. In contrast to the respiratory effects of propofol, etomidate, and the barbiturates, in the absence of comorbid diseases, ketamine can generally be expected to result in minimal respiratory depression in clinically relevant doses and may preserve airway protective reflexes.64,65 Ketamine also stands apart from the other intravenous anesthetic agent in that the release of endogenous catecholamines following its administration results in bronchodilatation, thereby making it a suitable induction agent in patients who are actively wheezing or at risk for reactivity during airway manipulation. Propofol has also been shown to have beneficial airway effects in patients with airway reactivity. In a prospective trial, 77 adult patients were randomized to receive one of three agents: propofol (2.5 mg/kg), etomidate (0.4 mg/kg), or thiopental (5 mg/kg) for anesthetic induction and tracheal intubation.66 Following endotracheal intubation, respiratory resistance was lower with propofol when compared to either etomidate or thiopental. Additional evidence for the beneficial effects of propofol on airway reactivity are provided by Pizoz et al., who randomized asthmatic or nonasthmatic patients to anesthetic induction with thiopental/thiamylal (5 mg/kg), methohexital (1.5 mg/kg), or propofol (2.5 mg).67 In asthmatic patients, the incidence of wheezing was 45% with thiopental/thiamylal, 26% with methohexital, and 0% with propofol. In nonasthmatic patients, the incidence of wheezing was 16% with thiopental/thiamylal and 3% with propofol. The potential beneficial effects of propofol on airway reactivity are further supported by animal studies.68,69 The proposed mechanism for these effects are a decrease of intracellular inositol phosphate, resulting in a depression of intracellular calcium availability.
During anesthetic induction or maintenance, the intravenous anesthetic agents can depress the cardiovascular system resulting in hypotension by various mechanisms. These include a reduction of central and/or peripheral autonomic nervous system activity, blunting compensatory baroreceptor reflexes, decreasing preload, systemic vasodilatation, or directly depressing myocardial contractility. Hemodynamic function during the induction of anesthesia may also be affected by comorbid cardiovascular disease, intravascular volume status, resting sympathetic nervous system tone, concomitant medications (angiotensin-converting enzyme inhibitors, β-adrenergic antagonists), and the administration of other agents used for anesthetic care including opioids and benzodiazepines. An induction dose of thiopental causes a variable decrease in cardiac output, systemic vascular resistance, and arterial pressure. The decrease in cardiac output is the result of vasodilation as well as direct myocardial depression. This effect is generally well tolerated in patients with adequate cardiovascular function, but can be exaggerated with preexisting cardiovascular disease, necessitating the use of a lower dose of thiopental or, preferably, the use of alternative agents in patients with compromised cardiovascular function. Propofol demonstrates cardiovascular depressant effects similar to or greater than those of thiopental. Propofol is a direct myocardial depressant and reduces systemic vascular resistance. Significant cardiovascular responses following propofol are more common with high doses, in hypovolemic patients, in elderly patients, and in patients with significant cardiovascular disease.71,72 The deleterious cardiovascular effects of propofol can be attenuated by the administration of calcium chloride (10 mg/kg).73 Additional cardiovascular effects from propofol may result from its augmentation of central vagal tone leading to bradycardia, conduction disturbances, and asysole.74,75 The negative chronotropic effects of propofol are more common when it is administered with other medications known to alter cardiac chronotropic function (fentanyl or succinylcholine). Although the relative bradycardia may be beneficial in elderly patients at risk for myocardial ischemia, it may be detrimental if cardiac output is heart rate dependent.
In contrast to the negative inotropic effects of propofol and the barbiturates, etomidate causes minimal cardiovascular depression and may be used for anesthetic induction in patients with significant cardiovascular disease.76,77 As etomidate has little effect on systemic vascular resistance, it may be used in patients with cyanotic congenital heart disease in whom pulmonary blood flow is dependent on mean arterial pressure. Although suppression of adrenal cortical function occurs even following a single bolus dose of etomidate through inhibition of the activity of 17-α hydroxylase and 11-β hydroxylase, it remains controversial whether such effects are of clinical significance.78,79 However, the risk: benefit ratio of this effect must be entertained when etomidate is chosen for anesthetic induction. Additionally, although still used as a single induction dose in patients with comorbid cardiovascular disease, repeated doses or continuous infusions are not recommended.80
The cardiovascular effects of ketamine are different from those of the other intravenous anesthetic agents. Ketamine stimulates the cardiovascular system by activation of the sympathetic nervous system and the release of endogenous catecholamines.81 Anesthetic induction doses of ketamine (1 to 2 mg/kg) generally increase heart rate and MAP. Although the indirect effects of ketamine include the release of endogenous catecholamines and stimulation of the sympathetic nervous system, ketamine is a direct myocardial depressant. In most clinical scenarios, the indirect effects compensate for the direct negative inotropic effects. However, in critically ill patients who have depleted their endogenous catecholamines, cardiovascular collapse may occur.
The pharmacokinetic profile of the intravenous anesthetic agents is characterized by a rapid onset of CNS effects secondary to the high lipid solubility of these agents and the high percentage of cardiac output perfusing the brain. The termination of the central CNS effect results from redistribution of the drug from the central to the peripheral compartment. It is not dependent on primary metabolism and elimination of the drug from the body. Most intravenous anesthetics are metabolized in the liver and excreted in the kidney. Some metabolites are active, such as desmethyldiazepam (diazepam) and norketamine (ketamine), and may result in prolonged effects especially with repeated dosing or the use of continuous infusions. There is a wide variation in the elimination half-lives of intravenous anesthetic agents because of differences in clearance. Drugs with short elimination half-lives include propofol, etomidate, ketamine, and midazolam, whereas thiopental has a long elimination half-life. Propofol is widely used, especially in ambulatory surgery centers, because of its short duration of action, fast recovery time, and early discharge potential.82,83 This rapid recovery results in less “hangover” effect or residual drowsiness following outpatient surgical procedures, thereby facilitating return to work and resumption of activities of daily life.
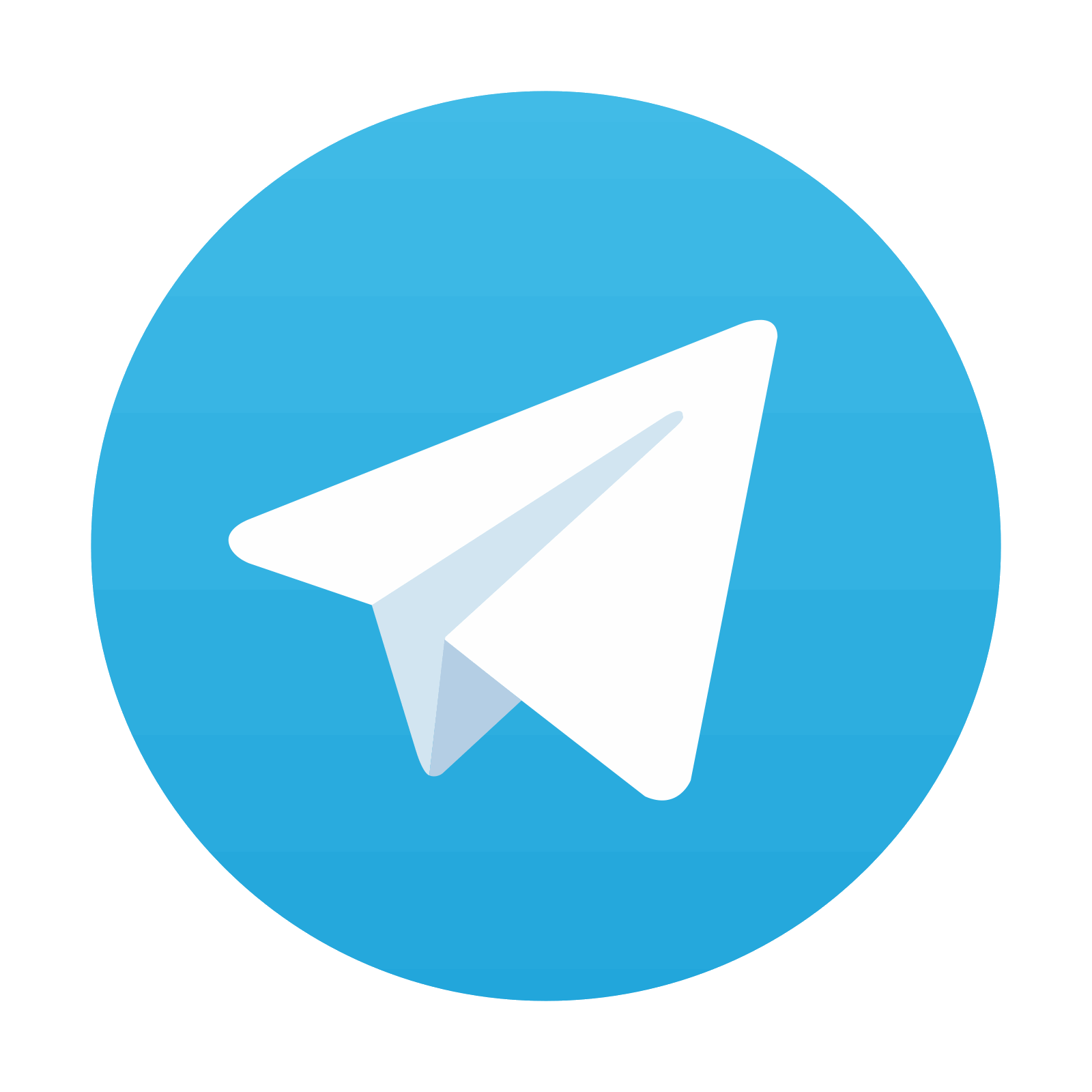
Stay updated, free articles. Join our Telegram channel
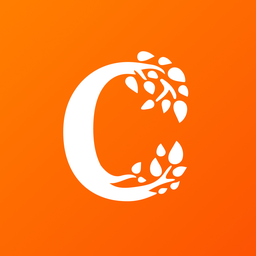
Full access? Get Clinical Tree
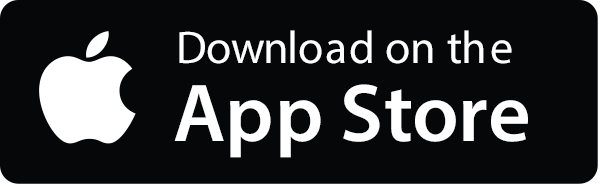
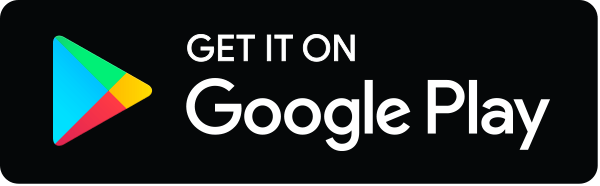