Key Points
- ▪
Perioperative anesthesia care for patients who have undergone acute trauma depends on an understanding of trauma system design and surgical priorities.
- ▪
Successful emergency airway management is based on having a clear plan, such as the American Society of Anesthesiologists algorithm for difficult airways adapted for trauma. In general, rapid sequence induction of anesthesia and in-line cervical stabilization, followed by direct laryngoscopy or video laryngoscopy, is the safest and most effective approach. The use of cricoid pressure is controversial and is no longer a class I recommendation.
- ▪
Recognition of hemorrhagic shock is at the center of advanced trauma life support. Hemorrhagic shock indicates the need for rapid operative treatment, with the possibility of a damage control approach. Although establishing an adequate airway remains the initial priority, obvious hemorrhage should be concurrently addressed through immediate application of tourniquets or direct pressure.
- ▪
Resuscitation during acute hemorrhagic shock has undergone a significant change in emphasis. Current recommendations are to allow permissive hypotension during active bleeding by limitation of crystalloid infusion. Recognizing the impact of early coagulopathy in trauma, a “hemostatic” resuscitation should be employed, with an emphasis on maintenance of blood composition by early transfusion of red blood cells, plasma, and platelets, and viscoelastic monitoring when available.
- ▪
Management of patients with severe traumatic brain injury requires monitoring and maintenance of cerebral perfusion and oxygenation for successful operative and intensive care management.
- ▪
Operative timing for the surgical management of traumatic injuries, including orthopedic trauma, must be balanced between early definitive repairs and the potential for worsening overall physiologic stress.
- ▪
Trauma anesthesiology includes a substantial component of critical care practice (see also Chapter 83 ).
Acknowledgment
The editors, publisher, and Dr. Thomas E. Grissom would like to thank Drs. Maureen McCunn and Richard P. Dutton for their contribution to this chapter in the prior edition of this work. It has served as the foundation for the current chapter.
Introduction
Epidemiology
Death and disability caused by injuries remain a public health threat worldwide. For both children and adults younger than age 45, traumatic injuries remain the leading cause of death in the United States. Moreover, injury-associated deaths have substantial economic consequences; the total estimated lifetime medical and work-lost costs associated with fatal injuries has been reported to be over $240 billion and is projected to increase. Unintentional injuries (i.e., injuries due to motor vehicle accidents, falls) remain the top cause of death in adults younger than age 45, followed by suicide, and homicide.
As with other endemic diseases, successful treatment of trauma extends well beyond the boundaries of an individual hospital. Community-based prevention has included efforts to incorporate airbags in motor vehicles, mandate the use of helmets on motorcycles, encourage citizens to wear seat belts, punish intoxicated drivers, and promote responsible handgun ownership. All these measures have had an impact on the demographics of injury in much the same manner that smoking cessation, dietary modification, and routine mammography have affected the incidence of heart disease and cancer. When prevention fails, outcomes after injury are heavily influenced by the community’s commitment to an organized system of trauma care.
Modern Trauma Systems and Regionalization
Following the landmark National Academy of Sciences report “Accidental Death and Disability: The Neglected Disease of Modern Society” in 1966, a framework for trauma systems was developed by the American College of Surgeons (ACS) Committee on Trauma. Comprised of governmentally designated or internally validated trauma centers, trauma systems represent a coordinated, organized, and patient-centric approach to the care of the injured patient. A distinction between trauma center designation and verification is made to identify the types of resources available and the number of patients treated annually. Trauma center designation is a process outlined and developed at a state or local level. Trauma center verification is an evaluation process done by the ACS to evaluate and improve trauma care; the verification process is voluntary and identifies the presence of resources considered essential for the optimal care of the injured patient. Trauma center levels range from Level I (a comprehensive regional resource providing 24-hour in-house coverage, referral resource for communities in nearby regions, leadership in prevention, research, and more) to Level V (basic emergency department [ED] facilities to implement advanced trauma life support [ATLS], after-hours activation protocols, limited surgery and critical care). Level I and Level II centers represent tertiary care centers; the standards for the provision of clinical care to injured patients for Level I and Level II trauma centers are identical. A trauma system is an example of tiered regionalization because the most seriously injured patients in a geographical catchment area are cared for at designated tertiary care trauma centers. Over the past three decades, many studies have demonstrated significantly improved mortality, morbidity, and cost savings after establishment of regionalized trauma systems.
The Role of the Anesthesiologist
At all levels of trauma care, anesthesiologists are uniquely juxtaposed with the multidisciplinary trauma team, serving both an administrative role in preparing the operating room (OR) and allocating resources for resuscitation, while providing direct patient care through definitive airway management and advanced resuscitation where appropriate. Anesthesiologists also play a significant role as intensivists and pain management experts. Trauma patients represent a significant proportion of all OR cases handled during night and weekend shifts. Regrettably, very few anesthesiologists in the United States consider trauma their primary specialty. This is distinct from European practice, where anesthesiologists frequently are found working in the prehospital environment, as an ED director, or as leader of a trauma team. The United States model, in which all anesthesiologists treat trauma patients—but few do so exclusively—has led to a relative dearth of research, publication, and education in this field. This situation is unfortunate because trauma is a rapidly evolving field of study that presents unique challenges to the clinician and one in which improvements in care can have a dramatic impact on society as a whole.
Anesthesia for trauma patients is different from routine OR practice. Most urgent cases occur during off-hours, when the most experienced OR and anesthesia personnel may not be available. In small hospitals and military and humanitarian practice, austere conditions may influence the resources available. Patient information may be limited, and allergies, genetic abnormalities, and previous surgeries may create sudden crises. Patients are frequently intoxicated, with full stomachs and the potential for cervical spine instability. Simple operations may become complicated, and specialty surgical and anesthesia equipment may be required on short notice. Patients often have multiple injuries requiring complex positioning, multiple procedures, and the need to consider priorities in management. Occult injuries, such as tension pneumothorax, can manifest at unexpected times. Fortunately, there does not appear to be a higher risk for medical liability associated with the provision of anesthesia for trauma versus nontrauma surgical anesthesia cases. Successful perioperative care of these patients requires a good understanding of the basics, supplemented by preparation, flexibility, and the ability to react quickly to changing circumstances.
This chapter provides an overview of important areas of trauma care for the anesthesiologist beginning with a description of the initial approach to an injured patient, followed by discussions of emergency airway management, resuscitation, and care of patients with central nervous system (CNS) injuries. The needs of orthopedic and reconstructive surgery patients are outlined and the chapter concludes with a discussion of postoperative issues for the anesthesiologist managing the trauma patient.
Prioritizing Trauma Care
Prehospital Triage
Prehospital triage of the seriously injured trauma patient begins in the field, and is fraught with difficulty. Estimations of blood loss are imprecise and classically taught shock classifications are commonly confounded by extremes of age and variations in physiological reserve. In 2011, the Centers for Disease Control and Prevention along with the National Highway Traffic Safety Administration collaborated with the ACS Committee on Trauma to revise previous field triage decision schemes in order to reduce over triage of patients with non–life-threatening injuries, and to help direct patients in most need of lifesaving interventions to appropriate trauma centers. Current guidelines recommend a four-step assessment to assist prehospital providers with making decisions about which patients are most in need of transport to a trauma center ( Box 66.1 ).
Physiological Considerations
Systolic blood pressure <90 mm Hg
Glasgow Coma Scale ≤13
Respiratory rate <10 or >29 (or need for ventilatory support)
Anatomical Considerations
Any penetrating injury to the head, neck, torso, and extremities (proximal to the elbow or knee)
Chest wall instability/deformity
Amputation proximal to the wrist or ankle
Pelvic fracture
Open/depressed skull fracture
Paralysis
Mechanisms of Injury
Death of occupant in same vehicle
Fall from >20 feet
Extrication time >20 min
Special Patient or System Considerations
Age >55 years
Children
Patients on anticoagulants or with bleeding disorders
Burns (to be triaged to designated burn centers)
Pregnancy >20 weeks
Traditionally, mechanism of injury has been referred to as blunt versus penetrating trauma, with no further delineation as to how much energy was imparted, or information regarding anatomical and physiological insults. Some studies have suggested that mechanism of injury alone is a poor predictor for trauma center referral. Others have demonstrated that distinct mechanisms, such as ejection from a vehicle or prolonged extrication time, clearly warrant trauma team activation. In a study by Lerner and associates, the ACS Field Triage Decision scheme was examined, and interviews conducted with emergency medical technicians who transported patients to trauma centers based on mechanism alone. Only three mechanisms of injury reliably predicted the need for referral to a trauma center when patients did not meet anatomical or physiological injury criteria: death of an occupant in the vehicle, fall greater than 20 feet, and extrication time greater than 20 minutes. Additional studies have justified mechanism of injury as a parameter that helps reduce inappropriate transport of patients with major trauma to nontrauma centers. For more information on this subject see Chapter 67 .
Blunt Versus Penetrating Trauma
Blunt and penetrating injuries are regularly disparate in presentation but may share similarities in terms of extent of injury. Penetrating injuries are identified as ballistic and nonballistic. The point of injury in the patient with penetrating trauma may be utterly discernible—even to the inexpert provider—but the extent of tissue damage and depth of shock may be less detectible compared to the patient suffering from a blunt traumatic injury. Conversely, the patient with penetrating trauma will lose blood volume externally together with loss into body cavities, whereas the patient with blunt trauma may present in hemorrhagic shock with no obvious signs of hemorrhage. Multiple blunt traumatic insults, bleeding into compartments (e.g., unstable long-bone fractures), retroperitoneal hemorrhage (e.g., pelvic fractures, major vascular injury, solid organ damage), and bleeding into other body cavities may present as indolent hemorrhagic shock.
Advanced Trauma Life Support
The performance of a thorough patient assessment, application of rapid diagnostic tests, and early activation of resources is vital for ensuring optimal outcomes in patients with severe traumatic injuries. The ATLS course of the ACS is the most widely recognized training program for trauma physicians of all disciplines. Although not comprehensive in subspecialty areas, the ATLS curriculum provides a framework and a common language for the care of injured patients. ATLS is based on a “primary survey” that includes simultaneous efforts to identify and treat life- and limb-threatening injuries, beginning with the most immediate. This focus on urgent problems first is captured by the “golden hour” catchphrase and is the most important lesson of ATLS. Resolution of urgent needs is followed by a meticulous secondary survey and further diagnostic studies designed to reduce the incidence of missed injuries. Knowing the basics of ATLS is essential for any physician who interacts with trauma patients. Fig. 66.1 is a simplified representation of the ATLS protocol.

ATLS emphasizes the “ABCDE” mnemonic: airway, breathing, circulation, disability, and exposure. Verification of an open airway and acceptable respiratory mechanics is of primary importance because hypoxia is the most immediate threat to life. Inability to oxygenate the patient will lead to permanent brain injury and death within 5 to 10 minutes. Trauma patients are at risk for airway obstruction and inadequate respiration for the reasons listed in Box 66.2 . Endotracheal intubation, whether performed in the prehospital environment or in the ED, must be confirmed immediately by capnometry. Esophageal intubation or endotracheal tube (ETT) dislodgement are common and devastating if not promptly corrected. Patients in cardiac arrest may have very low end-tidal carbon dioxide (CO 2 ) values; direct laryngoscopy should be performed if there is any question about the location of the ETT (see also Chapter 44 ).
Airway Obstruction
Direct injury to the face, mandible, or neck
Hemorrhage in the nasopharynx, sinuses, mouth, or upper airway
Diminished consciousness secondary to traumatic brain injury, intoxication, or analgesic medications
Aspiration of gastric contents, blood, or a foreign body (i.e., dentures, broken teeth, soft tissue)
Misapplication of oral airway or endotracheal tube (esophageal intubation)
Inadequate Ventilation
Diminished respiratory drive secondary to traumatic brain or high cervical spine injury, shock, intoxication, hypothermia, or oversedation
Direct injury to the trachea or bronchi
Pneumothorax or hemothorax
Chest wall injury
Aspiration
Pulmonary contusion
Cervical spine injury
Bronchospasm secondary to smoke or toxic gas inhalation
If establishment of a secure airway and adequate ventilation requires a surgical procedure such as a tracheostomy, tube thoracostomy, or open thoracotomy, this procedure must precede all others. Indeed, these procedures are commonly performed in the ED, often before the arrival of an anesthesiologist. Subsequent surgery to convert a cricothyroidotomy to a tracheostomy or close an emergency thoracotomy may then follow in the OR.
Hemorrhage is the next most pressing concern since ongoing blood loss is inevitably fatal. The symptoms of shock are presented in Box 66.3 . Shock is presumed to result from hemorrhage until proven otherwise. Assessment of the circulation consists of an early phase, during active hemorrhage, and a late phase, which begins when hemostasis is achieved and continues until normal physiology is restored. In the early phase, diagnostic efforts focus on the five sites of bleeding detailed in Table 66.1 , the only areas in which exsanguinating hemorrhage can occur. Immediate actions to control hemorrhage can include application of pelvic binders for bleeding associated with pelvic fractures or tourniquet application for extremity injuries. Any surgical procedure to diagnose or control active hemorrhage is an emergency case that must be brought to the OR as soon as possible. This includes exploration of the neck or pericardium to rule out hemorrhage in sensitive compartments. In the OR, the trauma surgeon focuses on anatomic control of hemorrhage, whereas the anesthesiologist is responsible for restoring the patient’s physiology. Goals for early and late resuscitation are discussed in more detail later.
Pallor
Diaphoresis
Agitation or obtundation
Hypotension
Tachycardia
Prolonged capillary refill
Diminished urine output
Narrowed pulse pressure
Site of Bleeding | Diagnostic Modalities | Treatment Options |
---|---|---|
Chest | Chest x-ray | Observation |
Thoracostomy tube output | Surgery | |
Chest CT | ||
Abdomen | Physical examination | Surgical ligation |
Ultrasound (FAST) | Angiography | |
Abdominal CT | Observation | |
Peritoneal lavage | ||
Retroperitoneum | CT | Angiography |
Angiography | ||
Long bones | Physical examination | Fracture fixation |
Plain x-rays | Surgical ligation | |
Outside the body | Physical examination | Direct pressure |
Surgical ligation |
After management of the circulation follows the assessment of the patient’s neurologic status by calculation of the Glasgow Coma Scale (GCS) score ( Box 66.4 ) ; examination of the pupils for size, reactivity, and symmetry; and determination of sensation and motor function in each of the extremities. Significant abnormalities on the neurologic examination are an indication for immediate cranial computed tomography (CT) scan. Most trauma patients with a diminished GCS score will have nonoperative conditions, but for the few who require operative evacuation of an epidural or subdural hematoma, timeliness of treatment has a strong influence on outcome. Patients with unstable spinal canal injuries and incomplete neurologic deficits will also benefit from early surgical decompression and stabilization.
Eye-Opening Response
- 4
= Spontaneous
- 3
= To speech
- 2
= To pain
- 1
= None
Verbal Response
- 5
= Oriented to name
- 4
= Confused
- 3
= Inappropriate speech
- 2
= Incomprehensible sounds
- 1
= None
Motor Response
- 6
= Follows commands
- 5
= Localizes to painful stimuli
- 4
= Withdraws from painful stimuli
- 3
= Abnormal flexion (decorticate posturing)
- 2
= Abnormal extension (decerebrate posturing)
- 1
= None
The final step in the primary survey is complete exposure of the patient and a head-to-toe search for visible injuries or deformities, including deformities of bones or joints, soft tissue bruising, and any breaks in the skin. The anesthesiologist can assist in this procedure by support of the head and neck, maintenance of the airway, and care in manipulating the spine.
After the primary survey, a more deliberate secondary examination is undertaken that includes a thorough history and physical examination, diagnostic studies, and subspecialty consultation. Any remaining injuries are diagnosed at this time and treatment plans established. Indications for urgent or emergency surgery may also arise during the secondary survey. The presence of a limb-threatening injury due to vascular compromise, compartment syndrome, or a severely comminuted fracture is one such indication. Although the ABCDE issues must be addressed first, a pulseless extremity, compartment syndrome, near-amputation, or massively fractured extremity must go to the OR as soon as the patient is otherwise stable.
Injury Patterns Prompting Urgent Operative Intervention
Fig. 66.2 , an algorithm for prioritizing surgical management in trauma patients, is presented with the understanding that individual situations will vary according to available resources and the patient’s response to therapy. A trauma patient will often arrive at the OR with the need for more than one surgical procedure by more than one surgical service. A trauma patient may have injuries requiring emergency surgery coexisting with injuries that can be repaired at any time. The anesthesiologist plays an important role in determining which procedures to perform, in which order, and which procedures should be postponed until the patient is more stable.

In selected cases with obvious, imminent exsanguination, patients should be directly admitted to the OR, bypassing the ED and radiology suite. Historically, it has been shown that up to a third of preventable trauma deaths may be caused by delays getting to the OR; in one registry study, mortality was increased by 1% for every 3 minutes of delay to laparotomy among hypotensive patients with abdominal injuries. Steele and associates were among the first to describe a “direct to the OR” approach in San Diego, reporting data gathered over a 10-year period. Patients with traumatic cardiac arrest, systolic blood pressure persistently lower than 100 mm Hg, amputation, or uncontrolled external hemorrhage were admitted directly to the OR for resuscitation, regardless of mechanism of injury. These triage criteria had poor sensitivity (24.1%) but high specificity (98%) in identifying patients truly in need of immediate surgery. Observed compared to predicted survival was significantly higher for the “direct to the OR” approach in this observational study. Similarly, Martin and associates reviewed their 10-year data from 2000 to 2009 for direct OR admission of 1407 patients (5% of all admissions) based on an expanded set of criteria including specific mechanisms. After excluding patients who died on arrival (8%), 3.6% died in the OR and overall observed (5%) mortality was significantly lower than predicted (10%). Emergent surgical procedures were started within 30 minutes of arrival in 77% of patients and within 60 minutes in 92%.
Anesthesia in War and Austere Conditions
“While it is evident that the general principles of anesthesia are not affected by the circumstances of war, it is equally evident that it is our duty to assiduously seek those means in anesthesia which are especially suited to the exigencies of battle.”
Although written in 1942, these words are still true today, and many of the principles developed from earlier wars still apply on the modern battlefield or in a large-scale disaster (see also Chapter 68 ). Recent conflicts and events have allowed anesthesiologists, nurse anesthetists, and other providers to help improve management of traumatically injured patients in the areas of anesthesia, resuscitation, and damage control surgery. Management of battlefield casualties typically follows the same flow as outlined earlier, but with special consideration in the areas of prehospital interventions, resuscitation, technologic and logistic support, patient movement, mass casualty management, and surgical interventions. Pain management considerations may also be affected by the nature of the injuries and transport considerations.
Modern advancements in battle armor, prehospital interventions, provision of forward surgical support, and resuscitative strategies have had an impact on survival from combat injuries. During the most recent conflicts in Iraq and Afghanistan, the killed-in-action rate decreased to 13.9% from 20.2% in Vietnam and World War II. This is mirrored by a similar reduction in the case fatality rate. Paradoxically, the ability to get many of the severely wounded patients to a hospital (e.g., rapidly by helicopter) has led to an increase in the “died-of-wounds” rate. Most likely this rate would be even higher if not for improvements in surgical management such as damage control techniques, improved intensive care unit (ICU) care, evolving resuscitation strategies, tactical combat casualty care initiatives, and institution of a theater-wide trauma system approach. More recently, transition from a 2-hour to an aggressive 1-hour target for definitive care for combat casualties has also contributed to a reduction in case fatality and killed-in-action rates.
One of the major advances in battlefield medical support has been the rapid movement of patients out of the theater of operations to more comprehensive medical facilities. Even in the late l960s, wounded soldiers were evacuated out of Vietnam within 3 days of injury. In the most recent conflict, the time from injury in the Middle East until movement to more definitive medical care in Europe or North America is often within 24 to 48 hours. This may include initial in-theater damage control surgery followed by one or two aeromedical evacuation missions lasting up to 12 hours with a critical care air transport team (CCATT). In preparation for such rapid movement, the anesthesiologist must ensure that perioperative interventions such as airway management, pain control, and adequacy of resuscitation are addressed before transfer. In addition, anesthesiologists are frequently assigned to a CCATT as the physician team member based on their overall skill set and ability to provide support en route to critically ill or injured patients. Beyond the support provided during wartime, the CCATT also has proved useful for the movement of critical patients during large-scale disasters such as occurred after Hurricane Katrina in 2005.
Mass casualty situations are common in wartime conditions, although the role of the anesthesiologist will vary depending on the number of patients and requirements for urgent surgical interventions. Given the limited number of anesthesia providers in most combat-related scenarios, often they are not involved in the triage process. If available, however, anesthesia support can enhance emergency airway management, establishment of venous access, and supervision of resuscitative efforts. Only 10% to 20% of arriving casualties require immediate lifesaving interventions, although a much larger percentage will ultimately require surgical procedures. A well-developed trauma system is persistently evolving, and management of mass casualty scenarios will become routine.
Overall, anesthetic management of battlefield casualties is similar to that for patients in a civilian trauma setting; however, many factors must be considered in the perioperative plan for a combat casualty. Environmental considerations such as extremes of temperature, availability of water, contamination with sand, lack of consistent electricity, and other aspects may have to be taken into consideration. Logistic support chains may be long and unable to provide sufficient supplies in the early phases of a conflict. Deployable equipment, such as drawover vaporizer systems or portable anesthesia systems, may be different from those used during peacetime, so predeployment training is vital ( Fig. 66.3 ). In addition, techniques such as total intravenous anesthesia and regional anesthesia or analgesia may be better options making familiarity with their management and associated equipment an imperative (see also Chapter 46 ).

Optimal care of wartime casualties or victims of large disasters requires not only knowledge of a broad range of anesthetic principles and techniques but also the ability to be flexible in the face of a rapidly changing environment. With special training in airway management, provision of anesthesia and sedation, resuscitation, and pain management, anesthesiologists may find themselves involved in triage, emergency management, and perioperative and critical care.
Emergency Airway Management
The American Society of Anesthesiologists (ASA) algorithm for management of difficult airways (see also Fig. 44.1 ) modified for trauma is a useful starting point for the trauma anesthesiologist, whether in the ED or the OR (see also Chapter 44 ). The concept of the algorithm is an important one. The anesthesiologist should have a plan for the initial approach to the airway and for coping with any difficulties that might develop. Fig. 66.4 is a typical algorithm for emergency intubation of an unstable trauma patient. Note that it differs from the ASA algorithm in that reawakening the patient is seldom an option because the need for emergency airway control will presumably remain. Once the decision to obtain a definitive airway is made, efforts will continue until a cuffed tube is in position in the trachea, whether by conventional intubation or via a surgical approach. Failure to commit to a surgical airway soon enough results in bad outcomes more commonly than do complications of a procedure that might have been unnecessary.

Indications
The goal of emergency airway management is to ensure adequate oxygenation and ventilation while protecting the patient from the risks for aspiration. Endotracheal intubation is commonly required and is specifically indicated in the following conditions:
- ▪
Cardiac or respiratory arrest
- ▪
Respiratory insufficiency (see Box 66.2 )
- ▪
Airway protection
- ▪
Need for deep sedation or analgesia, up to and including general anesthesia
- ▪
Ventilation management of patients with space-occupying intracranial lesions and evidence of increased intracranial pressure (ICP)
- ▪
Delivery of a 100% fraction of inspired oxygen (FiO 2 ) to patients with carbon monoxide poisoning
- ▪
Facilitation of the diagnostic workup in uncooperative or intoxicated patients
Approach to Endotracheal Intubation
In general, monitoring standards for airway management should be the same in the ED and OR, including an electrocardiogram (ECG), blood pressure, oximetry, and capnometry. Appropriate equipment, including an oxygen source, bag-valve-mask ventilating system, mechanical ventilator, suction, and a selection of laryngoscope blades (including video laryngoscope), ETTs, and devices for managing difficult tracheal intubations, should be available in any location where emergency intubation is likely, including the ED.
Endotracheal intubation is best accomplished in almost all cases with a modified rapid sequence approach by an experienced clinician. Although concern may exist that the use of neuromuscular blocking drugs and potent anesthetics outside the OR will be associated with a more frequent complication rate, in fact the opposite is more likely correct. Anesthesia and neuromuscular blockade allow the best tracheal intubating conditions on the first approach to the airway, which is advantageous in an uncooperative, hypoxic, or aspirating patient. Attempts to secure the airway in an awake or lightly sedated patient increase the risk for airway trauma, pain, aspiration, hypertension, laryngospasm, and combative behavior. Experienced providers, supported by appropriate monitoring and equipment, have achieved results of medication-assisted intubation outside the OR that are equivalent to those for emergency tracheal intubation within the OR.
Prophylaxis Against Pulmonary Aspiration of Gastric Contents
A trauma patient should always be treated as having a full stomach and at risk for aspiration of gastric contents during induction of anesthesia (see also Chapter 44 ). Reasons include ingestion of food or liquids before the injury, swallowed blood from oral or nasal injuries, delayed gastric emptying associated with the stress of trauma, and administration of liquid contrast medium for abdominal CT scanning.
Cricoid pressure—the Sellick maneuver—has been recommended to be applied continuously during emergency airway management from the time the patient loses protective airway reflexes until ETT placement and cuff inflation are confirmed. The Sellick maneuver consists of elevating the patient’s chin (without displacing the cervical spine) and then pushing the cricoid cartilage posteriorly to close the esophagus. However, cricoid pressure may worsen the laryngoscopic grade of view in up to 30% of patients without providing effective prevention of aspiration of gastric contents. In a prehospital study evaluating the impact of cricoid pressure on subsequent intubation success, discontinuing cricoid pressure usually facilitated intubation of the trachea without worsening the grade of laryngoscopic view. Thus cricoid pressure should be released in the trauma patient if likely to facilitate intubation attempts. The lack of evidence supporting the use of cricoid pressure and its potential to make intubation more difficult led the American Heart Association to recommend discontinuation of its use during cardiac arrest situations. Additionally, the Eastern Association for the Surgery of Trauma Practice Management Guidelines for emergency tracheal intubation have removed it as a class 1 recommendation.
In the traditionally defined rapid sequence induction of anesthesia, any attempt at ventilation between administration of medication and intubation is avoided, presumably because positive-pressure ventilation may force gas into the patient’s stomach, leading to regurgitation and aspiration. Sellick’s original paper described ventilation during cricoid pressure in patients with full stomachs with the belief that cricoid pressure during mask ventilation would prevent gastric inflation. Although this may be true, cricoid pressure reduces tidal volumes, increases peak inspiratory pressure, or prevents ventilation. On the other hand, the increase in oxygen consumption in trauma patients necessitates preoxygenation whenever possible. If preoxygenation is not possible because of facial trauma, decreased respiratory effort, or agitation, rapid desaturation is a possibility. Positive-pressure ventilation during all phases of induction provides the largest possible oxygen reserve during emergency airway management and will help mitigate hypoxia if intubation proves difficult. In this situation, large tidal volumes and high peak inspiratory pressures should be avoided. Application of cricoid pressure during attempts at positive-pressure ventilation should be considered to reduce gastric inflation, but it may prevent effective ventilation in some patients necessitating discontinuation.
Protection of the Cervical Spine
Standard practice dictates that all victims of blunt trauma be assumed to have an unstable cervical spine until this condition is ruled out. The airway management of these patients receives much attention from anesthesiologists because direct laryngoscopy causes cervical motion, with the theoretical potential to exacerbate spinal cord injury (SCI). Stabilization of the cervical spine will generally occur in the prehospital environment, with the patient already having a rigid cervical collar in place. This collar may be kept in place for several days before the complete gamut of tests to rule out cervical spine instability have been completed (see later discussion). The presence of an “uncleared” cervical spine mandates the use of manual in-line stabilization without application of traction throughout any attempt at intubation. This approach allows removal of the front of the cervical collar to facilitate wider mouth opening and jaw displacement; however, this may slightly lengthen the time to intubation and worsen laryngeal visualization during laryngoscopy compared to intubation attempts without manual in-line stablilization. Manual in-line stabilization has been tested through considerable clinical experience and is the standard of care in the ATLS curriculum. Emergency awake fiberoptic intubation, though requiring less manipulation of the neck, is generally very difficult because of airway secretions and hemorrhage, rapid desaturation, and lack of patient cooperation; and is best reserved for cooperative patients with known cervical instability under controlled conditions. Indirect video laryngoscopy offers the potential to enjoy the best of both worlds: an anesthetized patient and decreased cervical motion. In comparative studies of direct laryngoscopy, video laryngoscopy, fiberoptic intubation, blind nasal intubation, or cricothyrotomy—in patients with known cervical cord or spine injuries, or both—there is no difference in neurologic deterioration with technique used, and no clear evidence that direct laryngoscopy worsens outcome.
Personnel
Emergency endotracheal intubation requires more assistance than an intubation performed under controlled conditions (see also Chapter 6 ). Three providers are required to ventilate the patient and manage the airway, administer medications, and provide manual in-line stabilization (if indicated); a fourth provider may be needed to provide cricoid pressure if deemed appropriate. Fig. 66.5 is an illustration of this approach. Additional assistance may be required to restrain a patient who is combative because of intoxication or traumatic brain injury (TBI).

The immediate presence of a surgeon or other physician who can expeditiously perform a cricothyroidotomy is desirable. Even if a surgical airway is not required, additional experienced hands may prove useful during difficult intubations. The surgeon may also wish to inspect the upper airway during laryngoscopy or video laryngoscopy if trauma to the face or neck has occurred. Urgent tube thoracostomy may prove necessary in some trauma patients to treat a tension pneumothorax that develops with the onset of positive-pressure ventilation.
Anesthetics and Induction of Anesthesia
Any intravenous anesthetic administered to a trauma patient in hemorrhagic shock may cause profound hypotension and even cardiac arrest because of inhibition of circulating catecholamines. Although propofol is the mainstay of intravenous induction in the OR, its use in trauma patients is problematic because of its vasodilatory and negative inotropic effects. Moreover, pharmacokinetic and pharmacodynamic studies in a swine hemorrhagic shock model suggest a significant reduction in propofol dosage of more than 80% to achieve the targeted effect site concentration. Unfortunately, there is no corresponding clinical data on the impact of reduced propofol dosing in the setting of hemorrhagic shock on recall and awareness.
The most commonly used induction agent in the United States in the ED or trauma setting is etomidate. Etomidate administered in a range of 0.2 to 0.3 mg/kg is associated with hemodynamic stability and has an onset/duration profile like that of succinylcholine. Its safety for use in rapid sequence induction in trauma patients has been challenged although these studies are largely retrospective with the potential for selection bias and other methodologic deficiencies. Although etomidate is associated with transient adrenal cortical suppression after a single dose, this appears not to be clinically significant when a single dose is used for induction for intubation in both trauma and mixed surgical-medical patients undergoing rapid sequence induction. Etomidate can cause myoclonic jerks during its onset, but use of a rapidly acting neuromuscular blocking agent, such as succinylcholine, mitigates this effect substantially.
Ketamine is also a frequently used induction agent for hypotensive trauma patients due to its centrally mediated increase in sympathetic tone and catecholamine release. Its use in patients with concomitant TBI has been questioned based on older reports of associated ICP elevation. More recent analysis, however, suggests that the preservation of cerebral perfusion by maintenance of mean arterial blood pressure in hemodynamically unstable patients is more important than any theoretical risk to the brain caused by ketamine’s tendency to increase cerebral activity and ICP. Some investigators have also raised concern that the psychotropic effects associated with ketamine may increase the risk of acute- and posttraumatic stress disorders in trauma patients, although this was not found in a study examining its intraoperative use in burn patients. Of more concern is the potential for barriers to its use based on institutional dispensing, tracking, and documentation procedures preventing timely access to ketamine. When barriers exist limiting its availability, ketamine may not be as readily available in the emergency setting compared to other induction agents. Due to its abuse potential, consideration has been made to reclassify ketamine as a Schedule 1 drug, potentially placing further barriers to its availability. Overall, ketamine continues to be a very commonly used drug for rapid sequence induction in the trauma patient.
Hypotension will likely develop in patients with hypovolemia with the administration of any anesthetic because of interruption of compensatory sympathetic outflow and the sudden change to positive-pressure ventilation. Previously healthy young patients can lose up to 40% of their blood volume before hypotension occurs, thereby leading to potentially catastrophic circulatory collapse with induction of anesthesia, regardless of the anesthetic chosen. The dose of anesthetic must be decreased in the presence of hemorrhage, including no anesthetic at all in patients with life-threatening hypovolemia. Rapid sequence induction of anesthesia and endotracheal intubation may proceed with muscle relaxants alone, although onset time may be prolonged in a patient with circulatory impairment. Subsequent patient recall of intubation and emergency procedures is highly variable and affected by the presence of coexisting TBI, intoxication, and the depth of hemorrhagic shock (see also Chapter 9 ). Decreased cerebral perfusion inhibits memory formation but cannot be reliably associated with any particular blood pressure or chemical marker. Administration of 0.2 mg of scopolamine (a tertiary ammonium vagolytic) may inhibit memory formation in the absence of anesthetic drugs in this situation, but it may interfere with subsequent neurologic examination because of its long half-life. Small doses of midazolam will reduce the incidence of patient awareness but also can contribute to hypotension. Although recall of ED and OR events is not unusual in this circumstance, anesthesia provider liability appears to be limited; an analysis of intraoperative awareness lawsuits in the ASA Closed Claims Database revealed no claims related to surgery in trauma patients.
Neuromuscular Blocking Drugs
Succinylcholine remains the neuromuscular blocker with fastest onset—less than 1 minute—and shortest duration of action—5 to 10 minutes. These properties make it popular for rapid sequence induction of anesthesia. Although the use of succinylcholine may allow return of spontaneous respiration before the development of significant hypoxia in the “cannot intubate, cannot ventilate” situation, this is unlikely to be of benefit in an emergency intubation in a trauma patient. The anesthesiologist should not rely on return of spontaneous breathing in time to salvage a difficult airway management problem but should instead proceed with efforts to obtain a definitive airway, including cricothyroidotomy if other possibilities have been exhausted.
Administration of succinylcholine is associated with several adverse consequences. Increases in serum potassium of 0.5 to 1.0 mEq/L are expected, but in certain patients K + may increase by more than 5 mEq/L. A hyperkalemic response is typically seen in burn victims and those with muscle pathology secondary to direct trauma, denervation (as with SCI), or immobilization. Hyperkalemia is not seen in the first 24 hours after these injuries, and succinylcholine may be used safely for acute airway management. Patients at risk are those with underlying pathologic processes before their traumatic event or those undergoing subsequent surgery in the weeks to months after injury.
Succinylcholine causes an increase in intraocular pressure and should be used cautiously in patients with ocular trauma. Succinylcholine may also increase ICP, making its use in patients with brain trauma controversial. In both these cases, however, hypoxia and hypercapnia may be as damaging as the transient increase in pressure caused by the drug. If the use of succinylcholine will lead to faster intubation, its benefits may outweigh its risks. The provider must weigh the use of succinylcholine in each individual situation based on acuity, anticipated speed with which intubation can be accomplished, and likelihood that hypoxia will develop.
Alternatives to succinylcholine include rocuronium 0.9 to 1.2 mg/kg and vecuronium 0.1 to 0.2 mg/kg. Rocuronium is preferred because it has a more rapid onset of action than that of vecuronium. With the availability of sugammadex, a rapid onset selective binding agent for rocuronium, rapid sequence induction and intubation with rocuronium followed by reversal with sugammadex allows for more rapid return of spontaneous ventilation than with succinylcholine. Basically, the combination of rocuronium and sugammadex provides all the advantages of succinylcholine, but none of the complications. Because these drugs have no significant cardiovascular toxicity, large doses can be administered to achieve rapid (1- to 2-minute) paralysis.
Specific situations will always exist in which maintaining spontaneous ventilation during intubation is the preferred way to proceed. If patients can maintain their airway temporarily but have clear indications for an artificial airway (e.g., penetrating trauma to the trachea), slow induction with ketamine or inhaled sevoflurane through cricoid pressure will enable placement of an ETT without compromising patient safety.
Adjuncts to Endotracheal Intubation
Equipment to facilitate difficult intubation should be readily available wherever emergency airway management is performed. The equipment available depends on the preferences of the anesthesiologist; the usefulness of most special equipment depends more on previous experience than on any intrinsic properties of the device. Certain items deserve mention, however, because they are frequently cited as aids to management of a difficult airway.
The gum elastic bougie, or intubating stylet, is an inexpensive and easily mastered adjunct for management of a difficult airway. The stylet is placed through the vocal cords via direct laryngoscopy, and the ETT is then advanced over the stylet into the trachea. Placement of the bougie is easier than direct placement of an ETT because of both its smaller diameter and the ability of an experienced operator to feel it enter the trachea even when the glottic opening cannot be visualized. The bougie is passed under the epiglottis and gently advanced; if resistance is met, the bougie is withdrawn, rotated slightly, and advanced again. In this fashion the anesthesiologist can blindly palpate the larynx until the bougie advances into the trachea. The bougie also can be used with direct and indirect video laryngoscopy systems and is especially useful in the ED when the sniffing position cannot be used because of uncertainty about the cervical spine.
Supraglottic airway (SGA) devices, such as the laryngeal mask airway (LMA) (LMA North America, San Diego, CA), are recommended in the ASA algorithm for management of a patient with a difficult airway. The LMA can be used as a guide for intubation when an unsuspected difficult intubation is encountered in a trauma patient; an ETT may be placed blindly through the lumen of the LMA and into the trachea, or a fiberoptic bronchoscope may be used to guide the tube through the LMA. The LMA is an appropriate rescue device for a difficult airway situation in trauma, provided no major anatomic injury or hemorrhage is present in the mouth and larynx. In our practice the LMA has most commonly been used as a bridge to emergency tracheostomy because it allows more controlled conditions than a cricothyroidotomy.
Facial and Pharyngeal Trauma
Trauma to the face and upper airway poses difficulties for the anesthesiologist. Serious skeletal derangements may be masked by apparently minor soft tissue damage. Failure to identify an injury to the face or neck can lead to acute airway obstruction secondary to swelling and hematoma. Laryngeal edema is also a risk in patients who have suffered chemical or thermal injury to the pharyngeal mucosa. Intraoral hemorrhage, pharyngeal erythema, and change in voice are all indications for early intubation.
In general, both maxillary and mandibular fractures will make ventilation by mask more difficult, whereas mandibular fractures will make endotracheal intubation easier. Palpation of the facial bones before manipulation of the airway will alert the anesthetist to these possibilities. Patients with injuries to the jaw and zygomatic arch often have trismus. Although the trismus will resolve with the administration of neuromuscular blocking agents, preinduction assessment of airway anatomy may be difficult. Bilateral mandibular fractures and pharyngeal hemorrhage may lead to upper airway obstruction, particularly in a supine patient, although intubation may be easier because of loss of skeletal resistance to direct laryngoscopy. A patient arriving at the ED in the sitting or prone position because of airway compromise is best left in that position until the moment of anesthetic induction and intubation.
The Field Placed Airway
In many settings, prehospital providers work under protocols that allow for advanced airway management including intubation and placement of an SGA. It is recommended that the individuals responsible for airway management at the ED level be familiar with the protocols and devices available in their region to facilitate early assessment and airway exchange if indicated. All intubations done in the field should be immediately confirmed using capnometry. In the event of cardiac arrest and inability to confirm correct placement by observation of end-tidal CO 2 , direct laryngoscopy or video laryngoscopy can be employed to visualize position of the ETT. When an SGA device is used in the prehospital setting, the positioning and ability to ventilate should be confirmed by capnometry, presence of breath sounds, and chest movement. If there is adequate ventilation, replacement of the SGA can be delayed during the initial evaluation.
Since the SGA is not considered a secured definitive airway, it should be changed out to a cuffed ETT as soon as practical. When faced with changing out an SGA, preparation is the key concept. Unfortunately, there is a paucity of literature discussing the technique of “changing out” an SGA with no single preferred method in the trauma patient. This procedure can be challenging with increased risk of losing the patient’s airway. Prior to deciding which technique to use, it is extremely important to have a discussion with the prehospital care provider that managed the patient’s airway in the field to determine the following information:
- ▪
What was the reason for placing an SGA?
- ▪
Were attempts at oral or nasal intubation made? How many?
- ▪
What laryngoscope/video laryngoscope was used?
- ▪
What anatomy was visualized during attempts?
- ▪
What, if any, medications were administered during the attempts?
The answers to these questions may suggest a difficult airway prompting more guarded management during the exchange process. The three options for exchange in the trauma setting are: (1) remove the SGA and replace under direct or video laryngoscopy, (2) use the SGA to place an ETT or exchange catheter, or (3) proceed to a surgical airway. The second option will largely be guided by the specific SGA, channel size, and available equipment. Of note, there have been reports of pharyngeal, glottic, and lingual edema with the use of various SGA devices likely secondary to exaggerated anatomical distortion and indirect vascular compression. It is unclear whether this is an anticipated finding in some patients with proper placement and inflation of the proximal oropharyngeal cuff or due to overinflation of the cuff. In the only published series of patients presenting with prehospital placement of the King LT (S)-D (King Systems; Noblesville, IN), 7 of 9 trauma patients ultimately underwent tracheostomy in the OR due to concerns for concomitant facial trauma, observed upper airway edema, or failed attempts at direct laryngoscopy.
On occasion, prehospital personnel will perform a blind nasotracheal intubation in the spontaneously ventilating trauma patient. Successful exchange of this nasotracheal tube on arrival to the ED is consistently easier in comparison with the SGA. Nasotracheally placed tubes in the field are usually a result of the prehospital providers not being credentialed for drug-facilitated intubation. As a result, most of these patients have not had laryngoscopy performed and therefore are less likely to have airway edema from intubation attempts. Again, in this scenario, it is prudent to facilitate the intubation with muscle relaxation and adequate sedation/anesthesia prior to attempting laryngoscopy. A video laryngoscope is preferred as it allows for an expanded view of the glottis with visualization of the nasotracheal tube prior to removal of the nasal tube. Simple exchange under direct or video visualization with an oral ETT is usually all that is required. Once again, it cannot be overemphasized that a bougie be readily available in these situations for rapid placement into the glottis with various sized ETTs readily available. If one is unable to adequately visualize the glottis with laryngoscopy, it is recommended that the nasotracheal tube be left in place for a period to allow for either a controlled tracheostomy or swelling to improve and an exchange for an oral tube made later.
Resuscitation from Hemorrhagic Shock
Resuscitation refers to restoration of normal physiology after injury. Resuscitation from hemorrhagic shock refers specifically to restoration of normal circulating blood volume, normal vascular tone, and normal tissue perfusion. Resuscitation begins immediately after injury, via the patient’s own compensatory mechanisms, and continues through the prehospital, ED, OR, and ICU phases of care.
Pathophysiology of Hemorrhagic Shock
During massive hemorrhage an imbalance occurs between systemic oxygen delivery and oxygen consumption. Blood loss leads to hemodynamic instability, coagulopathy, decreased oxygen delivery, decreased tissue perfusion, and cellular hypoxia. The initial response to hemorrhage takes place on the macrocirculatory level and is mediated by the neuroendocrine system. Decreased arterial blood pressure leads to vasoconstriction and release of catecholamines to preserve blood flow to the heart, kidney, and brain, whereas other regional beds are constricted. Pain, hemorrhage, and cortical perception of traumatic injuries lead to the release of hormones and other inflammatory mediators, including renin, angiotensin, vasopressin, antidiuretic hormone, growth hormone, glucagon, cortisol, epinephrine, and norepinephrine. This response sets the stage for the microcirculatory response that follows.
Individual ischemic cells respond to hemorrhage by taking up interstitial fluid, thus further depleting intravascular fluid. Cellular edema may choke off adjacent capillaries and result in the no-reflow phenomenon, which prevents reversal of ischemia even in the presence of adequate macroperfusion. Ischemic cells produce lactate and free radicals, which accumulate in the circulation if perfusion is diminished. These compounds cause direct damage to the cell and form the bulk of the toxic load that washes back to the central circulation when flow is reestablished. Ischemic cells also produce and release inflammatory factors—prostacyclin, thromboxane, prostaglandins, leukotrienes, endothelin, complement, interleukins, tumor necrosis factor, and others. Fig. 66.6 shows the generalized inflammatory response to shock, with an emphasis on immune system amplification. This inflammatory response, once begun, becomes a disease process independent of its origin. Such alterations lay the foundations for subsequent development of multiple organ failure, a systemic inflammatory process that leads to dysfunction of different vital organs and accounts for high mortality rates.

Specific organ systems respond to traumatic shock in specific ways. The CNS is the prime trigger of the neuroendocrine response to shock, which maintains perfusion to the heart, kidney, and brain at the expense of other tissues. Regional glucose uptake in the brain changes during shock. Reflexes and cortical electrical activity are both depressed during hypotension; these changes are reversible with mild hypoperfusion but become permanent with prolonged ischemia. Failure to recover preinjury neurologic function is a marker for a poor prognosis, even when normal vital signs are restored.
The kidney and adrenal glands are prime responders to the neuroendocrine changes associated with shock and produce renin, angiotensin, aldosterone, cortisol, erythropoietin, and catecholamines. The kidney maintains glomerular filtration in the face of hypotension by selective vasoconstriction and concentration of blood flow in the medulla and deep cortical area. Prolonged hypotension leads to decreased cellular energy and an inability to concentrate urine (renal cell hibernation), followed by patchy cell death, tubular epithelial necrosis, and renal failure.
The heart is preserved from ischemia during shock because of maintenance of or even an increase in nutrient blood flow, and cardiac function is well preserved until the late stages. Lactate, free radicals, and other humoral factors released by ischemic cells all act as negative inotropes and, in a bleeding patient, may produce cardiac dysfunction as the terminal event in the shock spiral. A patient with cardiac disease or direct cardiac trauma is at great risk for decompensation because a fixed stroke volume inhibits the body’s ability to increase blood flow in response to hypovolemia and anemia. Tachycardia is the patient’s only option, with potentially disastrous consequences on the oxygen supply-demand balance in the heart. Therefore shock in older patients may be rapidly progressive and not respond predictably to fluid administration.
The lung is the filter for the inflammatory by-products of the ischemic body. Immune complex and cellular factors accumulate in pulmonary capillaries and lead to neutrophil and platelet aggregation, increased capillary permeability, destruction of lung architecture, and acute respiratory distress syndrome (ARDS). The lung is the sentinel organ for the development of multiple organ dysfunction (MOD) in a patient with traumatic shock. Pure hemorrhage, in the absence of hypoperfusion, does not produce pulmonary dysfunction. Traumatic shock is obviously more than just a hemodynamic disorder.
The gut is one of the earliest organs affected by hypoperfusion and may be one of the prime triggers of MOD. Intense vasoconstriction occurs early and frequently leads to a no-reflow phenomenon, even when the macrocirculation is restored. Intestinal cell death causes a breakdown in the barrier function of the gut that results in increased translocation of bacteria to the liver and lung, thereby potentiating MOD and ARDS.
The liver has a complex microcirculation and may experience reperfusion injury during recovery from shock. Hepatic cells are also metabolically active and contribute to the ischemic inflammatory response and to irregularities in blood glucose. Failure of synthetic function of the liver after shock is almost always lethal.
Skeletal muscle is not metabolically active during shock and tolerates ischemia better than do the other organs. The large mass of skeletal muscle, though, makes it important in the generation of lactic acid and free radicals from ischemic cells. Sustained ischemia of muscle cells leads to an increase in intracellular sodium and free water, with an aggravated depletion of fluid in the vascular and interstitial compartments.
More recently, there appears to be a role for endothelial injury in the pathophysiology of hemorrhagic shock. The endothelium is one of the “largest” organs in the body with a surface area of up to 5000 m 2 . Under normal conditions the endothelium is anticoagulated by a number of natural anticoagulant systems including the negatively charged luminal surface layer, the glycocalyx, which is rich in heparinoids and interacts with antithrombin. As noted earlier, increasing injury severity and shock lead to high catecholamine levels which can directly injure the endothelium. This is evidenced by an increase in syndecan-1 levels, a marker of endothelial glycocalyx degradation. The release of heparin-like substances in the glycocalyx may also contribute to endogenous heparinization and the coagulopathy of trauma (discussed in the next section). The net effect of this endothelial injury results in glycocalyx shedding, breakdown of tight junctions with capillary leakage, and a pro-coagulant microvasculature that further reduces oxygen delivery due to increased tissue pressure and microvascular thrombosis.
Acute Traumatic Coagulopathy
During resuscitation from hemorrhagic shock, attention must also be directed to avoidance or correction of coagulopathy. In patients with identical injury severity scores (ISS), the presence of coagulopathy is associated with at least a twofold to fourfold increase in mortality ; thus current resuscitation strategies focus on coagulopathy and shock during the initial and subsequent resuscitation. The presence of trauma-induced coagulopathy—defined as a “multifactorial, global failure of the coagulation system to sustain adequate hemostasis after major trauma”—has an endogenous component linked to hypoperfusion and tissue injury referred to as acute traumatic coagulopathy (ATC). A proposed mechanism for the development of ATC is endothelial activation of protein C secondary to the traumatic inflammatory response described earlier. Activated protein C (APC) is generated by thrombomodulin-thrombin complex production as a result of tissue hypoperfusion. APC inactivates factors Va and VIIIa, and in combination with the reduction in thrombin availability for fibrin formation, supports the development of ATC. Additionally, degradation of the endothelial glycocalyx as a result of hypoperfusion may play a supporting role in ATC.
By clinical definition, ATC starts with the early presence of reduced clot strength as demonstrated by viscoelastic monitoring and changes in laboratory-based coagulation testing associated with an increase in mortality and likelihood of receiving a massive transfusion. Davenport and colleagues proposed a clot amplitude threshold of less than 35 mm at 5 minutes using rotational thromboelastometry (ROTEM) (Tem Innovations, Munich, Germany) analysis, which predicted the subsequent need for a massive transfusion with a detection rate of 77% and false-positive rate of 13% (see also Chapter 50 ). Similar results have been obtained using RapidTEG (Haemonetics, Niles, IL) viscoelastic testing. Laboratory-based coagulation testing is of limited utility in early detection of ATC because of time considerations. However, Frith and associates found patients with a prothrombin ratio of greater than 1.2 on admission to have larger transfusion requirements and increased mortality. Regardless of the means used to detect coagulopathy in the severely traumatized patient undergoing resuscitation for hemorrhagic shock, the resuscitation itself should include consideration for early treatment of ATC.
In addition to the described cascade, hyperfibrinolysis occurs in some of the more severely injured patients and contributes to ATC. The mechanism behind this early fibrinolysis is not clearly understood but may be related to hypoperfusion-induced APC formation resulting in the consumption of plasminogen activator inhibitor. The latter normally serves to downregulate tissue plasminogen activator, which promotes fibrin clot degradation. The reported incidence of hyperfibrinolysis varies significantly based on the methods and cutoffs used to diagnose fibrinolysis, but its presence is clearly associated with the increased mortality and transfusion requirements seen with ATC.
Assessment of the Hemostatic System
Given the importance of coagulopathy in the severely injured trauma patient, assessment of the hemostatic system is essential in the early phases of management. This is best accomplished by collecting and integrating information from the four pillars of perioperative coagulation monitoring: (1) medical history, (2) clinical presentation, (3) standard laboratory-based coagulation tests, and (4) viscoelastic monitoring.
Obtaining a focused patient medical history is one of the key pieces for the evaluation of the individual bleeding risk. In some settings, standardized assessments and specific questionnaires can be deployed to raise sensitivity and specificity, resulting in superior detection of hemostasis issues compared to routine coagulation studies alone. The assessment of the clinical phenotypes of bleeding is critical for the differential diagnosis and enables the clinician to differentiate and semi-quantify the underlying coagulation disorder in a very simple and quick fashion. The clinical assessment also helps to put in context abnormal coagulation studies, so that clinical interventions are not based on laboratory values alone. The clinical assessment aims to differentiate whether the cause of bleeding is “surgical” or “nonsurgical.” The latter is characterized by a more diffuse pattern. Standard laboratory coagulation tests typically include prothrombin time, international normalized ratio, activated partial thromboplastin time, and platelet count. In some institutions, other laboratory values such as fibrinogen levels, factor XIII, and thrombin time may be part of a routine laboratory coagulation battery. Standard coagulation tests alone play a limited role in the initial diagnostic steps of patients with deranged hemostasis due to trauma. When used in isolation, the standard tests come with significant limitations regarding the detection of extent and origin of a given patient’s coagulopathy. These tests allow only the determination of certain questions and are of restricted value. The key restrictions of standard coagulation tests are: delayed results in a dynamic situation, lack of validation, and inability to detect both hyperfibrinolysis and hypercoagulability. A recent metaanalysis concluded that standard plasma coagulation tests represent historically established parameters that are not fully supported by evidence for the management of perioperative coagulopathic bleeding. This is where viscoelastic monitoring as the fourth pillar of perioperative coagulation assessment brings added value. Viscoelastic coagulation monitoring devices like RapidTEG and ROTEM assess the whole coagulation process from initial thrombin generation to maximum clot formation up to clot lysis. In contrast to the standard laboratory coagulation tests, TEG and ROTEM have been better validated for the diagnosis of coagulopathy in trauma patients. These tests can significantly improve the coagulation assessment and management of a given patient; and they may reduce unnecessary administration of procoagulant substances such as platelets, fresh frozen plasma (FFP), and coagulation factor concentrates. The rapid turnaround for results may further enable the anesthesiologist to distinguish between a surgical cause of bleeding and trauma-associated coagulopathy.
General Approach to Resuscitation
Fluid administration is the cornerstone of resuscitation (see also Chapter 47, Chapter 87 ). Intravascular volume is lost to hemorrhage, uptake by ischemic cells, and extravasation into the interstitial space. Administration of intravenous fluids will predictably increase cardiac output and arterial blood pressure in a hypovolemic trauma patient. The ATLS curriculum initially advocated rapid infusion of up to 2 L of warmed isotonic crystalloid solution in any hypotensive patient, with the goal of restoring normal arterial blood pressure. More recently, this has been revised to recognize the importance of a balanced resuscitation with elimination of the emphasis on a more aggressive approach. The current recommendation suggests initiation of resuscitation with 1 L of crystalloid and earlier use of blood and blood products for patients in shock.
The change in ATLS recommendations recognizes that aggressive crystalloid fluid resuscitation during active hemorrhage may be counterproductive. Dilution of red cell mass reduces oxygen delivery and contributes to hypothermia and coagulopathy. Increased arterial blood pressure may lead to increased bleeding because of disruption of clots and reversal of compensatory vasoconstriction. The result of aggressive fluid administration is often a transient increase in arterial blood pressure, followed by increased bleeding, another episode of hypotension, and the need for more volume administration. This vicious circle has been recognized since the First World War and remains a complication of resuscitation therapy today. The ATLS manual characterizes such patients as “transient responders” with active, ongoing hemorrhage. Resuscitation of these patients should be considered in the following three phases ( Table 66.2 ):
- ▪
Phase 1, Uncontrolled Hemorrhage: ongoing active bleeding with focus on damage control with pragmatic resuscitation;
- ▪
Phase 2, Controlled Hemorrhage: major bleeding sources under control with focus on goal-directed and tailored management of coagulopathy and resuscitation;
- ▪
Phase 3, Restoration of Physiology: hemorrhage has been fully controlled with focus on end-organ perfusion and optimization of physiologic state.
Phase 1 | Phase 2 | Phase 3 | |
---|---|---|---|
Clinical status |
|
|
|
Clinical priorities |
|
|
|
Blood products |
|
|
|
Crystalloids/colloids |
|
|
|
Special points |
|
|
|
Managing late resuscitation (phase 3) is driven by end-point targets and consists of giving enough fluid to optimize oxygen delivery. Early resuscitation (phase 1) is much more complex because the risks associated with aggressive intravascular volume replacement ( Box 66.5 ), including the potential for exacerbating hemorrhage and prolonging the crisis, must be weighed against the risk for hypoperfusion and ischemia. These phases do not always have distinct transitions and tend to occur as gradual transitions from initial presentation to the OR and ultimately to the ICU.
Increased blood pressure
Decreased blood viscosity
Decreased hematocrit
Decreased clotting factor concentration
Greater transfusion requirement
Disruption of electrolyte balance
Direct immune suppression
Premature reperfusion
Increased risk for hypothermia
Phase 1: Uncontrolled Hemorrhage
During the initial phase of management, the goal in trauma patients with massive hemorrhage requiring an emergent surgical procedure in the OR is to stop the bleeding as soon as possible. In this setting, there is little opportunity to perform additional studies, await test results, or evaluate for perioperative optimization. The role of the anesthesia team is to help achieve hemostasis as quickly as possible, while bridging the patient’s physiologic status to allow for surgical stabilization. In phase 1, the general approach employs the concept of damage control resuscitation (DCR). DCR combines an empiric hemostatic resuscitation strategy in combination with permissive hypotension during surgical or angioembolization control of ongoing hemorrhage. In combination with surgical approaches incorporating damage control techniques, the initial goal of controlling hemorrhage, physiologic stabilization, and correction of coagulopathy allows for more definitive surgical interventions later.
Permissive Hypotension
In the setting of trauma, “permissive” rather than “deliberate” hypotension is controversial and has been the focus of numerous laboratory and clinical research efforts. Deliberate hypotensive management is an accepted standard of anesthetic care for elective surgical procedures such as total joint replacement, spinal fusion, radical neck dissection, reconstructive facial surgery, and major pelvic or abdominal procedures. Tolerating some degree of hypotension in the trauma patient during or prior to control of hemorrhage has been more controversial.
In 1965, Shaftan and colleagues published the results of a study of coagulation in dogs that demonstrated that the formation of a soft extraluminal clot limits bleeding after arterial trauma. This study compared the quantity of blood lost from a standardized arterial injury under a variety of conditions. The least blood loss occurred in hypotensive animals (whether hypotensive from hemorrhage or from administration of a vasodilator), followed by the control group and then vasoconstricted animals. The largest amount of blood was lost in animals that underwent vigorous reinfusion during the period of hemorrhage.
Laboratory data have shown the benefits of limiting intravascular fluid volumes and blood pressure in actively hemorrhaging animals. In the most sophisticated models, direct assessment of cardiac output and regional perfusion showed no difference between moderate-volume or large-volume resuscitation in terms of cardiac output, arterial blood pressure, or regional perfusion of the heart, kidneys, and intestines. Burris and co-workers studied both conventional resuscitation fluids and various combinations of hypertonic saline and dextran and found that rebleeding was correlated with higher mean arterial pressure (MAP) and that survival was best in groups resuscitated to lower than normal MAP. The optimum target blood pressure for resuscitation varied with the composition of the fluid used. A 1994 consensus panel on resuscitation from hemorrhagic shock noted that mammalian species are capable of sustaining MAP as low as 40 mm Hg for periods as long as 2 hours without deleterious effects. The panel concluded that spontaneous hemostasis and long-term survival were maximized by reduced administration of resuscitation fluids during the period of active bleeding to keep perfusion only just above the threshold for ischemia.
Hypotensive resuscitation has increasingly been accepted as a component of early DCR to avoid excessive administration of fluid until control of active hemorrhage has been achieved. This strategy uses less fluids and blood products during the early stages of treatment for hemorrhagic shock compared with the standard of care. Hypotensive resuscitation has also been shown to be feasible and safe during early resuscitation of trauma patients in the prehospital and hospital settings. Early trials suggested outcomes were improved in patients with penetrating trauma with no evidence for worsened outcomes in those with blunt trauma.
Initial work by Bickell and colleagues in 1994 randomized victims of penetrating torso trauma to one of two treatment groups: standard of care (up to 2 L of crystalloid infused in the prehospital setting) or delayed resuscitation (no fluid until the patient reached the OR). This well-managed 37-month study eventually included 598 patients. Average times of transport and care were 30 minutes from injury to the ED and then 50 minutes before reaching the OR; the fluid-restricted group received an average of about 0.8 L of fluid during this time. The immediate-resuscitation group received an average of 2.5 L of crystalloid and 130 mL of blood over this same period. Although substantially different during the period of study, blood pressure on arrival at the OR was similar in both groups, which the authors took as evidence that the unresuscitated group had achieved spontaneous hemostasis. Survival to hospital discharge in the delayed-resuscitation group was significantly improved over the immediate-resuscitation group (70% vs. 62% [ P < .04]). No data were presented on the conduct of anesthesia after arrival at the OR, but before control of hemorrhage, or on the incidence of rebleeding after volume loading and induction of anesthesia in patients who had achieved hemostasis preoperatively.
A retrospective review of trauma admissions to the Los Angeles Medical Center published in 1996 supported these findings. Patients brought to the hospital by private conveyance fared substantially better than those delivered by paramedics, even with high levels of injury severity. Further corroboration was provided by retrospective examination of outcomes in a population of hemorrhaging trauma patients who received fluids via a commercial rapid infusion system (RIS, Haemonetics, Niles, IL) during initial resuscitation. The survival rate of this group was compared with that predicted by the institution’s trauma registry. Patients who received fluid by the rapid infusion system, when compared with case-matched controls, had a survival rate of only 56.8% versus 71.2% for patients of similar age with similar injuries ( P < .001).
This retrospective review was followed in 2002 by the second prospective trial of delayed resuscitation in trauma patients. Patients with systolic blood pressure lower than 90 mm Hg and clinical evidence of blood loss were randomized to fluid resuscitation titrated to a systolic blood pressure of 100 mm Hg (normal group) or 70 mm Hg (study group) until the end of surgical interventions to control hemorrhage. The results of this study are summarized in Table 66.3 . As in the Bickell study, hypotension allowed spontaneous resolution of hemorrhage and autoresuscitation; blood pressure would increase without exogenous fluid administration once hemostasis was achieved. The typical patient began with a low initial pressure, followed by recovery to the vicinity of the target, overshoots and undershoots as bleeding and fluid administration continued, and an eventual rise above the target when the hemorrhage resolved, even in the absence of further fluid administration ( Fig. 66.7 ). The 93% overall survival rate in this study was more frequent than predicted from historical data and substantially more frequent than seen in Bickell’s group. This reflects the exclusion of patients who died in the prehospital phase or arrived at the trauma resuscitation unit in a moribund condition. It may also reflect improvements in overall care, an observation effect (i.e., patients in both groups received better care than did patients not in the study), or a bias in subject recruitment. Over the first 24 hours, lactate and base deficit cleared to normal in both groups and required similar amounts of fluid and blood products, thus suggesting that both groups were reaching an equivalent resuscitation endpoint. The authors concluded that administration of fluids to an actively hemorrhaging patient should be titrated to specific physiologic endpoints, with the anesthesiologist navigating a course between the Scylla of increased hemorrhage and the Charybdis of hypoperfusion.
Conventional | Hypotensive | Total | |
---|---|---|---|
Patients enrolled | 55 | 55 | 110 |
Male | 46 | 41 | 87 |
Blunt trauma | 22 | 31 | 53 |
Penetrating trauma | 33 | 24 | 57 |
Injury severity score | 19.65 | 23.62 ( P = .11) | |
Predicted survival | 0.94 | 0.90 ( P = .19) | |
SBP during study period | 114 | 100 ( P < .001) | |
Survived to discharge | 51 | 51 | |
Died | 4 | 4 |

In a more recent study, Morrison and colleagues compared a hypotensive resuscitative strategy targeting a MAP of 50 mm Hg to one targeting a MAP of 65 mm Hg with conventional resuscitation for patients requiring emergent surgery. In a preliminary report, they found that patients in the hypotensive resuscitation group had a lower, early postoperative mortality, a reduced incidence of coagulopathy, and lower mortality related to coagulopathy. Subsequently, this study was terminated early for futility and clinical equipoise with no significant survival advantage and similar rates of coagulopathy, renal failure, and infection between the low (50 mm Hg) and high (65 mm Hg) MAP target groups. Of note, this study excluded blunt trauma patients included in the preliminary report. Despite not identifying a difference in major outcomes, the authors did make several interesting observations. First, the need for vasopressors use in the first hour of surgery was greater in the high MAP group. Although this group also received more fluid, it was not statistically significant. Second, the intraoperative MAP was not significantly different between the low and high MAP groups (65.5 ± 11.6 mm Hg vs. 69.1 ± 13.8 mm Hg, P = .07, respectively). Taken in combination with available data and experience, the current consensus at major trauma centers is to allow for hypotensive resuscitation in the setting of DCR barring specific caveats discussed below. While the optimal arterial blood pressure remains controversial, a reasonable approach is to target a systolic pressure of less than 100 mm Hg with MAP between 50 to 60 mm Hg.
As a component of intraoperative management, the effect of anesthetic drugs on the body’s response to hemorrhage is an important difference between deliberate hypotension occurring in the elective operative setting and hemorrhagic shock occurring in the ED. Trauma patients who are hypertensive receive a minimum of anesthetics, even for induction of anesthesia, because of the obvious effect of these drugs on arterial blood pressure. A hypotensive trauma patient is thus in a state of profound vasoconstriction, as opposed to a patient undergoing elective intraoperative hypotension who is vasodilated by general anesthesia before any blood loss. Table 66.4 summarizes the physiologic contrasts between these two states. It should be noted that blood loss without shock does not produce systemic complications such as ARDS in experimental models. Based on this physiology, the recommended goals for early resuscitation are expressed in Box 66.6 , and an algorithm for management is presented in Figure 66.2 . The emphasis in this situation must be on rapid diagnosis and control of ongoing hemorrhage; vascular volume should be restored and anesthesia provided together by shifting the patient from a vasoconstricted to a vasodilated state while facilitating hemostasis by maintenance of a decreased arterial blood pressure.
Aspect | Elective | Trauma |
---|---|---|
Intravascular volume | Euvolemic | Hypovolemic |
Temperature | Normal | Likely hypothermic |
Capillary beds | Dilated | Constricted |
Level of general anesthesia | Deep | Usually light |
Preexisting mental status | Normal | May be impaired |
Coexisting injuries | None | May be significant |
Comorbid conditions | Known and managed | Unknown |
Maintain systolic blood pressure of 80-100 mm Hg
Maintain hematocrit of 25%-30%
Maintain prothrombin time and partial thromboplastin time in normal ranges
Maintain platelet count at greater than 50,000 per high-power field
Maintain normal serum ionized calcium
Maintain core temperature higher than 35°C
Maintain function of pulse oximeter
Prevent increase in serum lactate
Prevent acidosis from worsening
Achieve adequate anesthesia and analgesia
Clinical trials of permissive hypotensive resuscitation have avoided the application of this technique to populations perceived to be at greater risk for ischemic complications, including patients with known ischemic coronary disease, older patients, and those with injuries to the brain or spinal cord. The prohibition against hypotension in patients with TBI is especially well established because of the observed disparity in outcome between TBI patients who experience hypotension and those who do not. Older trauma patients suffer worse outcomes from similar injuries than younger patients, presumably because of their reduced physiologic reserve. Clinical care of these patients is focused on avoidance of ischemic stress and rapid correction of hypovolemia. It may well develop, however, that permissive hypotensive management to enable rapid control of hemorrhage is equally beneficial in vulnerable populations. No clinical trials to date have been conducted on this subject, but a laboratory study did find a benefit of permissive hypotension in animals with both TBI and hemorrhagic shock. Absent convincing evidence in humans, permissive hypotension in older patients or patients with brain injury should generally be avoided.
Hemostatic Resuscitation
As discussed earlier, management of the early coagulopathy associated with trauma must be incorporated into the overall phase 1, Uncontrolled Hemorrhage DCR strategy—often referred to as a hemostatic resuscitation. Little utility is found in targeting endpoints of resuscitation in the face of ongoing hemorrhage. Life-threatening coagulopathy is one of the most serious complications of patients in profound shock from massive hemorrhage and is generally predictable at an early stage.
Because of evidence that severely injured trauma patients are likely to develop an early and aggressive endogenous coagulopathy separate from later loss and dilution of clotting factors compounded from hypothermia and acidosis, the practice of hemostatic resuscitation has become commonplace in the most severely injured patients with shock and ongoing hemorrhage. This entails the early and aggressive use of hemostatic products combined with red blood cells (RBCs) as the primary resuscitation fluids to avoid rapid deterioration into the “bloody vicious cycle” and the classic lethal triad of hypothermia, acidosis, and coagulopathy. Two very distinct paradigms of hemostatic resuscitation have emerged: (1) the DCR model, which uses preemptive administration of empiric ratios of blood and hemostatic products to approximate whole blood, often according to an established institutional massive transfusion protocol ( Fig. 66.8 ) ; and (2) goal-directed hemostatic resuscitation approaches (also often protocol based), which generally use point-of-care viscoelastic monitoring combined with the prompt administration of hemostatic concentrates. The DCR model is typically employed during phase 1, Uncontrolled Hemorrhage, with a transition to more goal-directed hemostatic resuscitation during phase 2, Controlled Hemorrhage.

In addition to the use of permissive hypotension and limited crystalloid usage, DCR relies on the administration of empiric ratios of blood and hemostatic products to restore blood volume. In a retrospective review of combat casualties, Borgman and colleagues found a mortality rate of 65% in patients receiving less than 1 unit plasma for every 4 units RBCs, but only 20% in those with a ratio of 1:2 or above. Evidence exists of a survivor bias because patients bleeding more rapidly were likely to die after receiving RBCs but before plasma could reach the bedside. Although the issue of a survivor bias does exist, this approach has been substantiated in published reviews. Currently a ratio of 1:1:1 is most commonly adopted, although some experts believe that the amount of FFP can be reduced in most cases. In the only major, randomized trial comparing a 1:1:1 ratio with a 1:1:2 ratio of plasma, platelets, and RBCs, respectively, the transfusion of less plasma and platelets was not associated with a difference in mortality although the 1:1:1 group achieved hemostasis more rapidly and had fewer deaths due to exsanguination by 24 hours.
In addition to the hypocoagulability associated with ATC, fibrinolysis is especially deleterious in severely injured trauma patients and carries an associated mortality well upward of 50%. Many patients with primary fibrinolysis from severe hemorrhagic shock may never survive to reach the ICU. The Clinical Randomisation of an Antifibrolytic in Significant Haemorrhage 2 trial is the only class I evidence showing a 30-day survival benefit for a resuscitative therapy including tranexamic acid (TXA). Subgroup analysis showed that the benefit was greatest when therapy was instituted within 1 hour of admission. A subsequent analysis, however, showed that mortality increased when therapy was instituted after 3 hours, suggesting that the risks of therapy outweighed the benefits in patients who survived beyond that timeframe. Many resuscitative protocols for massive hemorrhage now include the early administration of TXA based on this and subsequent studies.
Other potential drugs that may play a role in hemostatic resuscitation include recombinant activated human coagulation factor VII (rFVIIa), prothrombin complex concentrates (PCCs), and fibrinogen concentrates. rFVIIa is licensed for the treatment of patients with hemophilia with active or anticipated hemorrhage and known antibodies to factor VIII. The observation of rapid hemostasis in this population led to the anecdotal use of rFVIIa in other congenital and acquired coagulopathies, including the dilutional coagulopathy of traumatic hemorrhage. Factor VIIa in pharmacologic doses works by triggering a burst of thrombin on the surface of platelets activated by exposed tissue factor, which produces rapid clot formation. Because tissue factor is required, coagulation is limited to the site of vascular injury, and inappropriate clotting of uninjured organs or vessels, though an acknowledged risk, occurs at only low frequency. One small placebo-controlled trial of rFVIIa in hemorrhaging trauma patients has demonstrated decreased blood loss, decreased transfusion requirements, and improved outcome, although a large, randomized trial failed to show a mortality benefit. In combination with an increased risk for thromboembolic adverse events, this lack of clear efficacy has contributed to decreased utilization of rFVIIa as an empiric therapy in the management of the bleeding trauma patient.
Experience with PCCs in clinical practice is limited. PCC has been used for many years for the treatment of congenital coagulation disorders and is recommended for reversing oral anticoagulation, particularly in the setting of traumatic intracranial hemorrhage. PCCs contain coagulation factors II, VII, IX, and X. Differences exist among products in the concentrations of these factors and other constituents, including heparin, protein C, and protein S, so results obtained with one product may not be obtained with a different formulation. Fibrinogen concentrates also may have a role in a hemostatic resuscitation for the patient with a coagulopathy with low levels of fibrinogen.
Phase 2, Controlled Hemorrhage
Once major aspects of bleeding have been controlled, a more individualized, tailored approach to resuscitation can generally be employed by the anesthesia team. In phase 2, there should be increased utilization of point-of-care testing such as arterial blood gas and other laboratory test results, viscoelastic monitoring parameters, and physiologic data to drive decision-making. Assessment of oxygen delivery and adequacy of perfusion can start to incorporate some targeted resuscitation measures such as lactate and base deficit. Use of other monitoring modalities such as transesophageal echocardiography (TEE) or transthoracic echocardiography (TTE) may be useful in quantifying and guiding the need for further intravascular volume replacement.
While surgical bleeding may be corrected at this time, the depth and duration of shock in combination with multisystem trauma may still contribute to coagulopathy. This will require ongoing assessment such as serial evaluations with viscoelastic coagulation monitoring. Transition from empiric replacement of FFP and platelets toward a more tailored approach can be accomplished using an algorithm ( Fig. 66.9 ) to guide specific product selection and limit exposure to unnecessary blood products.

Courtesy of San Francisco General Hospital and Trauma Center.
(From Steurer M, Chang T, Lancman B. Anesthesia for trauma. In: Pardo M, Miller RD, eds. Basics of Anesthesia . 7th ed. Philadelphia: Elsevier; 2018:724 [ Chapter 42 ].
Additionally, during this time, attention to other physiologic considerations can proceed. For example, hypothermia is commonly present on initial presentation. During phase 1, all attempts should be made to initiate active fluid and surface warming although it is difficult to fully correct during a massive resuscitation. During phase 2, additional measures can be instituted as the focus shifts from control of hemorrhage to stabilization of all physiologic processes.
Phase 3, Restoration of Physiology
Box 66.7 summarizes endpoints for late resuscitation, and Fig. 66.10 presents an algorithm for management. Intravenous fluid administration is an integral, mandatory component. The adequacy of resuscitation should not be judged by the presence of normal vital signs, but by restoration of organ and tissue perfusion. The role of the anesthesiologist-intensivist is to recognize the presence of ongoing shock after traumatic hemorrhage and to resuscitate the patient with the appropriate type and amount of fluids intravenously at the appropriate time.
Maintain systolic blood pressure higher than 110 mm Hg
Maintain hematocrit above individual transfusion threshold
Normalize coagulation status
Normalize electrolyte balance
Normalize body temperature
Restore normal urine output
Maximize cardiac output by invasive or noninvasive measurement
Reverse systemic acidosis
Document decrease in lactate to normal range

Late resuscitation begins once bleeding is definitively controlled by surgery, angiography, or the passage of time. The goal at this time is to restore normal perfusion to all organ systems while continuing to support vital functions. Hypoperfusion caused by hemorrhagic shock triggers a predictable cascade of biochemical events that will cause physiologic derangements persisting long after adequate blood flow is restored. The extent of hypoperfusion—the depth and duration of shock—dictates the magnitude of subsequent organ system failure. Unfortunately, traditional vital sign markers such as arterial blood pressure, heart rate, and urine output are insensitive to the adequacy of resuscitation. Occult hypoperfusion syndrome is common in postoperative trauma patients, particularly young ones. This syndrome is characterized by a normal blood pressure maintained by systemic vasoconstriction, decreased intravascular volume and cardiac output, and organ system ischemia. The patient will be at frequent risk for MOD if the hypoperfusion is not promptly corrected.
The search for the optimal endpoints of resuscitation has led to several different hemodynamic, acid-base, and regional perfusion targets. Table 66.5 summarizes modalities that are available to gauge the adequacy of resuscitation, along with the shortcomings of each technique. Although the flow of blood to tissue beds is a determinant of tissue perfusion, pressure should also be an important consideration. The left ventricular stroke work index is a variable that accounts for both flow and pressure. Furthermore, left ventricular power output has been used to quantify left ventricular performance. These indices were compared with purely flow-derived hemodynamic and oxygen transport variables as markers of perfusion and outcome in critically injured patients during resuscitation. A consecutive series of 111 patients were monitored with a volumetric pulmonary artery catheter during the first 48 hours of resuscitation. The ability to clear lactate in less than 24 hours and survival were studied. Survivors exhibited significantly higher stroke work and left ventricular power output than did nonsurvivors. In addition to heart rate, these were the only variables that were significantly related to lactate clearance and survival. The higher stroke work and left ventricular power output in survivors were related to better ventricular-arterial coupling and therefore more efficient cardiac function.
Technique | Shortcomings |
---|---|
Vital signs | Will not indicate occult hypoperfusion |
Urine output | May be confounded by intoxication, diuretic therapy, circadian variation, or renal injury |
Systemic acid-base status | Confounded by respiratory status |
Lactate clearance | Requires time to obtain laboratory result |
Cardiac output | Requires placement of a pulmonary artery catheter, or use of noninvasive technology |
Mixed-venous oxygenation | Difficult to obtain, but a very accurate marker |
Gastric tonometry | Requires time to equilibrate, subject to artifact |
Stroke volume variation | Requires an arterial line; may not be accurate in severe shock |
Tissue oxygenation | Emerging technology, appears beneficial |
Monitoring resuscitation with invasive monitors is gradually changing to noninvasive approaches that assess the return of adequate metabolism, respiration, and oxygen transport in peripheral tissue beds. One such technique is tissue oxygen monitoring (skin, subcutaneous tissue, or skeletal muscle). Skeletal muscle blood flow decreases early in the course of shock and is restored later during resuscitation, thus making the skeletal partial pressure of oxygen a sensitive indicator of decreased flow. Stroke volume variation, the change in arterial pressure driven by the respiratory cycle, is emerging as another less invasive measure of fluid volume status; increased variation in arterial pressure during positive-pressure ventilation is a reliable predictor of decreased intravascular volume. Inadequate tissue perfusion, as indicated by these specific monitors or by the traditional systemic markers of serum lactate, base deficit, and decreased pH, must be treated promptly once ongoing hemorrhage is controlled. The rate at which lactate returns to normal after shock is strongly correlated with outcome; failure to normalize within 24 hours is associated with a greater risk for MOD and eventual death.
Resuscitation Fluids
Isotonic crystalloids (normal saline, lactated Ringer solution, Plasma-Lyte A) are the initial resuscitative fluids administered to any trauma patient (see also Chapter 47 ). They have the advantage of being inexpensive, readily available, nonallergenic, noninfectious, and efficacious in restoring total body fluid. They are easy to store and administer, they mix well with infused medications, and they can be rapidly warmed to body temperature. Disadvantages of crystalloids include their lack of oxygen-carrying capacity, their lack of coagulation capability, and their limited intravascular half-life. Of note, data have implicated specific crystalloid solutions as immunosuppressants and triggers of cellular apoptosis. Unlike necrosis, apoptosis is highly regulated and involves gene modulation and complex pathways of signal transduction. It seems clear that apoptosis is an important element of reperfusion injury. In a rat model of controlled hemorrhage, animals receiving lactated Ringer solution showed an immediate increase in apoptosis in the liver and small intestine after resuscitation. Neither whole blood nor hypertonic saline increased the amount of apoptosis.
Hypertonic saline solutions, with or without the addition of polymerized dextran (HS or HSD), have been extensively studied in resuscitation from hemorrhagic shock. In theory, HS will draw fluid into the vascular space from the interstitium and thereby reverse some of the nonhemorrhagic fluid loss caused by shock and ischemia. A given amount of HS will thus have an enhanced ability to restore intravascular volume in contrast to an equivalent volume of an isotonic solution. This has made HS a popular choice for fluid resuscitation under austere conditions. HSD is licensed for prehospital use in some European countries and is used for resuscitation by units of the U.S. military. Multiple studies of otherwise lethal hemorrhage in animals have demonstrated improved survival after resuscitation with HSD versus either normal saline solution or the components of HSD alone. Studies of the efficacy of HSD in trauma patients have been inconclusive ; the most obvious benefit occurred in a subset of polytraumatized patients with both hemorrhage and TBI, in whom improved neurologic status was demonstrated in those who received HSD as a resuscitation fluid. Indeed, HS is commonly used as an osmotic agent in the management of TBI with increased ICP.
Colloids, including starch solutions and albumin, have been advocated for rapid plasma intravascular volume expansion. Like crystalloids, colloids are readily available, easily stored and administered, and relatively inexpensive. As with hypertonic solutions, colloids will increase intravascular volume by drawing free water back into the vascular space. When intravenous access is limited, colloid resuscitation will restore intravascular volume more rapidly than crystalloid infusion will and at a lower volume of administered fluid. Colloids do not transport oxygen or facilitate clotting; their dilutional effect will be similar to that of crystalloids. Systematic reviews continue to show no benefit of colloids over crystalloids in the setting of trauma resuscitation, although this topic continues to generate significant controversy and would benefit from several well-conducted, randomized trials. Recent concerns, however, have been expressed about specific colloids such as 6% hetastarch and an adverse effect on renal function and mortality (see also Chapter 49 ).
Many of the risks of aggressive intravascular fluid administration just summarized are related to dilution of the circulating blood volume. Recognition of this fact and continued improvement in the safety of donated blood led to increased use of blood products in the management of early hemorrhagic shock (see also Chapter 49 ). The risk for systemic ischemia is decreased by the maintenance of an adequate hematocrit, and the potential for dilutional coagulopathy can be decreased by the early administration of plasma. The composition of resuscitation fluids may be as important as the rate and timing of administration. A 4-year retrospective review of a cohort of critically injured patients who underwent emergency surgery examined the outcomes of short-term care based on the number of units of blood transfused. One hundred forty-one patients received massive blood transfusions (≥20 units of RBCs) during preoperative and intraoperative resuscitation. The number of blood units did not differ between survivors (30%) and nonsurvivors (70%). Eleven variables were significantly different: aortic clamping for control of arterial blood pressure, use of inotropic drugs, time with a systolic blood pressure higher than 90 mm Hg, time in the OR, temperature less than 34°C, urine output, pH lower than 7.0, PaO 2 /FiO 2 ratio less than 150, PaCO 2 more than 50 mm Hg, K + more than 6 mM/L, and calcium less than 2 mM/L. Of these, the presence of the first three variables in the face of transfusion of more than 30 units of packed RBCs was invariably fatal. Total blood loss and the amount of transfused blood were less critical than the depth and duration of shock. These concerns led to the concept of damage control surgery discussed earlier, which emphasizes rapid control of active hemorrhage.
RBCs are the mainstay of treatment of hemorrhagic shock. With an average hematocrit of 50% to 60%, a unit of RBCs will predictably restore oxygen-carrying capacity and expand intravascular volume as well as any colloid solution. RBCs of blood type A, B, or AB carry major incompatibility antigens that may precipitate a lethal transfusion reaction if given to a patient with the opposite blood type. Because RBCs also carry dozens of minor antigens that can cause reactions in susceptible patients, crossmatching is desirable when time allows (typically 1 hour from the time a sample reaches the blood bank until the RBCs reach the patient). Type-specific blood requires less time for delivery from the blood bank (usually ∼30 minutes) and may be an appropriate alternative in some situations. Type O blood—the universal donor type—can be given to patients of any blood type with little risk for a major reaction. This is the preferred approach for patients who arrive at the ED in hemorrhagic shock. If O-positive blood is given to a Rhesus-negative woman who survives, prophylactic administration of anti-Rh 0 antibody is indicated.
Risks of RBC administration include transfusion reaction, transmission of infectious agents, and hypothermia (see also Chapter 49 for details). For example, RBCs are stored at 4°C and will decrease the patient’s temperature rapidly if not infused through a warming system at the time of administration.
Plasma requires blood typing but not crossmatching; delay in availability of plasma is caused by the need to thaw frozen units before they can be administered. Busy trauma hospitals will often maintain a supply of prethawed plasma (thawed fresh plasma as opposed to FFP) that can be issued quickly in response to an emergency need; in smaller hospitals it is important to request plasma early in resuscitation if it is likely to be needed. Very busy centers are experimenting with keeping 2 to 4 units of prethawed type AB (universal donor) plasma available in the trauma resuscitation unit. Units are kept ready in this way for 2 days at a time; if not used on an emergency basis, the units are returned to the blood bank and released to the next patient needing plasma. Whether this approach improves outcomes has not yet been studied.
Platelet transfusion should normally be reserved for patients with clinical coagulopathy with a documented low serum level (>50,000 per high-power field). When the patient is in shock, however, and blood loss is likely to be substantial, platelets should be empirically administered in proportion to RBCs and plasma (1:1:1), as discussed earlier for DCR. Transfused platelets have a very short serum half-life and should be administered only to patients with active coagulopathic bleeding. Platelets should not be administered through filters, warmers, or rapid infusion systems because they will bond to the inner surfaces of these devices, thereby reducing the quantity of platelets actually reaching the circulation.
Rapid transfusion of banked blood carries the risk for inducing citrate intoxication in the recipient. Harvested blood units are treated with citrate to bind free Ca 2+ and thus inhibit the clotting cascade. Consecutive administration of multiple units of banked blood leads to a correspondingly large dose of citrate, which may overwhelm the body’s ability to mobilize free Ca 2+ and have a profound negative inotropic effect on the heart. Unrecognized hypocalcemia is a cause of hypotension in patients after massive transfusion and persists despite an adequate volume of resuscitation. Ionized Ca 2+ levels should be measured at regular intervals in a hemorrhaging patient, and Ca 2+ should be administered as needed (in a separate intravenous line from transfusion products) to maintain serum levels greater than 1.0 mmol/L.
Resuscitation Equipment
Intravascular fluid resuscitation of any kind is impossible in the absence of intravenous access. Immediate placement of at least two large-bore catheters (16 gauge or larger) is recommended during the primary assessment of any trauma patient. Practitioners should have a low threshold for placement of a large-caliber central line in any patient in whom antecubital or other peripheral placement attempts have been unsuccessful. Potential sites for central line placement include the internal jugular, subclavian, and femoral veins, each of which has its own benefits and potential risks. The internal jugular approach, though familiar to most anesthesiologists, will require removal of the cervical collar and manipulation of the patient’s neck and is not recommended in the acute setting unless other options have been exhausted. The femoral vein is easily and rapidly accessed and is an appropriate choice in patients without apparent pelvic or thigh trauma who require urgent drug or fluid administration. Caution should be used in patients with penetrating trauma to the abdomen because fluids infused via the femoral vein may contribute to hemorrhage from an injury to the inferior vena cava or iliac vein; these patients should have intravenous access placed above the diaphragm if possible. Femoral vein catheterization carries a high risk for the formation of deep venous thrombosis, thus limiting use of this approach to the acute setting. Femoral lines should be removed as soon as possible after the patient’s condition stabilizes. The subclavian vein is the most common site for early and ongoing central access in a trauma patient because the subclavian region is easily visible and seldom directly traumatized. This approach carries the highest risk for the development of pneumothorax, although many patients will already have indications for tube thoracostomy in one or both chest cavities; when possible, this is the preferred side for placement of a subclavian line. Placement of an arterial line facilitates frequent laboratory analysis and allows close monitoring of blood pressure; this should be undertaken as soon as possible but should not impede other diagnostic or therapeutic maneuvers.
The anesthesiologist should work to maintain thermal equilibrium in any trauma patient. Although deliberate hypothermia has been suggested as a management strategy for both hemorrhagic shock and TBI, insufficient evidence exists to support this approach. Hypothermia will potentiate dilutional coagulopathy and systemic acidosis while shivering and vasoconstriction in response to cold will demand an additional metabolic effort that may predispose the patient to myocardial ischemia. Hypothermia also greatly increases the subsequent risk for sepsis. Because many trauma patients arrive at the ED cold from exposure to the elements, early active warming measures are required. All IV fluids should be prewarmed or infused through a warming device. The patient should be kept covered with warmed blankets whenever possible, and the environment should be kept warm enough to make the patient comfortable. If hypothermia has already developed, the use of forced hot air warming is strongly indicated to restore normothermia. Even though all these measures are routine and obvious in the OR, the anesthesiologist can perform a valuable service by ensuring that they are available and applied in the ED, CT scanner, and angiography suite as well.
Commercial rapid-infusion devices are of great benefit in trauma care, particularly in the presence of hemorrhagic shock. These machines offer benefits when large quantities of fluid resuscitation are likely ( Box 66.8 ). Early experience with these devices demonstrated higher patient temperature and reduced acidosis at the conclusion of the initial surgery, although rapid infusers may contribute to over infusion of fluids, inappropriately increased arterial blood pressure, and contribute to rebleeding. Application of the principles described earlier for permissive hypotension can prevent this complication; basal fluid administration should be kept low (200-500 mL/h), with fluid boluses given in response to measured systolic blood pressure less than 80 to 90 mm Hg. In practice, fluid boluses are given alternately with anesthetics, with the goal of reaching a normal depth of anesthesia without significantly increasing systolic arterial blood pressure until active bleeding is controlled.
An active mechanical pump enables fluid administration rates up to 1500 mL/min
Compatible with crystalloid, colloid, packed red blood cells, washed salvaged blood, and plasma ( not platelets)
Reservoir allows for mixing of products in preparation for rapid blood loss
Fluids infused at a controlled temperature (38°C-40°C)
Able to pump simultaneously through multiple intravenous lines
Fail-safe detection systems to prevent infusion of air
Accurate recording of fluid volume administered
Portable enough to travel with patient between units
Trauma to the Central Nervous System
CNS trauma accounts for almost half of all trauma deaths after trauma center admission and as indicated by postmortem examinations in population-based analyses (see also Chapter 57 ). In the United States, 2.8 million TBI-related ED visits, hospitalizations, and deaths occurred in 2013. Although not as prevalent, acute traumatic SCI also remains a significant problem. As with hemorrhagic shock, CNS trauma consists of both the primary injury, in which tissue is disrupted by mechanical force, and a secondary response, in which the body’s reaction to the injury plays an important role. Mitigation of secondary CNS injury depends on prompt diagnosis and early, goal-directed therapy. Although primary CNS injuries cannot be minimized other than with preventive strategies, secondary injury accounts for much of the death and disability that follows trauma. The initial management of these patients can significantly affect outcome. Attention to the ABCDE approach is paramount to successful resuscitation, and the trauma anesthesiologist should be intimately involved in this process.
Trauma produces shear forces that result in primary damage to neuronal cell bodies and axons, and to the vasculature. The pathophysiologic processes of secondary injury include metabolic failure, oxidative stress, and a cascade of biochemical and molecular events leading to both delayed necrotic and apoptotic cell death. Secondary injury is often exacerbated by tissue hypoxia or ischemia and by inflammatory responses, and outcomes from TBI are influenced by a large number of interacting factors. Individual drugs such as free radical scavengers, antiinflammatory agents, and ion channel blockers have been effective in animals but have had little impact or yielded disappointing results in human trials. Long-term outcome cannot be predicted from TBI; thus full resuscitative efforts should be initiated and continued, even in the most severely injured. In a recent review of the Trauma Registry of the German Society for Trauma Patients looking at over 50,000 patients, outcome prediction was most accurate using the GCS motor score and pupil reactivity. In patients with bilateral fixed and dilated pupils, a good outcome was still seen in 8% of patients.
Patients with mild TBI (GCS score of 13-15) who maintain a stable GCS score for 24 hours after injury are very unlikely to deteriorate further, although they are at risk for several postconcussive effects, including headache, memory loss, emotional lability (including aggressive behavior and violence), and sleep disturbance. Moderate TBI (GCS score of 9-12) may be accompanied by intracranial lesions that require surgical evacuation, and early CT is strongly indicated. Early tracheal intubation, mechanical ventilation, and close observation may be required in the management of patients with moderate TBI because of combative or agitated behavior and the potentially catastrophic consequences of respiratory depression or pulmonary aspiration occurring during diagnostic evaluation. Extubation of the trachea can be undertaken if the patient is hemodynamically stable and appropriately responsive after the diagnostic workup. Treatment of secondary brain injury is accomplished by early correction and subsequent avoidance of hypoxia, prompt intravascular fluid resuscitation, and management of associated injuries. The timing of indicated noncranial surgery in these patients with moderate injury not requiring decompressive craniectomy is highly controversial because early surgery can increase the episodes of hypoxia and hypotension.
Neurologic monitoring of patients with moderate TBI consists of serial assessment of consciousness and motor and sensory function (see also Chapter 39 ). Deterioration of the GCS score is an indication for urgent CT to establish the need for craniotomy or invasive monitoring of ICP. If frequent neurologic monitoring is not possible because of general anesthesia continuing longer than 2 hours, aggressive analgesia, or prophylaxis against delirium tremens, invasive ICP monitoring may be indicated. Although mortality from moderate TBI is infrequent, many patients will have significant long-term morbidity.
Severe TBI is classified as a GCS score of 8 or less at the time of admission and carries a significant risk for mortality. Patients with severe TBI have mortality three times that of patients with other types of traumatic injury. Early, rapid management focused on restoration of systemic homeostasis and perfusion-directed care of the injured brain will produce the best possible outcomes in this difficult population. Guidelines for all aspects of the management of patients with severe TBI have been published by the American Association of Neurological Surgeons and Brain Trauma Foundation, now in the fourth edition. The clinical pathway in place at the R Adams Cowley Shock Trauma Center in Baltimore appears in Fig. 66.11 .

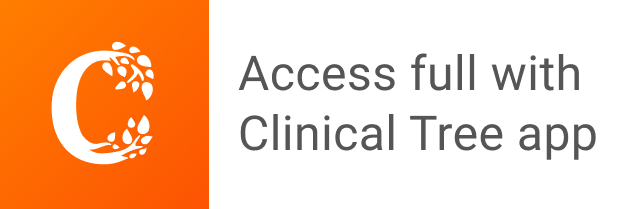