Key Points
- ▪
Organ system maturation, from birth through adolescence, affects physiologic function and therefore anesthetic and surgical management and outcome.
- ▪
The understanding of congenital heart disease (CHD) and consequent anesthetic management is based on the pathophysiologic determinants of four categories of defects: shunts, mixing lesions, stenotic lesions, and regurgitant lesions.
- ▪
The chronic sequelae of CHD (repaired, palliated, or unrepaired) affect anesthetic management: ventricular failure, residual hemodynamic effects (e.g., valve stenosis), arrhythmias, and pulmonary blood flow changes (e.g., pulmonary artery hypertension).
- ▪
Preoperative assessment of cardiac status and planning are the keys to a successful anesthesia outcome.
- ▪
Intraoperative transesophageal echocardiography and central nervous system (CNS) monitoring enhance surgical outcome and reduce morbidity.
- ▪
The induction technique needs to consider degree of cardiac dysfunction, cardiac defects, level of sedation achieved with premedication, and the presence of an indwelling venous catheter. Other factors to be considered include pulmonary hypertension, electrical rhythm disorders, and other comorbidities.
- ▪
The maintenance of anesthesia depends on the age and condition of the patient, the nature of the surgical procedure, the duration of cardiopulmonary bypass (CPB), and the need for postoperative ventilation. It is usually desirable to return the patient to an arousable, sedated state with spontaneous ventilation at the end of the procedure.
- ▪
The physiologic effects of CPB on neonates, infants, and children are significantly different from the effects on adults. During CPB, pediatric patients are exposed to biologic extremes not seen in adults, including deep hypothermia (18°C), increased hemodilution, low perfusion pressures, and wide variation in pump flow rates.
- ▪
After repair of complex congenital heart defects, separating patients from CPB may be difficult. The causes may be an inadequate surgical result, pulmonary artery hypertension, low systemic vascular resistance, low hemoglobin, and right or left ventricular dysfunction
- ▪
The use of modified ultrafiltration (MUF) reverses the deleterious effects of hemodilution and the inflammatory response associated with CPB in children. Furthermore, with MUF perioperative blood loss and blood use are significantly reduced, left ventricular function and systolic blood pressure are improved, oxygen delivery is increased, and pulmonary compliance and brain function are also better.
- ▪
Neonates, infants, and children undergoing cardiac surgery with CPB have a more frequent rate of postoperative bleeding than that seen in older patients. This is due to an inflammatory response that a disproportionate exposure to the nonendothelialized extracorporeal circuit produces, the type of surgery performed in neonates and infants that involves more extensive reconstruction and suture lines, the frequent utilization of deep hypothermia or circulatory arrest, a more immature coagulation system in neonates, and an increased bleeding tendency in patients with cyanotic heart disease.
- ▪
The management of the postoperative patient warrants an understanding of both normal and abnormal convalescence after anesthesia and cardiac surgery, as it is characterized by continuous physiologic change.
- ▪
The care of adults with CHD is a new and expanding field of medicine that requires the skilled management of an experienced multidisciplinary team.
- ▪
Additional anesthetic considerations exist in patients with CHD who undergo transplantation, closed heart operations without CPB, cardiac interventional procedures, and noncardiac surgery.
Acknowledgment
The editors, publisher, and returning author Dr. William J. Greeley, would like to thank Dr. Aruna T. Nathan and the late Dr. Chad C. Cripe for their contributions to this chapter in the prior edition of this work. It has served as the foundation for the current chapter.
Cardiac surgery is an established and effective treatment for children with congenital heart defects. Early successes in surgical treatment led to a new therapeutic era in the management of congenital heart disease (CHD) and fostered the development of the subspecialties for pediatric cardiology and cardiac surgery and their collaboration. Through this cooperative effort, tremendous progress in medical diagnosis and surgical treatment has been achieved. In turn, these accomplishments gave rise to the development of pediatric cardiac anesthesiologists, individuals who understand the pathophysiology of congenital heart malformations, the diagnostic and surgical procedures used to treat heart disease, and the principles of pediatric and cardiac anesthesia and intensive care medicine. Pediatric cardiac anesthesia continues to evolve as an exciting and technically demanding subspecialty in which anesthetic management is based on physiologic principles.
Cardiovascular surgery and anesthesia in CHD are often performed under unusual physiologic conditions. Rarely in clinical medicine are patients exposed to such biologic extremes as during surgery for CHD. Commonly, patients are cooled to 18°C, are acutely hemodiluted by more than 50% of their extracellular fluid volume, and undergo periods of total circulatory arrest lasting up to 1 hour. Management of patients under these physiologic extremes is a vital function of the pediatric cardiovascular anesthesiologist. As with other areas of medicine, the application and management of technology preceded a comprehensive understanding of its physiologic effects.
Clearly, the perioperative management of these complex cases requires a group of physicians (surgeon, anesthesiologist, cardiologist, critical care specialist), nurses, and perfusionists to work as a team. Although the quality of the surgical repair, the effects of cardiopulmonary bypass (CPB), and postoperative care are the major determinants of outcome, meticulous anesthetic management is also imperative. Ideally, despite the complexity of the cases and the marked physiologic changes attributed to CPB and the surgical procedures, anesthetic care should never contribute substantially to morbidity or mortality. The challenge is to understand the principles underlying the management of patients with CHD and apply them to clinical anesthesia.
Acknowledgment
The editors, publisher, and returning author Dr. William J. Greeley, would like to thank Dr. Aruna T. Nathan and the late Dr. Chad C. Cripe for their contributions to this chapter in the prior edition of this work. It has served as the foundation for the current chapter.
Cardiac surgery is an established and effective treatment for children with congenital heart defects. Early successes in surgical treatment led to a new therapeutic era in the management of congenital heart disease (CHD) and fostered the development of the subspecialties for pediatric cardiology and cardiac surgery and their collaboration. Through this cooperative effort, tremendous progress in medical diagnosis and surgical treatment has been achieved. In turn, these accomplishments gave rise to the development of pediatric cardiac anesthesiologists, individuals who understand the pathophysiology of congenital heart malformations, the diagnostic and surgical procedures used to treat heart disease, and the principles of pediatric and cardiac anesthesia and intensive care medicine. Pediatric cardiac anesthesia continues to evolve as an exciting and technically demanding subspecialty in which anesthetic management is based on physiologic principles.
Cardiovascular surgery and anesthesia in CHD are often performed under unusual physiologic conditions. Rarely in clinical medicine are patients exposed to such biologic extremes as during surgery for CHD. Commonly, patients are cooled to 18°C, are acutely hemodiluted by more than 50% of their extracellular fluid volume, and undergo periods of total circulatory arrest lasting up to 1 hour. Management of patients under these physiologic extremes is a vital function of the pediatric cardiovascular anesthesiologist. As with other areas of medicine, the application and management of technology preceded a comprehensive understanding of its physiologic effects.
Clearly, the perioperative management of these complex cases requires a group of physicians (surgeon, anesthesiologist, cardiologist, critical care specialist), nurses, and perfusionists to work as a team. Although the quality of the surgical repair, the effects of cardiopulmonary bypass (CPB), and postoperative care are the major determinants of outcome, meticulous anesthetic management is also imperative. Ideally, despite the complexity of the cases and the marked physiologic changes attributed to CPB and the surgical procedures, anesthetic care should never contribute substantially to morbidity or mortality. The challenge is to understand the principles underlying the management of patients with CHD and apply them to clinical anesthesia.
Unique Features of Pediatric Cardiac Anesthesia
Pediatric cardiovascular management is unique, with important differences from adult cardiac surgery ( Box 78.1 ). These differences are attributable to normal organ system maturation in the neonate and young infant, differing pathophysiologic conditions in CHD, the diversity of surgical repairs, and the use of specialized CPB techniques such as deep hypothermia, total circulatory arrest, cerebral regional perfusion, and three-region perfusion techniques.
Patient
Normal organ system development and maturational changes of infancy
Cardiovascular: Blood flow patterns of circulation at birth, myocardial compliance, systemic and pulmonary vasculature, and β-adrenergic receptors
Pulmonary: Respiratory quotient, closing capacity, chest compliance
Central nervous system: Brain growth, cerebral blood flow, autonomic regulation
Renal: Glomerular filtration rate, creatinine clearance
Hepatic: Liver blood flow, microsomal enzyme activity
Disease and growth interrelationship
Effects of systemic disease alter somatic and organ growth
Compensatory ability of developing organs to recover from injury
Immunologic immaturity of the infant
Obligatory miniaturization (i.e., small patient size and body surface area)
Congenital Heart Disease
Diverse anatomic defects and physiologic changes
Altered ventricular remodeling owing to myocardial hypertrophy and ischemia
Chronic sequelae of congenital cardiac disease
Surgical Procedures
Diversity of operations
Frequent intracardiac and right ventricular procedures
Use of deep hypothermia and circulatory arrest during repair
Trend toward repair in early infancy
Evolution of surgical techniques to avoid residua and sequelae
Trend toward wider application of certain operations
Physiologic Considerations and Maturational Features of the Pediatric Patient
The cardiovascular system changes markedly at birth because of a dramatic alteration in blood flow patterns ( Fig. 78.1 ). During fetal life, blood flow returning to the right atrium (RA) bypasses the unventilated fluid-filled lungs. Blood is then preferentially shunted across the patent foramen ovale into the left atrium (LA) or passes from the right ventricle (RV) across the patent ductus arteriosus (PDA) to the systemic circulation. At birth, physiologic closure of the PDA and of the foramen ovale brings about the normal adult circulatory pattern. The presence of certain congenital heart defects or pulmonary disease can disrupt this normal adaptive process, creating a transitional circulation in which right-to-left shunting persists across the foramen ovale or the PDA. Under such circumstances, the continued presence of a transitional circulation can lead to severe hypoxemia, acidosis, and hemodynamic instability, which are poorly tolerated in the neonate. In contrast, when initially treating some forms of CHD, the prolongation of this transitional circulation is actually beneficial, promoting systemic blood flow or pulmonary blood flow (PBF) and postnatal viability. An example of the latter is pulmonary atresia, in which PBF is supplied via the PDA. In the absence of collateral vessels, closure of the PDA eliminates the principal source of PBF, resulting in hypoxemia and death. In such patients, ductal patency can be maintained with the administration of prostaglandin E1. Importantly, the transitional circulation can be manipulated by pharmacologic and ventilatory strategies, thereby promoting hemodynamic stability.

Another unique feature of the normal neonatal and infant cardiovascular system is the reduced myocardial reserve in contrast to that in the healthy adult. The newborn left ventricular function is restricted by a reduced number of α-adrenergic receptors, high resting levels of circulating catecholamines, limited recruitable stroke work, an immature calcium transport system, and decreased ventricular compliance. These factors limit contractile reserve with the result of a left ventricle (LV) with a high level of resting tone. Although the resting performance of the neonatal myocardium may be greater than in adults and older children, sensitivity to β-blockade is greater and only modest increases occur in cardiac performance after administration of the β-agonist drugs dobutamine and isoproterenol. Furthermore, the contractile mass of the heart is effectively reduced, resulting in a ventricle with low compliance. Preload augmentation is effective at low filling pressures (1-7 mm Hg). However, when left-sided filling pressures exceed 7 to 10 mm Hg, further increases in left ventricular stroke volume are minimal. As a consequence, neonates are more dependent on heart rate and, to a lesser extent, on preload, to maintain cardiac output at filling pressures of 7 to 10 mm Hg or greater. Additionally, the calcium transport system in the neonatal myocardium is underdeveloped. The neonatal heart therefore depends more on extracellular calcium levels than the adult myocardium, and normal or even elevated plasma levels of ionized calcium may be necessary to augment or maintain an effective stroke volume. This is in contrast to adult cardiac patients, in whom calcium use during cardiac surgery has fallen into some disfavor, owing to direct concerns over myocardial ischemia and reperfusion injury.
Another unique feature relates to the pulmonary circulation. The pulmonary circulation undergoes significant changes during the first months of life. In the immediate newborn period, the large decrease in pulmonary vascular resistance (PVR) is due to lung expansion and the vasodilatory effects of a higher PaO 2 than existed in utero. Further decline in PVR throughout the next 2 months of life is attributable to regression of the smooth muscle layer in the pulmonary arterioles. A corresponding decrease in pulmonary artery pressure occurs as PVR declines. Acute physiologic stress in the newborn period, such as hypoxemia or acidosis, may increase pulmonary artery pressure and thus PVR. If the resulting right ventricular hypertension causes reduced right ventricular compliance, right-to-left shunting can occur at the foramen ovale. Once PVR exceeds systemic vascular resistance (SVR), right-to-left shunting develops at the PDA. Either phenomenon will worsen the hypoxemia and eventually limit tissue oxygen (O 2 ) delivery. In contrast, left-to-right shunts, such as those present with a ventricular septal defect (VSD), increase PBF which over time produces intimal changes in the pulmonary vasculature, delaying regression of medial muscle hypertrophy and producing persistent elevation of PVR.
Size differences between adult and pediatric cardiac patients require different anesthetic techniques and miniaturization. Anatomically, pediatric patients have smaller upper and lower airways, smaller veins and arteries, and decreased body surface area compared to adult patients; these differences have anesthetic implications. Availability of ultrasound has made placement of arterial catheters more expedient, even in the smallest patients, reducing the need for arterial cutdown. Pulmonary artery catheters are used infrequently because of technical difficulties in positioning the tip in the pulmonary artery and because of the fundamental fact that pulmonary flow bears no obligatory relationship to systemic output in children with either intracardiac or extracardiac communications. Transthoracic catheters for pressure monitoring and delivery of vasoactive substances may be placed from the surgical field instead of percutaneous approach via the neck. Adequacy of surgical repair and function is assessed by transesophageal echocardiography (TEE) with Doppler color flow imaging with miniaturized probes.
Patient size also influences CPB management. The ratio of pump priming volume to patient blood volume is considerably higher in small children than in adults, resulting in a greater degree of hemodilution. Several studies demonstrated a heightened inflammatory response to CPB in children in contrast to in adults, related to the disproportionate exposure of the child’s blood elements to the nonendothelialized surfaces of the pump circuit per body surface area.
In pediatric patients with CHD, the cardiovascular system often represents the sole cause of the medical problem. A special disease and growth interrelationship, unique to growing infants and children, permits developing organs to compensate for and modify existing disease processes. Reparative and recuperative processes in children are greater as a result of this compensatory ability of developing organ systems. Despite adaptation to their cardiovascular pathologic processes CHD pediatric patients do experience detrimental and sometimes permanent effects on somatic growth and on the growth and development of the brain, myocardium, and lung.
The premature infant with CHD deserves special consideration. Premature infants are classified as low birth weight (31-34 weeks, 1-1.5 kg), very low birth weight (26-30 weeks, 600 g-1 kg), and extremely low birth weight (<26 weeks, 400-600 g). Respiratory failure is common and multifactorial. The small airways are prone to obstruction, which results in increased airway resistance and work of breathing with easy fatigability. Lung compliance is reduced because of a deficiency of surfactant, resulting in intrapulmonary shunting and ventilation-perfusion mismatch. Mechanical ventilation prevents alveolar collapse, maintains patency of the airway, and maintains lung volume, preventing hypoxia, but must be used cautiously as premature lungs are susceptible to barotrauma and oxidant injury. Ventilatory strategies for lung protection include reduced peak inspiratory pressures and the lowest inspired oxygen concentration that produces reasonable levels of oxygenation.
Premature infants are also prone to perioperative periods of apnea that may be central in origin or obstructive, either of which can be aggravated by anesthetic drugs. Apnea may also be precipitated by abrupt changes in oxygenation or pulmonary mechanics, brain hemorrhage, and hypothermia. After emergence from anesthesia, sustained apnea may occur and persist for up to 48 hours. Continuous apnea and saturation monitoring with correction of anemia (hematocrit > 30) and intravenous administration of caffeine are therapeutic options. The incidence of postoperative apnea is related to postconceptional and gestational age, the presence of anemia, and the type of surgical procedure.
Cardiac pathophysiology can compound respiratory concerns. The premature heart is a poorly contractile organ with poor diastolic function, and is sensitive to changes in intracellular calcium. Cardiac output depends chiefly on heart rate, with marginal reserve. The premature infant also has a relatively low absolute blood volume and tolerates blood loss poorly. Autoregulation is not well developed, and blood loss compromises cerebral and coronary flow before other manifestations of hypovolemia. However, fluid overload is also not well tolerated. Patency of the ductus arteriosus results in left-to-right shunting and pulmonary overcirculation with heart failure (HF). If uncorrected, this would result in pulmonary hypertension secondary to the development of pulmonary vascular intimal hypertrophy.
Thermoregulation by nonshivering thermogenesis is also poor owing to inadequate stores of brown fat in prematurity. It is critical to maintain normothermia by increasing the operating room temperature, using incubators for transport, warming and humidifying respiratory gases, and warming all intravenous fluids. Glycemic control is difficult, with a tendency to both hypoglycemia and hyperglycemia. Frequent glucose checks are important. Parenteral glucose solutions should be continued in the perioperative period. Premature infants are also prone to retinopathy of prematurity with high inspired O 2 concentrations and intraventricular hemorrhage. Every attempt should be made to avoid hemodynamic perturbances and fluctuation in O 2 saturations. In general, immaturity of organ systems results in both increased drug effect and duration of action, warranting careful titration of drugs.
Premature infants have a twofold increase in cardiovascular malformations compared with term infants. One in six infants with CHD, not including those with PDA or atrial septal defect (ASD), is born prematurely. Some malformations such as tetralogy of Fallot (TOF), pulmonary stenosis, pulmonary atresia with VSD, complete atrioventricular (AV) septal defect, large VSDs in isolation or associated with coarctation, and aortic stenosis are more prevalent in this population. A significant increase in the likelihood of being small for gestational age is seen among infants with TOF, complete AV septal defect, hypoplastic left heart, pulmonary stenosis, or large VSD.
While cardiac catheterization, intervention, and complete surgical repair in the newborn of very low birth weight (<1.5 kg) can be successfully undertaken with low risk, it is notable that given the complexity of premature organ systems and superimposed cardiorespiratory pathophysiology, morbidity and mortality are increased. Low-birth-weight neonates with complex single ventricle disease undergoing surgical palliation have a significant mortality risk. Interventional catheterization in premature infants is associated with a higher risk for complications related to vascular access, arrhythmias, and respiratory compromise. Maintenance of euglycemia and normothermia and attention to fluid and electrolyte balance are important. O 2 delivery is optimized by maintaining age-appropriate blood pressures, adequate intravascular volume, and hematocrit. Any acidosis must be sought and aggressively corrected. Whenever possible, such infants should recover in a specialized pediatric cardiac intensive care unit (ICU).
Congenital Heart Disease
The marked array of anatomic and physiologic conditions seen with CHD distinguishes these processes from acquired adult cardiac disease. The spectrum of intracardiac shunts, valve pathologies, disrupted great artery connections, and the absence of one or more chambers of the heart preclude a uniform anesthetic approach to patients with CHD. Moreover, myocardial changes result from the hemodynamic impact and increased cardiac work incurred by these defects. Functionally, these myocardial changes place the ventricles at great risk for the development of intraoperative ischemia and failure. Therefore, an understanding of the isolated defect, associated myocardial changes, and hemodynamic consequences is fundamental to planning an appropriate anesthetic regimen. Distilling CHD into a finite number of physiologic categories enables the anesthesiologist to construct a strategy that employs the qualitatively predictable impact of drugs, ventilatory management, and fluid administration to optimize cardiovascular performance. Although an isolated heart malformation may be identified, the entire cardiopulmonary system is usually affected.
Physiologic Approach to Congenital Heart Disease
Although the structural variations seen in CHD constitute an encyclopedic list of malformations, anesthetic management is more logically designed to achieve physiologic goals. A general physiologic classification is listed in Table 78.1 . Fortunately, although structurally complex, these defects can be understood within a more limited physiologic spectrum. Identification and classification on the basis of physiology provide an organized framework for the intraoperative anesthetic management and postoperative care of children with complex congenital cardiac defects. In general, congenital heart lesions fit into one of four categories: shunts, mixing lesions, flow obstruction, and regurgitant valves (see Table 78.1 ). Each category imposes at least one of three pathophysiologic states: ventricular volume overload, ventricular pressure overload, or hypoxemia. Ultimately, these pathophysiologic conditions can result in myocardial failure or pulmonary vascular disease. Medical and surgical perioperative management strategies focus on minimizing the pathophysiologic consequences of these lesions.
Physiologic Classification | Pulmonary Blood Flow | Comments |
---|---|---|
Left-to-right shunts | ||
VSD | ↑ | Volume-overloaded ventricle |
ASD | Development of CHF | |
PDA | ||
AV canal | ||
Right-to-left shunts | ||
Tetralogy of Fallot (TOF) | ↓ | Pressure-overloaded ventricle |
Pulmonary atresia/VSD | Cyanotic | |
Eisenmenger complex | Hypoxemia | |
Mixing lesions | ||
Transposition/VSD | Generally↓ but variable <SPAN role=presentation tabIndex=0 id=MathJax-Element-1-Frame class=MathJax style="POSITION: relative" data-mathml='Q˙p/Q˙s’>Q˙p/Q˙sQ˙p/Q˙s Q ˙ p / Q ˙ s | Variable pressure versus volume loaded |
Tricuspid atresia | Usually cyanotic | |
Anomalous venous return | ||
Univentricular heart | ||
Obstructive lesions | ||
Interrupted aortic arch | Ventricular dysfunction | |
Critical aortic stenosis | Pressure-overloaded ventricle | |
Critical pulmonic stenosis | Ductal dependence | |
Hypoplastic left heart syndrome | ||
Coarctation of the aorta | ||
Mitral stenosis | ||
Regurgitant lesions | ||
Ebstein anomaly | Volume-overloaded ventricle | |
Other secondary causes | Development of CHF |
Q ˙ p
, pulmonary blood flow; <SPAN role=presentation tabIndex=0 id=MathJax-Element-3-Frame class=MathJax style="POSITION: relative" data-mathml='Q˙s’>?˙?Q˙s
Q ˙ s
, systemic blood flow; VSD, ventricular septal defect.
Shunt Lesions
Shunts are intracardiac connections between chambers (e.g., ASD or VSD) or extracardiac connections between a systemic and pulmonary artery (e.g., PDA). The direction of blood flow through the shunt depends on the relative resistances on either side of the shunt and on the size of the shunt orifice. The direction and magnitude of shunt at the atrial level are additionally governed by the relative differences in ventricular compliance and respective AV valve function. With a nonrestrictive VSD or PDA that does not impede blood in either direction, the main determinant of the direction of blood flow is relative resistance between the pulmonary and systemic vascular beds. The effect that a shunt lesion has on the cardiovascular system depends on both the size of the shunt and its direction.
Left-to-right shunts occur when the PVR is lower than the SVR, so that blood flow is preferentially directed toward the lungs, resulting in increased PBF. In patients with large left-to-right shunts and low PVR, this increase in PBF can be substantial and result in three pathophysiologic problems: (1) congestion of the pulmonary circulation; (2) intravascular volume overload with resulting increased cardiac work for the LV; and (3) excessive PBF, resulting in progressive elevation in PVR. Volume overload causes ventricular dilation that places the heart at a mechanical and physiologic disadvantage, resulting in reduced diastolic compliance. Diastolic changes lead to engorgement of the respective venous beds, which produces the signs and symptoms of clinical congestive heart failure (CHF) early in the natural history of volume-overload condition. The demand for increased cardiac output placed on the LV is limited in the infant by virtue of its immature structure, so that large left-to-right shunts may outstrip the capacity of the left side of the heart to maintain adequate systemic perfusion.
Surgical closure of a hemodynamically significant VSD usually provides immediate benefit by dramatically lowering left ventricular volume output demands. Occasionally, the sudden increase in wall stress imposed on a dilated ventricle that must now pump solely against SVR can produce worsening ventricular failure during the early postoperative period after eliminating the low-resistance “pop off” into the pulmonary circulation. If the left-to-right shunt is not repaired, prolonged exposure to increased PBF results in progressive elevations in PVR. Fixed changes in pulmonary arterioles may occur, leading to pulmonary vascular obstructive disease, which may become irreversible. Table 78.1 lists common left-to-right shunt lesions.
Right-to-left shunts occur when pulmonary vascular or right ventricular outflow tract resistance exceeds SVR, thereby reducing PBF. The systemic circulation receives an admixture of deoxygenated blood via the shunt. This manifests clinically as cyanosis and hypoxemia. Pure right-to-left shunting resulting from increased PVR is seen in the Eisenmenger complex and persistent pulmonary hypertension of the newborn with shunt at both the atrial and ductal levels. More commonly, PVR is low, and the right-to-left shunt is produced by a more complex lesion with obstruction of pulmonary outflow proximal to the pulmonary vasculature. A classic example of right-to-left shunt is TOF, where shunting occurs through the VSD because of pulmonary outflow obstruction. Systemic perfusion is generally normal with right-to-left shunting lesions unless hypoxemia becomes severe enough to impair O 2 delivery to tissues. Right-to-left shunting produces two pathophysiologic problems: (1) reduced PBF resulting in systemic hypoxemia and cyanosis, and (2) increased impedance to right ventricular ejection, which may ultimately lead to ventricular dysfunction and RV failure. However, the physiologic mechanisms designed to compensate for pressure overload rarely create abnormalities in systolic or diastolic function early in the natural history of the disease process. In contrast to lesions that produce excessive ventricular volume, lesions that produce isolated pressure overload typically require years to cause ventricular dysfunction and failure.
Mixing Lesions
Mixing lesions constitute the largest group of cyanotic congenital heart defects (see Table 78.1 ). In these defects, the mixing between the pulmonary and the systemic circulation is so large that the systemic and pulmonary artery O 2 saturations approach each other. The pulmonary-to-systemic flow ratio <SPAN role=presentation tabIndex=0 id=MathJax-Element-4-Frame class=MathJax style="POSITION: relative" data-mathml='Q˙p/Q˙s’>Q˙p/Q˙sQ˙p/Q˙s
Q ˙ p / Q ˙ s
is independent of shunt size, and is completely dependent on vascular resistance or outflow obstruction. The pulmonary and systemic circulations tend to be in parallel with one another rather than in series (see Table 78.1 ). In patients with no outflow obstruction, flow to the systemic or pulmonary circulation depends on the relative vascular resistances of both circuits, such as with univentricular hearts or double-outlet RV. If SVR exceeds PVR, as in the typical circumstance, the tendency is toward excessive PBF, and the predominant pathophysiologic process is left-to-right shunting. These patients have increased PBF, ventricular volume overload, and a gradual elevation of PVR over time. If PVR exceeds SVR, as may occur episodically in ductal-dependent lesions such as hypoplastic left heart syndrome (HLHS), systemic blood flow predominates and PBF dramatically decreases, causing hypoxemia ( Table 78.2 ).
PDA Provides Systemic Flow | PDA Provides Pulmonary Flow |
---|---|
Coarctation of the aorta | Pulmonary atresia |
Interrupted aortic arch | Critical pulmonary stenosis |
Hypoplastic left heart syndrome | Severe subpulmonic stenosis with VSD |
Critical aortic stenosis | Tricuspid atresia with pulmonic stenosis |
In patients with a mixing lesion and left ventricular outflow obstruction, PBF may be sufficiently excessive to impair systemic perfusion. In patients with mixing lesions and a right ventricular outflow obstruction, such as a single ventricle with subpulmonic stenosis, systemic-to-pulmonary flow can vary from balanced flow to significantly decreased PBF in which the severity of hypoxemia depends on the degree of obstruction. Typical mixing lesions include truncus arteriosus, univentricular heart, total anomalous pulmonary venous return, pulmonary atresia with large VSD, and single atrium.
Obstructive Lesions
Obstructive lesions range from mild to severe. Severe lesions manifest in the newborn period with a pressure-overloaded, diminutive, or profoundly dysfunctional ventricle proximal to the obstruction. Such lesions include critical aortic stenosis, critical pulmonic stenosis, coarctation of the aorta, and interrupted aortic arch. Although aortic and pulmonary atresia represent the most extreme variants of outflow tract obstruction, they are associated with such significant hypoplasia of the ventricle (HLHS and pulmonary atresia with intact ventricular septum, respectively) that the ventricle’s function does not contribute to the circulatory physiology. As with other critical obstructive lesions, these extreme variants have ductal-dependent circulations, but beyond that similarity they are perhaps better understood as univentricular hearts for which the management characteristics of a mixing lesion dominate in importance.
In critical neonatal left-sided obstructive defects, systemic perfusion depends on desaturated blood flow from the RV via the PDA; the coronary perfusion is supplied by retrograde flow from the descending aorta (see Table 78.2 ). In right-sided lesions, PBF is supplied from the aorta via the PDA and right ventricular function is impaired.
Pathophysiologic problems in critical neonatal left-sided heart obstructive lesions include (1) profound left ventricular failure, (2) impaired coronary perfusion with an increased incidence of ventricular ectopy, (3) systemic hypotension, (4) PDA-dependent systemic circulation, and (5) systemic hypoxemia. The pathophysiologic problems in critical neonatal right-sided heart obstructive lesions include (1) right ventricular dysfunction, (2) decreased PBF, (3) systemic hypoxemia, and (4) PDA-dependent PBF. Apart from the most extreme variants that become evident in the neonatal period, infants and children with outflow obstruction (e.g., mild-to-moderate aortic or pulmonary stenosis, coarctation of the aorta) manifest compensatory mechanisms for pressure overload, and often remain clinically asymptomatic for many years.
Regurgitant Valves
Regurgitant valves are uncommon as primary congenital defects. Ebstein malformation of the tricuspid valve is the only pure regurgitant defect manifesting in the newborn period. However, regurgitant lesions are frequently associated with an abnormality of valve structure, such as incomplete or partial AV canal defect, truncus arteriosus, and TOF with an absent pulmonary valve. The pathophysiology of regurgitant lesions includes (1) volume-overloaded circulation and therefore (2) progression toward ventricular dilation and failure.
When considering the incidence of all the congenital heart defects, three uncomplicated left-to-right shunts (VSD, ASD, PDA) and two obstructive lesions (pulmonic stenosis, aortic coarctation) constitute 60% of all congenital cardiac defects. Mixing lesions, complicated obstructive defects, and right-to-left shunting lesions account for the vast majority of the remaining 40%. The latter group of defects, which are more difficult to manage, have a significantly higher morbidity and mortality rate.
Chronic Consequences of Congenital Heart Disease
The chronic effects of CHD are a consequence of the imposed hemodynamic stress of the defect or the residua and sequelae after cardiac surgery. These effects continue to alter normal growth and development of the cardiovascular system and other organ systems throughout life. Complete surgical cures are rarely achieved, and some repairs are palliative rather than corrective; therefore, abnormalities before and after repair produce long-term effects in patients with CHD. Many of the abnormalities are trivial and have no major import. Others affect major organ system processes, such as ventricular function, the conduction system of the heart, central nervous system (CNS) growth, or PBF. Whether anesthetizing these patients for their primary or subsequent cardiac repair or for noncardiac surgery, these chronic changes should be ascertained and reflected in the anesthetic plan.
The myocardium is continually remodeled by specific hemodynamic stresses in utero and throughout life. Abnormal hemodynamic loading conditions associated with CHD interrupt the normal ventricular modeling process ( Fig. 78.2 ). Abnormal ventricular remodeling typically begins in utero and stimulates an increase in ventricular mass. Increased ventricular mass is due to both hyperplasia and hypertrophy of myocytes in response to altered wall stress on the developing ventricle. The resultant biomechanical deformation of the ventricle alters its geometry, affecting normal systolic and diastolic function.

Abnormalities of ventricular performance at rest and with exercise can be detected in patients with chronic hemodynamic overload and complex cyanotic lesions. These abnormalities in ventricular function are the consequences of chronic ventricular overload, repeated episodes of myocardial ischemia, and residua or sequelae of surgical treatment (ventriculotomy, altered coronary artery supply, inadequate myocardial protection). Although chronic volume or pressure overload of the LV results in CHF, the compensatory mechanisms for pressure overload create less physiologic disturbance than volume overload, particularly in diastolic function. Consequently, CHF occurs later in the natural history of isolated obstructive lesions that do not require treatment in the neonatal period. Similarly, chronic right ventricular volume overload as seen in pulmonic insufficiency after TOF repair is more likely to be associated with chronic ventricular dysfunction and failure than a pressure-loaded RV that manifests with residual pulmonic stenosis. In fact, the most potent combination for inducing ventricular dysfunction and failure occurs when a pressure overload is superimposed on a dilated, volume-overloaded ventricle (e.g., postoperative TOF with pulmonary insufficiency and branch pulmonary artery stenosis).
Initial manifestations of CHF reflect alterations in ventricular compliance that result from a variety of biophysical responses to abnormal loading conditions. The ventricular dilation and compensatory hypertrophy that accompany excessive intravascular volume provide effective compensation to preserve normal systolic wall stress, but alterations in diastolic wall stress become evident ( Fig. 78.3 ). Ultimately, chronic or severe pressure overload causes similar changes as the resultant myocardial hypertrophy outgrows vascular supply and results in ischemia and fibroblast proliferation. Permanent changes in myocardial structure and function are the end result.

In patients with cyanotic heart defects, the long-term compensation for chronic hypoxemia is major redistribution of organ perfusion with selected blood flow to the heart, brain, and kidney and decreased flow to the splanchnic circulation, skin, muscle, and bone. Chronic hypoxemia is associated with increased work of breathing in an attempt to increase O 2 uptake and delivery. The most dramatic complications are decreased rate of somatic growth, increased metabolic rate, and an increase in hemoglobin concentrations.
Congenital syndromes may have associated CHD that will influence long-term outcome ( Table 78.3 ).
Syndrome | Lesion | Cardiac Lesion | Comments |
---|---|---|---|
Syndromes With Airway Issues and CHD | |||
CHARGE syndrome (association) | VSD, ASD, PDA, TOF | Micrognathia, possible difficult airway | |
Edwards syndrome | Trisomy 18 | VSD, ASD, PDA | Micrognathia, small mouth, difficult intubation |
Di George sequence | Microdeletion 22q11.2 | Aortic arch and conotruncal lesions | Short trachea—tendency to endobronchial intubation |
Goldenhar syndrome | VSD, PDA, TOF, CoA | Maxillary and mandibular hypoplasia, C-spine anomalies—difficult intubation | |
Hurler syndrome | MPS 1, storage disorder | Multivalvular disease, CAD, cardiomyopathy | Macroglossia, short neck—extremely difficult intubation |
Noonan syndrome | PS, ASD, cardiomyopathy | Short webbed neck, macrognathia—difficult intubation | |
Turner syndrome | Monosomy X | LVOT O, AS, HLHS, CoA | Micrognathia, webbed neck—difficult intubation |
VATER association | VSD, TOF, ASD, PDA | Potential for difficult intubation | |
Syndromes With Risk for Arrhythmias | |||
Long QT syndrome (LQTS) | Torsade de pointes, SCD | ||
Brugada syndrome | VT/VF/SCD | ||
Arrhythmogenic right ventricular dysplasia (ARVD) | VT/SCD | ||
Catecholaminergic polymorphic ventricular tachycardia | Polymorphic VT/SCD | ||
Wolff-Parkinson-White syndrome | SVT | ||
Maternal lupus | CCHB in the newborn | ||
Chromosomal Disorders Associated With CHD | |||
Down syndrome | Trisomy 21 | VSD, ASD, CAVC | |
Edwards syndrome | Trisomy 18 | VSD, ASD, PDA | |
Patau syndrome | Trisomy 13 | VSD, PDA, ASD | |
Turner syndrome | Monosomy X | LVOT O, AS, HLHS, CoA | |
3p−syndrome | Deletion 3p | CAVC | |
Cri du chat syndrome | Deletion 4p | Variable | |
8p−syndrome | Deletion 8p | CAVC | |
9p−syndrome | Deletion 9p | VSD, PDA, PS | |
Williams syndrome | Microdeletion 7q11 | SVAS, SVPS, branch PS | |
Smith-Magenis syndrome | Microdeletion 17p11.2 | ASD, VSD, PS, AV valve malformations | |
Miller-Dieker syndrome | Microdeletion 17p13.3 | TOF, VSD, PS | |
CHARGE association | VSD, ASD, PDA, TOF | Coloboma, heart, choanal atresia, retardation, genital and ear anomalies |
Surgical Procedures and Special Techniques
The ultimate objectives for congenital heart surgery are (1) physiologic separation of the circulation, (2) relief of outflow obstruction, (3) preservation or restoration of ventricular mass and function, (4) normalization of life expectancy, and (5) maintenance of quality of life. The available surgical procedures to accomplish these objectives are diverse and complex ( Table 78.4 ). In general, operations performed for congenital heart defects can be divided into corrective and palliative procedures. The type and timing of repair depend on the age of the patient, the specific anatomic defect, and the experience of the surgeon and the team (see Table 78.4 ).
Anatomic Defects | Palliation | Complete Repair |
---|---|---|
Tetralogy of Fallot (TOF) | VSD closure and RVOT patch | |
With PA atresia | Shunt | |
With anomalous right coronary artery | Rastelli | |
HLHS | Norwood I /transplant | |
Transposition of the great arteries | Arterial switch | |
Unfavorable coronary anatomy | Atrial switch (Senning) | |
Tricuspid atresia | Shunt followed by Fontan | |
Pulmonary atresia with VSD | Shunt followed by Fontan | |
With intact septum | Shunt followed by Fontan | |
Critical aortic stenosis | Aortic valvotomy | |
Interrupted aortic arch | End-to-end anastomosis/reverse subclavian flap/tube graft | |
Total anomalous pulmonary | Anastomosis of pulmonary veins to left atrium venous return and ASD closure | |
Single ventricle/normal PAs | Band followed by Fontan | |
With small PAs | Shunt followed by Fontan | |
Truncus arteriosus | RV-PA conduit and VSD closure | |
Atrioventricular canal | Repair valve clefts/patch closure of ASD/attach valves to patch |
Palliation in infancy is usually performed when anatomic parts are missing, as in pulmonary atresia (absent RV and pulmonary artery), tricuspid atresia (absent RV and tricuspid valve), HLHS (aortic atresia and hypoplastic LV), univentricular heart (absent RV or LV), and mitral atresia (absent LV). These palliative procedures can be further subdivided into those that increase PBF, those that decrease PBF, and those that increase mixing (see Table 78.4 ). Palliative procedures that increase PBF include shunts (Blalock-Taussig, central, and Glenn), outflow patch, and enlargement of the VSD. Those that decrease PBF include pulmonary artery banding and ligation of a PDA. Those that improve intracardiac mixing include atrial septostomy (balloon, blade, and Blalock-Hanlon).
The improvements in surgical technique, coupled with advancements in anesthetic and technologic support, make repair in early infancy not only feasible but in many cases preferable. Currently, repair in infancy can be offered for a number of congenital heart defects, as shown in Table 78.4 . The timing of surgical intervention reflects medical necessity, physiologic and technical feasibility, and optimal outcome. Cardiac defects that require a PDA to sustain sufficient systemic blood flow or PBF (e.g., pulmonary atresia, HLHS, interrupted aortic arch, critical aortic stenosis, and critical pulmonic stenosis) require an intervention in the neonatal period. A variety of defects are optimally repaired in early infancy. Lesions such as transposition of the great arteries (TGA) may exhibit better left ventricular function if the arterial switch operation is performed in the first few weeks of life when the PVR has recently been high enough to increase left ventricular systolic pressure, whereas other repairs may manifest less volatile postoperative physiology if deferred a few weeks or months until PVR has consistently fallen (e.g., TOF, AV canal defect). Each defect may have mitigating factors for which deferred definitive repair will enable an optimal surgical result (e.g., TOF with aberrant coronary branching pattern or multiple VSDs; TGA with VSD and severe left ventricular outflow tract obstruction).
Pediatric cardiovascular surgery aims to preferentially repair defects in infancy rather than palliate. This trend reflects improved technical capabilities coupled with a desire to limit the morbidity and mortality associated with long-term medical management and the sequelae of multiple palliative operations. Early correction should decrease the incidence of the chronic complications of CHD, such as the problems associated with ventricular overload, cyanosis, and pulmonary vascular obstructive disease. Early repair also may have the selective advantage of enhancing organ system protection during repair because of poorly understood factors promoting resistance to injury and enhanced recovery potential (i.e., enhanced plasticity). With the continued improvement in surgical techniques and the early treatment of CHD, specific organ systems such as the brain, heart, and lungs will be spared the detrimental effects of chronic derangements of hemodynamics and O 2 delivery.
Procedures for the treatment of CHD continue to evolve to decrease long-term morbidity and enhance survival. For example, the long-term problems with right ventricular dysfunction and failure associated with the Mustard procedure for repair of TGA encouraged many surgical groups to develop the neonatal arterial switch operation, which probably provides an anatomic correction with better long-term results. A second example of the continuing evolution of technique is surgery for TOF. Long-standing pulmonary insufficiency after right ventricular outflow repair for TOF is associated with right ventricular dysfunction and failure. Preservation of the pulmonary valve at initial repair using a combined transatrial and transpulmonary approach during correction and the early insertion of a pulmonary homograft in the setting of pulmonary insufficiency are techniques employed to avoid the long-term problems of right ventricular dysfunction and failure.
Surgery for HLHS, once considered a fatal disease, has achieved significant long-term survival after a series of staged reconstructive procedures. The use of RV-PA conduits as an alternative to traditional systemic-to-pulmonary shunts confers some advantage in survival after stage 1 palliation owing to elimination of diastolic runoff into the pulmonary circulation with concurrent unloading of the systemic RV. Myocardial perfusion improves with higher diastolic pressures, no run off to the pulmonary circulation, and decreased myocardial work. The long-term impact of a right ventriculotomy in a univentricular heart is unknown. In 2008, the Pediatric Heart Network, an organization sponsored by the National Institutes of Health, completed enrollment in a randomized control trial comparing the effects of modified Blalock-Taussig shunt (mBTS) to RV-PA conduits as part of the stage 1 Norwood procedure. Recent results revealed infants treated with an RV-PA shunt had improved survival over those with mBTS, although the long-term outcomes were not different.
Neurologic outcome after surgical repair is an ongoing concern. Preoperative cerebral blood flow (CBF) was shown to be diminished in patients with a variety of congenital heart defects, and low CBF values were associated with periventricular leukomalacia. Some centers routinely advocate regional low-flow cerebral perfusion and measurement of regional cerebral O 2 saturation index and CBF velocity using transcranial Doppler imaging during arch reconstruction in this population. Reductions in the regional cerebral O 2 saturation index or CBF greater than 20% of baseline are treated aggressively in an attempt to increase cerebral O 2 delivery by increasing the mean perfusion pressure, red blood cell (RBC) transfusion, and maintenance of high normal levels of Pa CO 2 to achieve cerebral vasodilation.
Techniques have evolved to a “three-region” perfusion strategy for aortic arch reconstruction in the Norwood procedure. This technique involves direct perfusion of the coronaries and distal thoracic aorta as well as continuous cerebral perfusion via innominate cannulation. The arch repair occurs from distal to proximal at warmer patient temperatures, theoretically allowing decreased coronary and splanchnic ischemic times, decreasing the risk of cardiac dysfunction and abdominal organ damage, and mitigating the negative hypothermic effects on the hematologic system.
Surgical management has evolved in a broader application of certain surgical procedures initially designed for a specific defect. For example, modifications of the Fontan operation, which was originally devised for patients with tricuspid atresia, are now being used to repair a variety of univentricular hearts, including HLHS. Initially, the wider application of the Fontan operation to include complex defects once considered inoperable was associated with a rise in morbidity and mortality. However, this trend has been reversed in recent years by several groups who have demonstrated improved outcome with the staging of the operation (superior cavopulmonary anastomosis, subsequent completion of the Fontan operation), the creation of a fenestration between the RA and LA at the time of the Fontan operation, and the use of modified ultrafiltration (MUF) at least in the early years.
However, as patients who undergo the Fontan procedure grow older, they present with the unique pathophysiologic challenges of refractory arrhythmias, a failing single ventricle, protein-losing enteropathy, and plastic bronchitis. Most of these adults are cared for in a combined pediatric and adult cardiac program and require intensive multidisciplinary care to optimize cardiorespiratory status. Ingenuity and innovation such as demonstrated with the Fontan procedure have permitted continued improvements in survival for all patients with CHD. As incisions in the myocardium become smaller and sutures more precisely placed, and as improvements in surgical techniques continue to evolve, the complications of ventricular dysfunction, arrhythmias, and residual obstruction should decline, contributing to improved patient quality of life.
One final difference unique to congenital heart surgery that has a major impact on anesthetic management relates to the type of cardiopulmonary support. Because of the complexity of repair in small patients, surgery often requires significant alterations in the bypass techniques, such as the use of deep hypothermic CPB at temperatures of 18°C and total circulatory arrest. Despite widespread use of these techniques during CPB, their physiologic effects on major organ system function are just beginning to be understood. These effects are discussed in subsequent sections.
Anesthetic Management
Preoperative Management
Anesthetic Evaluation
Caring for children with CHD presents the anesthesiologist with a wide spectrum of anatomic and physiologic abnormalities. Patients range from young, healthy, asymptomatic children who are having closure of a small ASD to the neonate with HLHS requiring aggressive perioperative hemodynamic and ventilatory support. Intertwined with the medical diversity of these patients are the psychological factors affecting both the patient and their parents. Preparation of the patient and the family is time-consuming, but omitting or compromising this aspect of patient care is a major deterrent to a successful outcome and patient and parental satisfaction. This team-oriented approach also serves as a safeguard to prevent errors and omissions in the exacting perioperative care necessitated by the complexity of cardiac surgery for CHD. The preoperative visit offers the family the opportunity to meet the surgeon and anesthesiologist.
Parents should be questioned about the general health and activity of their child. Fundamentally, a child’s general health and activity will reflect cardiorespiratory reserve. Deficiencies may point toward cardiovascular or other systems that may influence anesthetic or surgical risk. Does the child have impaired exercise tolerance? Is the child gaining weight appropriately, or exhibiting signs of failure to thrive on the basis of cardiac cachexia? Does the child exhibit signs of CHF (diaphoresis, tachypnea, poor feeding, recurrent respiratory infections)? Is there progressive cyanosis or new onset of cyanotic spells? Any intercurrent illness such as a recent upper respiratory tract infection or pneumonia must be ascertained. Lower respiratory tract infections may require a delay in proposed surgery, based on the negative impact that airway reactivity and elevations in PVR may have on surgical outcome. Recurrent pneumonia is frequently associated with pulmonary overcirculation and altered lung compliance in patients with increased PBF.
A good history will delineate previous surgical and cardiologic interventions, which may impact both surgical and anesthetic plans for the current procedure. Patients who have had their subclavian artery sacrificed for a subclavian flap angioplasty to correct coarctation or a Blalock-Taussig shunt will not accurately display systemic arterial pressure or perhaps even pulse oximetry readings when the monitoring is applied to the affected side. Likewise, children who have femoral venous occlusion after catheterization are not candidates for femoral venous access, particularly for femoral CPB should sternotomy prove impossible. It is equally important to obtain current medications, previous anesthetic problems, and family history of anesthetic difficulties.
In the modern era of echocardiography and cardiac catheterization, physical examination rarely contributes additional anatomic information about the underlying cardiac lesion. However, it is extremely useful in assessing the overall clinical condition of the child. For example, an ill-appearing, cachectic child in respiratory distress has limited cardiorespiratory reserve and the use of excessive premedication or a prolonged inhaled induction of anesthesia could result in significant hemodynamic instability.
Concurrent Medications and Drug Interactions
Drug interactions are common both among the co-therapeutic cardiovascular agents and between hemodyamic drugs and anesthetic drugs. An understanding of the mechanisms and interactions is useful to the pediatric cardiovascular anesthesiologist. Some common cardiovascular medications and anesthesia considerations are shown in Table 78.5 .
Cardiac Drug Class | Interactions | Considerations |
---|---|---|
Angiotensin-converting enzyme inhibitors | Hypotension with induction of general anesthesia | Consider withholding morning dose, or reducing dosage, in hypotensive patients; avoid fixed dose induction regimens with drugs having a profound vagomimetic effect |
β-Blockers | Acute withdrawal can precipitate tachycardia and arrhythmias; can potentiate hypotension with volatile anesthesia; can decrease response to inotropic agents | Continue in the perioperative period |
Calcium channel blockers | May augment the negative inotropic and chronotropic effects of volatile anesthesia | Continue in the perioperative period |
Diuretics | Hypovolemia/hypokalemia; may augment effect of neuromuscular blocking agents | Discontinue preoperatively |
Antiarrhythmics | Can be proarrhythmic with inotropes, electrolyte disturbances; high catecholaminergic states; can interact with other antiarrhythmics and precipitate bradycardia | Avoid electrolyte imbalance Avoid drugs that are proarrhythmic Monitor carefully |
α 2 -Agonists | Reduces perioperative shivering, ischemia, anesthetic and analgesic requirements | Continue into the perioperative period with appropriate monitoring |
Pediatric oncology patients presenting for cardiac or noncardiac procedures may manifest higher cardiovascular risk because of cardiotoxic chemotherapy. Common cardiotoxic agents include the antimetabolite 5-fluorouracil, the anthracycline antibiotics doxorubicin and daunorubicin, and the alkylating agent cyclophosphamide. The acute form of toxicity is characterized by acute ST segment/T wave changes on the electrocardiogram (ECG), serious dysrhythmias, and CHF associated with pericardial effusion. Chronic cardiotoxic HF is cumulative, dose related, and unresponsive to digoxin therapy. Serious cardiomyopathy can occur and is related to dose, irradiation, and use of an anthracycline. The mortality rate can exceed 50%. These patients should undergo thorough preoperative evaluation, including a full blood cell count, assessment of renal and hepatic function and coagulation parameters, and an echocardiogram. An isoflurane/nitrous oxide (N 2 O)-based anesthetic might confer better hemodynamic stability than opioid-based anesthesia in such patients.
Anesthetics can induce torsade de pointes, a malignant arrhythmia. Risk factors include female gender, electrolyte imbalances such as hypokalemia and hypomagnesemia, genetic ion channel polymorphisms of congenital long QT syndrome (LQTS), subclinical LQTS, baseline QT prolongation, and use of QT-prolonging drugs especially in high concentrations or as rapid intravenous infusions. Conditions with reduced repolarization reserve such as CHF or digoxin toxicity can also precipitate torsade de pointes. Drugs that may cause torsade de pointes in patients with congenital LQTS are shown in Table 78.6 . The website https://crediblemeds.org provides an updated list of drugs that prolong the QT interval.
Drug Category | Drug Name |
---|---|
Antiarrhythmics | Amiodarone |
Procainamide | |
Disopyramide | |
Ibutilide | |
Quinidine | |
Sotalol | |
Antipsychotics | Chlorpromazine |
Haloperidol | |
Thioridazine | |
Mesoridazine | |
Antimicrobials | Erythromycin |
Clarithromycin | |
Miscellaneous | Cisapride |
Arsenic | |
Methadone | |
Droperidol | |
Domperidone | |
Dolasetron | |
Ondansetron | |
Glycopyrrolate |
Traditionally, patients undergoing cardiac surgery have blood drawn for laboratory evaluation as standard of care (hemoglobin, electrolytes, type, and screen). Recently the utility of this practice has been questioned especially in the patient coming from home. These tests are expensive, utilize significant hospital resources, cause pain and anxiety to the patient, and rarely lead to a change in care. A more thoughtful, directed, and individualized strategy can limit costs and discomfort without sacrificing patient safety. On the other hand, special populations such as patients with trisomy 21, cyanotic heart disease, and those on antiplatelet therapy may require additional specific testing.
An increased hematocrit in a normovolemic child gives an indication of the magnitude and chronicity of hypoxemia. A hematocrit more than 60% may predispose to capillary sludging and secondary end organ damage, including stroke. Despite these risks, liberalized guidelines for nothing by mouth that permit children to consume clear liquids up to 2 hours before anesthetic induction have virtually eliminated the need to admit these patients to the hospital early for preoperative intravenous hydration.
Echocardiography with Doppler color flow imaging (echo-Doppler) is an invaluable tool that provides a noninvasive means of assessing intracardiac anatomy, blood flow patterns, and estimates of physiologic data. For many cardiac defects, more invasive studies are generally not required if a good echocardiographic assessment is made. Echo-Doppler imaging is especially helpful for defining intracardiac abnormalities. Extracardiac abnormalities, such as pulmonary artery or vein stenosis, are more difficult to define by echo-Doppler and may require computed tomography (CT) or cardiac catheterization. The ability to interpret anatomy and physiology accurately requires a skilled echocardiographer, reaffirming the need for a well-integrated interactive team. Although the complexities posed by extreme anatomic variation and changing loading conditions render intraoperative echo-Doppler challenging even for experienced echocardiographers, the pediatric cardiac anesthesiologist should develop some familiarity with its capabilities and limitations so as to participate in critical intraoperative management decisions.
Magnetic resonance imaging (MRI) of the heart and major vessels has become a very useful noninvasive imaging tool in children with heart disease. Typically, MRI is used for segmental description of cardiac anomalies; evaluation of thoracic aortic anomalies; noninvasive detection and quantification of shunts, stenoses, and regurgitations; evaluation of conotruncal malformations and complex anomalies; identification of pulmonary and systemic venous anomalies; and postoperative studies and evaluation of CHD in adult patients. MRI is particularly useful in quantifying ventricular function, regional wall motion, valvular competence, and velocity flow mapping. It is especially useful for imaging the aortic arch, pulmonary arteries, and the mediastinal vessels in children with complex CHD. Lesions for which MRI provides accurate and useful information include coarctation of the aorta, anomalies of the pulmonary arteries, anomalous pulmonary venous connections and persistent left superior vena cava, and intracardiac baffles, conduits, and shunts. MRI is also useful in older patients with poor acoustic windows and patients with chest wall deformities. It may be an alternative to cardiac catheterization in select patients and may provide noninvasive assessment of coronary anomalies, myocardial perfusion defects, and the detection of conditions associated with myocardial scarring (e.g., arrhythmogenic RV dysplasia). Even more novel, MRI images are now being used to reconstruct complex lesions using 3D printers to build a model of the heart to help plan the surgical procedure. Adenosine stress cardiac MRI is used to delineate areas of inducible ischemia. However, physiologic data such as O 2 saturations cannot be obtained with MRI.
Anesthetic considerations remain the same as for all cardiac lesions, with the additional concerns for MRI safety and restricted access to an anesthetized patient with suboptimal monitors. MRI scans are prolonged and traditionally require absolute patient immobility, with control of ventilator parameters to obtain optimal images. However, with advances in technology such as respiratory gating and use of free-breathing protocols, images can be acquired with the patient spontaneously breathing. This eliminates the need for general anesthesia with an endotracheal tube and breath-holds, thus allowing for intravenous sedation with spontaneous breathing instead.
Cardiac catheterization remains the gold standard for assessing anatomy and physiologic function in CHD. Although many anatomic questions can now be reliably answered noninvasively, catheterization remains a vital tool for cases that present complex anatomic questions or require knowledge of physiologic data. Important catheterization data for the anesthesiologist include the following:
- 1.
Child’s response to sedative medications
- 2.
Pressure and O 2 saturation in all chambers and great vessels
- 3.
Location and magnitude of intracardiac and extracardiac shunt <SPAN role=presentation tabIndex=0 id=MathJax-Element-5-Frame class=MathJax style="POSITION: relative" data-mathml='Q˙p/Q˙s’>Q˙p/Q˙sQ˙p/Q˙s
Q ˙ p / Q ˙ s
- 4.
PVR and SVR
- 5.
Chamber size and function
- 6.
Valvular anatomy and function
- 7.
Distortion of systemic or pulmonary arteries related to prior surgery
- 8.
Coronary artery anatomy
- 9.
Anatomy, location, and function of previously created shunts
- 10.
Acquired or congenital anatomic variants that might have an impact on planned vascular access or surgery
Careful review of the cardiac catheterization data and an understanding of their potential impact on the operative and anesthetic plan are essential. Not all medical problems can be evaluated and corrected preoperatively; the surgeon, cardiologist, and anesthesiologist must discuss the potential management problems and need for further evaluation or intervention before arrival in the operating room. Appropriate communication and cooperation will optimize patient care and facilitate perioperative clinical management.
Intraoperative Management
Operating Room Preparation
Advance, careful preparation of the operating room and anesthesia equipment is essential. The anesthesia machine must have the capacity to provide air, O 2, and N 2 O to help balance pulmonary and systemic blood flow. Some anesthetic machines may be equipped with carbon dioxide (CO 2 ) as an additional gas that can be added to help balance the circulations. NO is invaluable to help lower the PVR and is usually added into the inspiratory limb of the circuit via a separate machine, which then also allows continuous NO administration, also while transporting the patient. Intravenous tubing must be free from air bubbles to prevent paradoxical air embolism, and air filters should be added to all infusion lines. Resuscitative drugs, labeled and ready for administration, should include succinylcholine, calcium gluconate or calcium chloride, sodium bicarbonate, atropine, lidocaine, phenylephrine, and epinephrine. An inotropic infusion, usually epinephrine or dopamine, should be premixed and ready for administration in high-risk cases, but additional infusions are prepared if their need is strongly suspected (e.g., milrinone, vasopressin). For all pediatric cases, certain anesthetic drugs should be available (etomidate, propofol, ketamine). No single drug can be recommended; how a particular drug is used is more important. In pediatric cardiac anesthesia, many patients have limited reserve and high endogenous catecholamine levels released in an adaptive response to their underlying cardiac disease. The resuscitative drugs should therefore be prepared and immediately available before anesthetic induction.
For congenital heart surgery, the ability to alter body temperature rapidly for cooling and rewarming is essential. During deep hypothermic CPB, patients are cooled to 18°C. Surface cooling with a heating and cooling water mattress, ice in watertight bags, and an efficient room and ambient temperature control system are important in the operative management of these patients.
Physiologic Monitoring
The specific monitoring used should depend on the child’s condition and the magnitude and nature of the planned surgical procedure. The perioperative monitoring techniques available are listed in Box 78.2 . Noninvasive monitoring equipment is ideally placed before induction of anesthesia, although the anesthesiologist may elect to defer application of monitoring devices in the crying child until immediately after the induction. Standard monitoring includes electrocardiography, pulse oximetry, capnography, and an appropriate-sized blood pressure cuff (either oscillometric or Doppler). Additional monitoring includes an indwelling arterial catheter and temperature probes. Foley catheters are generally employed when surgical intervention entails CPB or might produce renal ischemia, or when the anesthetic management includes a regional technique associated with urinary retention. Most centers routinely employ percutaneously placed central venous pressure (CVP) monitoring or alternatively, directly placed transthoracic atrial lines by the surgeons to help with separation from CPB and the hemodynamic management in the postoperative period.
Cardiopulmonary System
Esophageal stethoscope
Electrocardiogram
Standard five-lead system, ST-T wave analysis, esophageal electrocardiographic lead
Pulse oximetry
Automated oscillatory blood pressure
Capnograph
Ventilator parameters
Indwelling arterial catheter
Central venous pressure catheter
Pulmonary artery catheter
Transthoracic pressure catheter
Left or right atrium, pulmonary artery
Echocardiography with Doppler color flow imaging
Epicardial or transesophageal
Central Nervous System
Peripheral nerve stimulator
Processed electroencephalography
Specialized
Cerebral blood flow: Xenon clearance methodology
Cerebral metabolism: Near-infrared spectroscopy, oxygen consumption measurements
Transcranial Doppler
Jugular venous bulb saturations
Temperature
Nasopharyngeal, rectal, esophageal, tympanic
Renal Function
Foley catheter
Continuous monitoring of arterial pressure is possible only through an indwelling intraarterial catheter. In young children, cannulation of the radial artery with a 22- or 24-gauge catheter is preferred. In older children and adolescents, a 20-gauge catheter may be substituted. Careful inspection, palpation, four-extremity noninvasive blood pressure determinations, and ultrasound use help ensure that previous or currently planned operative procedures (e.g., a previous radial artery cutdown, subclavian flap for coarctation repair, or Blalock-Taussig shunt) do not interfere with the selected site of arterial pressure monitoring. Other sites available for cannulation include the ulnar, femoral, axillary, and umbilical (in neonates) arteries. Cannulation of the posterior tibial or dorsalis pedis arteries is not usually sufficient for complex operative procedures. Peripheral arterial catheters, principally of the distal lower extremities, function poorly after CPB and do not reflect central aortic pressure when distal extremity temperature remains low.
Myocardial and cerebral preservation is maintained principally through hypothermia; therefore, the accurate and continuous monitoring of body temperature is crucial. Rectal and nasopharyngeal temperatures are monitored because they reflect core temperature and brain temperature, respectively. Monitoring of esophageal temperature is a good reflection of cardiac and thoracic temperature. Tympanic probes, although a useful reflection of cerebral temperature, can cause tympanic membrane rupture.
Pulse oximetry and capnography provide instantaneous feedback concerning adequacy of ventilation and oxygenation. They are useful guides in ventilatory and hemodynamic adjustments to optimize <SPAN role=presentation tabIndex=0 id=MathJax-Element-6-Frame class=MathJax style="POSITION: relative" data-mathml='Q˙p/Q˙s’>Q˙p/Q˙sQ˙p/Q˙s
Q ˙ p / Q ˙ s
before and after surgically created shunts and pulmonary artery bands. Peripheral vasoconstriction in patients undergoing deep hypothermia and circulatory arrest renders digital O 2 saturation probes less reliable. In the newborn, the use of a tongue sensor has been advocated to provide a more central measure of O 2 saturation, with less temperature-related variability.
The use of transthoracic or transvenous pulmonary artery catheters is determined on an individual basis based on the disease process, physiologic state, and surgical intervention. For example, in children undergoing a Fontan procedure for tricuspid atresia or a univentricular heart, catheters in the Fontan pathway and the pulmonary venous atrium are especially useful. After a Fontan operation, PBF must occur without benefit of a ventricular pumping chamber. Subtle changes in preload, PVR, and pulmonary venous pressure will influence PBF and thus systemic cardiac output. Data derived from the difference between the CVP and left atrial pressure ([LAP]; also known as the transpulmonary gradient) help identify the relative importance of intravascular volume (CVP), PVR (CVP-LAP gradient), or ventricular compliance (LAP), each of which requires a different therapeutic approach.
As a general guideline, a transvenous pulmonary artery catheter may be placed using the internal jugular approach in children weighing more than 7 kg. A 5.0-Fr catheter is used for children weighing between 7 and 25 kg, and a 7.0-Fr one is used for children weighing more than 25 kg. For infants weighing less than 7 kg, percutaneous placement of a pulmonary artery catheter can be performed from the femoral vein. Occasionally, the latter technique will require fluoroscopy. The use of intraoperative transthoracic monitoring lines and echo-Doppler imaging limits the need for transvenous pulmonary artery catheters in most cases.
Special Monitoring
Intraoperative Echocardiography
The use of echo-Doppler imaging is now regarded as standard of care for almost all pediatric cardiac surgeries. Two-dimensional echocardiography combined with pulsed-wave Doppler ultrasonography and color flow mapping provide detailed morphologic and physiologic information in the majority of operative cases. Using echo-Doppler in the operating room, anatomic and physiologic data can be obtained before CPB, thus refining the operative plans. Prebypass echo-Doppler precisely defines anesthetic and surgical management. Because of the unrestricted epicardial and TEE approaches in anesthetized patients, new findings are frequently discovered and management plans changed accordingly ( Fig. 78.4 ).

Postbypass echo-Doppler evaluation is able to immediately assess the quality of the surgical repair and cardiac function by examining ventricular wall motion and systolic thickening. This technique can show residual structural defects after bypass, which can be immediately repaired, thus avoiding the patient leaving the operating room with significant residual structural defects that will require reoperation at a later time ( Fig. 78.5 ). By identifying patients with right or left ventricular contraction abnormalities after bypass, as determined by a change in wall motion or systolic thickening, echo-Doppler provides guidance for immediate pharmacologic interventions. Importantly, postbypass ventricular dysfunction and residual structural defects identified by echo-Doppler imaging are associated with an increased incidence of reoperation and higher morbidity and mortality rates. Thus, this monitoring tool is helpful in assessing surgical repair and identifying operative risk factors, which will hopefully improve outcomes.

Two techniques for intraoperative echo-Doppler imaging have been described: epicardial and TEE. Using TEE, the probe is placed after induction of anesthesia and intubation and is then available for monitoring of the patient. The advantage of this technique is its utility as a continuous monitor of cardiac structure and function, without interrupting surgery. Because of its ideal imaging location, TEE has been especially helpful in evaluating pulmonary venous return and the integrity of the left AV valve after mitral valvuloplasty, complete AV valve repair, and correction of complex CHD. Early limitation in views has been virtually eliminated as a result of clinical experience and improved biplane images. Pediatric biplane TEE probes have now extended the patient weight limits to neonates between 2.5 and 3 kg. Potential hazards of TEE that merit particular vigilance include descending aorta and airway compression because of probe size or during probe flexion. There have been reports of esophageal damage during hypothermia and low or no flow states because of the heat energy the probe produces while connected to the TEE machine, leading most institutions to pause the imaging ability of the probe, disconnect the probe from the machine, or even remove it during the CPB period of the procedure.
A second technique for intraoperative echocardiographic analysis in children is the epicardial approach. This approach requires passing a clean, short-focused 5.0- or 7.0-MHz transducer over the anesthesia screen into a sterile sheath, where it then can be placed on the epicardial surface of the heart. This technique best facilitates the probe manipulations necessary for thorough interrogation of the major structures and dynamic function of the heart. The advantage of this approach is that all views can be obtained in patients of any size. Among the disadvantages are the need for sufficient operator skill and experience to perform the manipulations, the need to interrupt surgery to manipulate the probe, and the possible deleterious impact of direct myocardial mechanical manipulation. Given current TEE capabilities, epicardial imaging is rarely employed.
Specialized Central Nervous System Monitoring
The primary goal of brain monitoring is to improve our understanding of cerebral function during cardiac surgery so that effective brain protection strategies can be developed. Because many of the determinants of normal brain perfusion become externally controlled by the cardiac team during CPB, such as flow rate (cardiac output), perfusion pressure, temperature, hematocrit, and Pa CO 2 , a knowledge of the effect of these factors on the brain in neonates, infants, and children is essential. Numerous intraoperative techniques have been used for monitoring the brain to prevent secondary brain injury from hypoxia, ischemia, emboli, and electrophysiologic derangements. These have primarily included the following three modalities in isolation or combination: (1) electroencephalography (EEG) to assess perfusion-related changes in cortical activity; (2) transcranial Doppler imaging to measure arterial flow and resistance; and (3) near-infrared spectroscopy (NIRS) to provide a measure of venous-weighted, tissue oxyhemoglobin saturation. Additionally, the measurement of CBF and metabolism with specialized clinical research tools has been very important in furthering our understanding of brain function during and after surgery. Multimodal neurologic monitoring is also used to guide CPB, deep hypothermic circulatory arrest (DHCA), and regional low-flow cerebral perfusion techniques in neonatal arch reconstruction.
Electroencephalographic monitoring allows detection of ischemia or recognition of an adequate decrease in cerebral metabolic activity during hypothermia before DHCA. EEG is helpful in monitoring physiologic functions of the CNS during deep hypothermic bypass and total circulatory arrest. For example, during deep hypothermia and before total circulatory arrest, the processed EEG can identify residual cerebral electrical activity. Isoelectric silence can then be induced by further cooling and any further brain activity detected by EEG. Because this residual electrical activity during arrest is associated with ongoing cerebral metabolism, an isoelectric state may prevent ischemic injury to the brain during circulatory arrest. EEG also may be useful in detecting the level and depth of anesthesia. Postoperative electroencephalographic analysis has demonstrated subclinical seizure activity in a number of high-risk patients, potentially linking these abnormalities to poorer neuropsychologic outcome. The value of intraoperative electroencephalographic monitoring after CPB and the significance of the findings remain to be determined.
Transcranial Doppler imaging has been used primarily for research purposes in infants and allows detection of venous or arterial flow abnormalities and the detection of microemboli. This technology uses the Doppler principle to detect shifts in the frequency of reflected signals from blood in the middle cerebral artery to calculate blood flow velocity. Because the diameter of this large cerebral artery is relatively constant, flow velocity can be used to approximate CBF. Transcranial Doppler imaging has several advantages: (1) it is noninvasive, (2) it does not require radiation exposure, (3) it is a continuous monitor, and (4) it captures rapid alterations in blood flow velocity caused by temperature or perfusion changes. The limitations of transcranial Doppler monitoring include (1) reproducibility, especially at low flow rates, when small movements of the patient’s head can dramatically alter the signal, and (2) the lack of validating studies during hypothermic CPB, when hypothermia, reduced flow rates, and the laminar flow characteristics of non-pulsatile perfusion may limit the accuracy of CBF velocity measurements.
Transcranial Doppler imaging has been used to investigate the effect of CPB and DHCA on cerebral hemodynamics in children, as well as to assess the incidence of cerebral emboli. Recent studies examining the brain using transcranial Doppler have enabled several investigative groups to provide important information regarding questions of normal and abnormal brain perfusion during cardiac surgery in children. Questions regarding cerebral perfusion pressure, autoregulation, effect of Pa CO 2 , and temperature have been addressed using transcranial Doppler imaging in children. This technique also has provided qualitative information regarding the presence of gaseous emboli in the middle cerebral artery during cardiac surgery.
NIRS is a noninvasive monitor of cerebral tissue oxygenation, reflecting the balance of O 2 delivery and consumption. Cerebral NIRS reflects O 2 saturation of the venous compartment, and values correlate with jugular venous bulb saturations. There is significant interest in studying the ability of NIRS to predict outcomes, particularly on the neurodevelopmental spectrum. A study of infants undergoing the stage 1 Norwood procedure for HLHS demonstrated an association between low NIRS (particularly when less than 50%-60%) and poor neurodevelopmental outcomes. Although there was not a linear association, it provides evidence that NIRS can be used to detect clinically consequential hypoxia. Somatic and cerebral saturations are further clinically relevant in the immediate postoperative period by predicting overall morbidity and mortality in patients with HLHS stage 1 palliation. The number of minutes of cerebral desaturation below 50% predicts morbidity and serves as an early warning sign for hypoxia, bleeding, and/or low cardiac output state. A decrease of greater than 20% from baseline in renal saturation as detected by NIRS for a period of 20 minutes has been associated with a longer duration of mechanical ventilation and ICU convalescence.
A 2016 review of NIRS use in pediatric cardiac surgery identified several prospective trials, mostly observational, that found inconsistent results overall regarding improved clinical outcomes with NIRS use. Importantly, the current evidence does not provide clear thresholds for cerebral or somatic NIRS, below which the chance of morbidity would increase. Differences in longer-term cardiac, renal, or neurodevelopmental outcomes have not been demonstrated based on differences in NIRS values. Despite these reservations, NIRS remains an important clinical tool to interpret alongside other monitoring technologies.
CBF studies using xenon clearance technology have improved the understanding of cerebrovascular dynamics in young children during CPB and especially during deep hypothermia and after periods of circulatory arrest. In general, this investigational tool has described the effects of CPB, temperature, and various perfusion techniques on CBF and, indirectly, on brain metabolism ( Fig. 78.6 ). Studies using this methodology have shown that some of the mechanisms of CBF autoregulation, such as pressure-flow regulation, are lost with deep hypothermia and that cerebral reperfusion is impaired after a period of total circulatory arrest.

Induction and Maintenance of Anesthesia
The principles of intraoperative management of cardiothoracic surgical procedures are based on an understanding of the pathophysiology of each disease process and a working knowledge of the effects of the various anesthetic and other pharmacologic interventions on a particular patient’s condition. Selecting a technique for induction of anesthesia needs to consider the degree of cardiac dysfunction, the cardiac defect, the degree of sedation provided by the premedication, and the presence of an indwelling catheter. In children with good cardiac reserve, induction techniques can be quite varied in well-monitored patients. The titration of anesthetics for induction of anesthesia is more important than the specific anesthetic technique in patients with reasonable cardiac reserve. A wide spectrum of anesthetic induction techniques have been used safely and successfully, including sevoflurane, isoflurane, N 2 O, intravenous and intramuscular ketamine, and intravenous propofol, fentanyl, and midazolam. Ketamine is often used in anesthetic induction in patients with cyanotic conditions because it increases SVR and cardiac output, thereby diminishing the magnitude of right-to-left shunting. Administration of ketamine can be intravenous or intramuscular, with the caveat that an intramuscular injection may result in pain, agitation, and subsequent arterial desaturation.
Inhaled inductions are generally well received and tolerated by most children. An inhaled induction of anesthesia with sevoflurane can easily and safely be performed even in cyanotic patients, such as those with TOF ( Fig. 78.7 ). In these patients, who are at risk for right-to-left shunting and systemic desaturation, oxygenation is well maintained with a good airway and ventilation despite reduction in systemic arterial pressure. Skilled airway management and efficiency of ventilation are equally essential components of anesthetic induction. Recognizing the complexities of shunts and vascular resistance changes, as well as airway and ventilation effects on the cardiovascular system, is of primary importance during the induction of anesthesia.

After anesthetic induction, intravenous access is established or augmented as appropriate. A nondepolarizing muscle relaxant is usually administered and an intravenous opioid and/or inhaled anesthetic chosen for maintenance of anesthesia. The child is preoxygenated with 100% FiO 2 , and an endotracheal tube is carefully positioned. Some degree of alveolar preoxygenation is recommended, even in the infant whose systemic perfusion might be jeopardized by lowering PVR with resulting increase in PBF, as this maneuver delays desaturation during intubation. A nasal route is usually selected in neonates and other patients who will remain intubated postoperatively, as it may provide greater stability and patient comfort than an oral endotracheal tube. However, with the trend of earlier and even intraoperative extubation, as well as new data showing an association between nasal intubation and increased infections in children older than 6 months of age, the prevalence of nasal intubation has decreased. If the child arrives in the operating room with an endotracheal tube in place, it is prudent to assess the depth and the overall state of the endotracheal tube. Inspissated secretions in a tube with a small internal diameter can cause significant obstruction to gas flow and may be made worse during periods of bypass when humidified ventilation is discontinued. This may be minimized by placing a new endotracheal tube at the beginning of the procedure.
Because of the diverse array of congenital heart defects and surgical procedures, an individualized anesthetic management plan is essential. The maintenance of anesthesia in these patients depends on the age and condition of the patient, the nature of the surgical procedure, the duration of the CPB, and the need for postoperative ventilation. An assessment of the hemodynamic objectives designed to lessen the pathophysiologic loading conditions should be developed for each patient, taking advantage of the known qualitative effects of specific anesthetic drugs and ventilatory strategies. These individualized plans must also be integrated with the overall perioperative goals to configure the optimal anesthetic. In patients with complex defects requiring preoperative inotropic and mechanical ventilatory support, a carefully controlled induction and maintenance anesthetic with a potent opioid is generally chosen. In patients with a simple ASD or VSD, a balanced anesthetic with inhaled anesthetics, opioid titration, and the use of dexmedetomidine is preferred. This allows for intraoperative tracheal extubation and a less prolonged period of intensive care monitoring. More important than the specific anesthetic techniques and drugs is the skilled execution of the anesthetic plan, taking into account patient response to drugs, the changes associated with surgical manipulation, and early recognition of intraoperative complications.
The reported changes in arterial blood pressure and heart rate from the inhaled anesthetic in normal children are observed in pediatric cardiac surgical patients as well. We use potent inhaled anesthetics in almost all cases, including while on CPB, as part of a balanced anesthetic technique and for their direct protective effects against ischemia/reperfusion myocardial injury. Although isoflurane decreases blood pressure in neonates, infants, and children, the vasodilatory properties of isoflurane may improve overall myocardial contractility in contrast to the effects of halothane. Despite improved cardiac reserve with isoflurane, the incidence of laryngospasm, coughing, and desaturation during induction of anesthesia limits its use as an induction agent in children with congenital heart defects.
Desflurane has cardiorespiratory properties similar to those of isoflurane. Its main advantage is low blood gas and tissue solubility, allowing for rapid equilibration between the inspired and alveolar concentrations and rapid decrease of alveolar concentrations during elimination. This provides greater precision in drug dosing during the operative period and may make desflurane a more titratable adjunctive drug for pediatric cardiac anesthesia. The three main disadvantages of desflurane are potency, pungency, and negative inotropic effect. Studies in normal infants and children suggest that 1 minimum alveolar concentration of desflurane requires concentrations of 8% to 10%. Desflurane is also quite pungent, and, although its uptake is rapid, early experience with this drug for inhalation induction in children has reported a fairly high incidence of airway reactivity and laryngospasm. Although its negative inotropic effect is significantly less potent than that of halothane, desflurane should not be used as the sole anesthetic in patients with significant cardiac dysfunction.
Sevoflurane, our volatile anesthetic of choice, offers a more tolerable aroma without the magnitude of myocardial depression that accompanies halothane. In addition, its blood gas solubility is nearly as low as that of desflurane. Hemodynamically, sevoflurane tends to produce some tachycardia, particularly in older children, and preserve systemic arterial pressure. Reductions in heart rate and systemic arterial pressure are more modest in infants anesthetized with sevoflurane than in control subjects anesthetized with halothane, and the former exhibit echocardiographic evidence of normal contractility and cardiac index. This effect is particularly seen in children with trisomy 21.
Children with complex CHD and limited cardiac reserve demand an anesthetic technique that provides hemodynamic stability. Inhalation anesthetics are less well tolerated as a sole primary anesthetic in patients who have limited cardiac reserve, especially after CPB. Fentanyl is an excellent induction and maintenance anesthetic for this group of patients. Low-to-moderate doses of this opioid can be supplemented with inhalation anesthetics. Adding low concentrations of inhalation anesthetics to smaller doses of opioids shortens or eliminates the need for postoperative mechanical ventilation while maintaining the advantage of intraoperative hemodynamic stability. Postoperative mechanical ventilation will be required when a high-dose (e.g., fentanyl > 20 μg/kg) opioid technique is used. The hemodynamic effects of fentanyl at a dose of 25 μg/kg with pancuronium given to infants in the postoperative period after operative repair of a congenital heart defect include no change in LAP, pulmonary artery pressure, PVR, and cardiac index and a small decrease in SVR and mean arterial pressure. Because of its cardiovascular effects, pancuronium was an ideal neuromuscular blocking drug for pediatric heart surgery, but it is no longer available for clinical use. Therefore, either vecuronium or rocuronium are most often used. Larger doses of fentanyl at 50 to 75 μg/kg with rocuronium or vecuronium compared to doses of fentanyl at 50 to 75 μg/kg with pancuronium result in a slightly larger decrease in arterial blood pressure and heart rate in infants undergoing repair for complex congenital heart defects. Despite the wide safety margin exhibited by this opioid, a selected population of infants and children with marginally compensated hemodynamic function sustained by endogenous catecholamines may manifest more extreme cardiovascular changes with these doses. Fentanyl also has been shown to block stimulus-induced pulmonary vasoconstriction and contributes to the stability of the pulmonary circulation in neonates after congenital diaphragmatic hernia repair. Thus, the use of fentanyl may be extrapolated to the operating room, where stabilizing pulmonary vascular responsiveness in newborns and young infants with reactive pulmonary vascular beds is crucial to weaning from CPB and stabilizing shunt flow. Fentanyl in the 8 to 12 μg/kg dose range should provide sufficient analgesia, but still allow adequate ventilation efforts to allow for intraoperative extubation with stable hemodynamics during the procedure.
Children receiving sufentanil for induction of anesthesia as a single dose of 5 to 20 μg/kg have a stable preintubation period. Intubation and other stimuli such as sternotomy do not produce clinically significant alterations in hemodynamics, although changes are more than with equipotent doses of fentanyl. The use of fentanyl as an infusion (1-2 μg/kg/h) produces fewer alterations in heart rate and blood pressure. This is particularly important in infants, in whom significant hemodynamic changes are poorly tolerated. For neonates with critical CHD, sufentanil anesthetic and postoperative infusion reduce morbidity after cardiac surgery when compared with a halothane anesthetic and routine morphine postoperatively. The blunting of the stress response observed in this study probably accounted for the differences in morbidity; no comparison group representing a more typical dose of a phenylpiperidine opioid (e.g., fentanyl, 0-75 μg/kg) was included to permit conclusions as to whether such large opioid doses are optimal.
In contrast to other opioids, remifentanil, an ultra–short-acting opioid, offers the unique advantage of metabolism by nonspecific and tissue esterases, thereby limiting the potential for accumulation related to protracted elimination. Remifentanil may provide advantages in the selected group of patients for whom the blunting of endogenous responses is desirable intraoperatively but potentially deleterious at the end of the procedure. A randomized controlled trial comparing equipotent doses of alfentanil and remifentanil for outpatient pediatric surgery revealed delayed emergence, requiring naloxone only in the alfentanil group. In both adults and children, remifentanil is associated with qualitative hemodynamic changes similar to those with other opioids, a variable tendency to bradycardia, and a small decrease in arterial blood pressure.
Because of the widespread use of the opioids for pediatric cardiac surgery and the availability of invasive monitoring, the pharmacokinetics and pharmacodynamics of these drugs have been well studied. In general, the clinical pharmacology of fentanyl and sufentanil share the same age-related pharmacokinetic and pharmacodynamic features. For example, sufentanil has an increased clearance in patients 1 month to 12 years of age, comparable to adult clearance in adolescents (12-16 years of age), and a decreased clearance during the neonatal period (newborn to 1 month of age) ( Table 78.7 ). Furthermore, sequential sufentanil anesthetics in neonates with CHD show marked increases in clearance and elimination between the first week and the third or fourth week of life ( Fig. 78.8 ). The latter observation is most likely attributable to maturational changes in hepatic microsomal activity and improved hepatic blood flow from closure of the ductus venosus. The variability in clearance and elimination, coupled with limited cardiovascular reserve in the neonate during the first month of life, makes opioid dosing difficult in this age group. Careful titration of fentanyl 5 to 10 μg/kg or sufentanil 1 to 2 μg/kg or a continuous infusion technique provides the most reliable method of achieving hemodynamic stability and an accurate dose response. CPB, different institutional anesthetic practices, and individual patient differences influence pharmacokinetic and pharmacodynamic disposition of the opioids in ways that are not predictable. Even certain disease states such as TOF or pathophysiologic conditions such as increased intraabdominal pressure alter pharmacokinetic processes.
Age Group | t ½ α (min) | t ½ β (min) | Clearance (mL/kg/min) | Vdss (L/kg) |
---|---|---|---|---|
1-30 days | 23 ± 17 | 737 ± 346 | 6.7 ± 6.1 | 4.2 ± 1.0 |
1-24 months | 16 ± 5 | 214 ± 41 | 18.1 ± 2.7 | 3.1 ± 1.0 |
2-12 years | 20 ± 6 | 140 ± 30 | 16.9 ± 2.2 | 2.7 ± 0.5 |
12-18 years | 20 ± 6 | 209 ± 23 | 13.1 ± 0.4 | 2.7 ± 0.5 |

Intraoperative use of methadone is an alternative pain control strategy introduced as an answer to counter acute tolerance to fentanyl infusions in the postoperative period. Adult data suggest that intraoperative use of methadone as the primary opioid in CPB cases significantly reduced the use of other opioids in the postoperative period, improved pain scores, and enhanced patient-perceived quality of pain management. There is no pharmacokinetic data in children having CPB surgery, but available data show that the pharmacokinetic parameters in children and neonates are similar to those reported in adults, and that there is no clearance maturation with age. For non-CPB cases, a dose of 0.2 mg/kg is suggested; we have used total doses of 0.3 to 0.4 mg/kg in CPB cases and extubated intraoperatively. Other strategies to address opioid tolerance include alternating opioid drugs, instituting opioid holidays, the addition of benzodiazepines on an as-needed basis, and the use of dexmedetomidine infusions.
Dexmedetomidine is an α 2 -agonist approved by the U.S. Food and Drug Administration for sedation in adults. It has been used in pediatric anesthesia as part of a balanced technique preoperatively and intraoperatively for sedation, anxiolysis, and analgesia, and postoperatively for prevention of emergence delirium and sedation. Dexmedetomidine has significant analgesic and antiinflammatory effects, attenuates the neuroendocrine response to surgery, and has no neurotoxic effects; it is a crucial adjunct to a balanced anesthetic as it reduces the need for other analgesics and hypnotics.
The pharmacodynamic effects of dexmedetomidine when used as an infusion are generally well tolerated. The clinical effects are predictable and usually insignificant with slight lowering of both heart rate and arterial blood pressure compared to baseline. However, when administered as a rapid bolus, the first physiologic effect noted is hypertension along with heart rate slowing, lasting approximately 2 to 5 minutes before arterial blood pressure decreases.
Dexmedetomidine demonstrates cardiac conduction effects, via both direct depression of the sinus and AV nodes in the heart, and decreased sympathetic tone in the locus coeruleus. Clinically, this translates into a significant reduction of the incidence of junctional ectopic tachycardia post-CPB. However, some studies and case reports, mostly in the adult literature, have documented clinically significant bradycardia, hypotension, and even asystole with its use. It is necessary to remain vigilant and titrate dexmedetomidine carefully. Dexmedetomidine should be used with particular caution in children at risk for bradycardia or sinus or AV node dysfunction, and possibly in patients who have had a heart transplant. At our institution, dexmedetomidine is used in almost every case, with an infusion of 0.2 μg/kg/h in neonates and 0.5 μg/kg/h in all other cases initiated postinduction. The infusion is continued throughout the surgery and into the postoperative period. This practice is particularly helpful in patients that are extubated intraoperatively and we will often increase the dose to 1 to 2 μg/kg/h after extubation to keep the child calm for transport to the ICU.
Cardiopulmonary Bypass
Differences Between Adult and Pediatric Cardiopulmonary Bypass
The physiologic effects of CPB on neonates, infants, and children are significantly different from the effects on adults ( Table 78.8 ). During CPB, pediatric patients are exposed to biologic extremes not seen in adults, including deep hypothermia (18°C), hemodilution (threefold to fivefold greater dilution of circulating blood volume), low perfusion pressures (20-30 mm Hg), wide variation in pump flow rates (ranging from total circulatory arrest to 200 mL/kg/min), and differing blood pH management techniques (α-stat, pH-stat, or both sequentially). These parameters deviate far from normal physiology and affect preservation of normal organ function during and after CPB. In addition to these prominent changes, subtle variations in glucose supplementation, cannula placement, presence of aortopulmonary collaterals, and patient age affect organ function during CPB.
Parameter | Adult | Pediatric |
---|---|---|
Hypothermic temperature | Rarely below 25°C -30°C | Commonly 15°C -20°C |
Use of total circulatory arrest | Rare | Common |
Pump prime | ||
Dilution effects on blood volume | 25%-33% | 150%-300% |
Additional additives in pediatric primes | Blood, albumin | |
Perfusion pressures | 50-80 mm Hg | 20-50 mm Hg |
Influence of α-stat versus pH-stat management strategy | Minimal at moderate hypothermia | Marked at deep hypothermia |
Measured Pa co 2 differences | 30-45 mm Hg | 20-80 mm Hg |
Glucose regulation | ||
Hypoglycemia | Rare—requires significant hepatic injury | Common—reduced hepatic glycogen stores |
Hyperglycemia | Frequent—generally easily controlled with insulin | Less common— rebound hypoglycemia may occur |
Adult patients are infrequently exposed to such biologic extremes; temperature is rarely lowered below 25°C, hemodilution is more moderate, perfusion pressure is generally maintained at 50 to 80 mm Hg, flow rates are maintained at 50 to 65 mL/kg/min, and pH management strategy is less consequential because of moderate hypothermic temperatures and rare use of circulatory arrest. Variables such as glucose supplementation rarely pose a problem in adult patients owing to large hepatic glycogen stores. Venous and arterial cannulas are less deforming of the atria and aorta, and their placement is more predictable. Although superficially similar, the conduct of CPB in children is considerably different from that in adults. Marked physiologic differences in the response to CPB in children can occur. Additionally, several modifiable intraoperative factors can influence neuropsychologic morbidity ( Box 78.3 ).
Air or particulate embolus
Rate and depth of core cooling (if used)
Deep hypothermic circulatory arrest (if used)
Reperfusion injury and inflammation
Rate of core rewarming/hyperthermia
Hyperglycemia
Hyperoxia
pH management during cardiopulmonary bypass
Hematocrit management during cardiopulmonary bypass
Volume of Priming Solutions
The priming solutions used in pediatric CPB take on great importance because of the disproportionately large priming volume–to–blood volume ratio in children. In adults, the priming volume is equivalent to 25% to 33% of the patient’s blood volume, whereas in neonates and infants the priming volume may exceed the patient’s blood volume by 200%. With contemporary low-volume bypass circuits (e.g., small volume oxygenators, smaller tubing), priming volume is not more than one blood volume in a small neonate. Care must be taken, therefore, to achieve a physiologically balanced priming solution and limit the volume as much as possible. Most pediatric priming solutions, however, have quite variable levels of electrolytes, calcium, glucose, and lactate. Electrolytes, glucose, and lactate levels may be quite high if the solution includes large amounts of banked blood or quite low if a minimal amount of banked blood is added. Calcium levels are generally very low in pediatric priming solutions; this may contribute to the rapid slowing of the heart with the initiation of bypass.
The main constituents of the priming solution include crystalloid, colloid, and, if necessary, banked blood to maintain a temperature-appropriate hematocrit. Other potential supplements are fresh frozen plasma, mannitol, a buffer (sodium bicarbonate or trishydroxymethylaminomethane [THAM]), and steroids. Low concentrations of plasma proteins have been shown experimentally to impair lymphatic flow and alter pulmonary function by increasing capillary leak. Although adding albumin to the pump prime has not been shown to alter outcome in adults during CPB, one study suggested that maintaining normal colloid osmotic pressure may improve survival in infants undergoing CPB.
Whole blood, if available, is an alternative to adding both packed RBCs and fresh frozen plasma. Blood cells are added to the prime solution to maintain a postdilutional hematocrit of at least 20% to 25% (usually higher in patients with cyanotic CHD), and plasma restores levels of procoagulants. Low-volume bypass circuits enable perfusionists and anesthesiologists to share a single unit of whole blood, thereby limiting the donor.
The addition of any blood products will cause a much higher glucose load in the priming solution. Hyperglycemia may increase the risk for neurologic injury if brain ischemia occurs. Mannitol is added to promote an osmotic diuresis and scavenge O 2 free radicals from the circulation. Steroids are added to stabilize membranes and produce the theoretic advantage of reducing ion shifts during periods of ischemia, attenuating inflammation caused by CPB, decreasing low cardiac output states, and improving fluid balance in the postoperative period. Steroids, however, may raise glucose levels, which can be detrimental if there is a period of cerebral ischemia, and may suppress immune function. Steroids remain a controversial additive in priming solutions. Recent retrospective data suggest negative effects with its use and an association with decreased survival in neonates having the Norwood procedure. A number of prospective studies are ongoing to address the role of steroids in pediatric cardiac surgery.
Temperature
Hypothermic CPB is used to preserve organ function during cardiac surgery. Three distinct methods of CPB are used: moderate hypothermia (25°-32°C), deep hypothermia (18°C), and DHCA. The choice of method of bypass is based on the required surgical conditions, patient size, type of operation, and potential physiologic impact on the patient.
Moderate hypothermic CPB is the principal method of bypass employed for older children and adolescents. In these patients, venous cannulas are less obtrusive and the heart can easily accommodate superior and inferior vena cava cannulation. Bicaval cannulation reduces right atrial blood return and improves the surgeon’s ability to visualize intracardiac anatomy. Moderate hypothermia may also be chosen for less demanding cardiac repairs, such as an ASD or uncomplicated VSD. Most surgeons are willing to cannulate the inferior and superior venae cavae in neonates and infants. In these patients, however, this approach is technically more difficult and likely to induce brief periods of hemodynamic instability. Additionally, the pliability of the venae cavae and the rigidity of the cannulas may result in caval obstruction, impaired venous drainage, and elevated venous pressure in the mesenteric and cerebral circulation.
Deep hypothermic CPB is generally reserved for neonates and infants requiring complex cardiac repair. However, certain older children with complex cardiac disease or severe aortic arch disease benefit from deep hypothermic temperatures. For the most part, deep hypothermia is selected to allow the surgeon to operate under conditions of low-flow CPB or total circulatory arrest. Low pump flows (50 mL/kg/min) improve the operating conditions for the surgeon by providing a nearly bloodless field. DHCA allows the surgeon to remove the atrial or aortic cannula. If this technique is used, surgical repair is more precise because of the bloodless and cannula-free operative field. Arresting the circulation, even at deep hypothermic temperatures, introduces the concern of how well deep hypothermia preserves organ function, with the brain being at greatest risk. Three-region perfusion techniques may be an option to deep hypothermic CPB, but further studies are needed to assess feasibility and outcomes of this newer strategy.
Hemodilution
Hemodilution is used during CPB to decrease homologous blood use and improve microcirculatory flow by reducing blood viscosity during periods of hypothermia. Although hemoconcentrated blood has an improved O 2 -carrying capacity, its viscosity reduces efficient flow through the microcirculation. With hypothermic temperatures, blood viscosity increases significantly and flow decreases. Hypothermia, coupled with the nonpulsatile flow of CPB, impairs blood flow through the microcirculation. Blood sludging, small vessel occlusion, and multiple areas of tissue hypoperfusion may result. Therefore, hemodilution is an important consideration during hypothermic CPB.
The appropriate level of hemodilution for a given hypothermic temperature, however, is not well defined. Further, hemodilution reduces perfusion pressure; increases CBF, thereby potentially increasing the microembolic load to the brain; and reduces the O 2 -carrying capacity of blood. Using an animal model, one group of investigators found that extreme hemodilution to a hematocrit less than 10% resulted in inadequate O 2 delivery, but with higher hematocrit levels of 30%, there was improved cerebral recovery after DHCA. Jonas and colleagues confirmed these findings in a randomized trial using two hemodilution protocols (20% vs. 30% hematocrit) in infants younger than 9 months of age. In the short term, the group with lower hematocrit values had lower nadirs of cardiac index, higher serum lactate levels 1 hour after CPB, and a greater increase in total body water on the first postoperative day. At 1 year of age, mental development index scores were similar but psychomotor development index scores were significantly lower in the group with lower hematocrit values. Also, infants in this group had psychomotor development scores that were 2 standard deviations below the mean. Because RBCs serve as the major reservoir of O 2 during circulatory arrest, especially during rewarming, hematocrit values closer to 30% are generally preferred for deep hypothermia when this technique is contemplated. Currently, most centers maintain hematocrit levels approximately 25% to 30% during CPB, enhancing O 2 delivery to vital organs such as the brain. Cerebral O 2 delivery is an especially important consideration because cerebral autoregulation is impaired at deep hypothermic temperatures and after DHCA.
To achieve a hematocrit level of 25% to 30% in neonates and infants, banked blood should be added to the priming solution. The mixed hematocrit level on CPB (the hematocrit level of the total priming volume plus the patient’s blood volume) can be calculated by the following formula:
HctCBP=BVpt×HCTpt/BVpt+TPV
AddedRBCs(mL)=(BVpt+TPV)(Hctdesired)−(BVpt)(Hctpt)
where BV pt is the patient’s blood volume, TPV is the total priming volume, Hct desired is the desired hematocrit level on CPB, and Hct pt is the starting hematocrit level of the patient.
Like in adults, the optimal hematocrit level after weaning from CPB is not clear for pediatric patients. Decisions concerning post-CPB hematocrit levels are made based on the patient’s post-repair function and anatomy. Neonates, patients with residual hypoxemia, and those with moderate-to-severe myocardial dysfunction benefit from the improved O 2 -carrying capacity of hematocrit levels of 40% or higher. Patients with a physiologic correction and excellent myocardial function may tolerate hematocrit levels of 25% to 30%. In children with mild-to-moderate myocardial dysfunction, accepting hematocrit values between these levels seems prudent. Therefore, in patients with physiologic correction, moderately good ventricular function, and hemodynamic stability, the risks associated with blood and blood product transfusion should be strongly considered during the immediate postbypass period.
Blood Gas Management
The theoretic benefit of α-stat versus pH-stat blood gas management during hypothermic CPB has been a topic of great debate. Although the pH-stat strategy may not be optimal for adults in whom the principal risk for brain injury is microembolism, this risk is thought to be lower in infants because of the lack of atherosclerotic disease. With pH-stat management, the addition of CO 2 to the inspired gas mixture during cooling on CPB increases CBF and may improve cerebral tissue oxygenation and outcomes.
The controversial issue of pH management during CPB has been addressed in a large study from Boston Children’s Hospital. In this study, infants younger than 9 months of age were randomized to α-stat versus pH-stat during deep hypothermic CPB with excellent long-term follow-up. Neurodevelopmental outcomes were evaluated in infants undergoing biventricular repair for a variety of cardiac defects when younger than 9 months of age. The short-term benefits identified with the pH-stat strategy included a trend toward less postoperative morbidity and shorter recovery time to first electroencephalographic activity. In patients with TGA, there was a shorter duration of intubation and ICU stay in patients. However, the use of either the α-stat or pH-stat strategies was not consistently related to either improved or impaired neurodevelopmental outcomes at 2- and 4-year follow-up.
Initiation of Cardiopulmonary Bypass
Arterial and venous cannula placed in the heart before initiating CPB may result in significant problems in the peri-bypass period. A malpositioned venous cannula has the potential for vena cava obstruction. The problems of venous obstruction are magnified during CPB in the neonate because arterial pressures are normally low (20-40 mm Hg), and large, relatively stiff cannulas easily distort these very pliable venous vessels. A cannula in the inferior vena cava may obstruct venous return from the splanchnic bed, resulting in ascites from increased hydrostatic pressure or directly reduced perfusion pressure across the mesenteric, renal, and hepatic vascular beds. Significant renal, hepatic, and gastrointestinal dysfunction may ensue and should be anticipated in the young infant with unexplained ascites. Similar cannulation problems may result in superior vena cava obstruction. This condition may be more ominous during bypass. Under these circumstances, three problems may ensue: (1) cerebral edema, (2) a reduction in regional or global CBF, and (3) reduced proportion of pump flow reaching the cerebral circulation, causing inefficient brain cooling.
In the operating room, superior vena cava pressures via an internal jugular catheter should be monitored by examining the patient’s head for signs of suffusion after initiating bypass. Discussions with the perfusionist regarding adequacy of venous return and large cooling gradients between the upper and lower body should alert the anesthesiologist and the surgeon to potential venous cannulae problems. Patients with anomalies of the large systemic veins (persistent left superior vena cava or azygous continuation of an interrupted inferior vena cava) are at particular risk for problems with venous cannulation and drainage.
Problems with aortic cannula placement can occur. The aortic cannula may slip beyond the takeoff of the innominate artery, with blood therefore selectively flowing to the right side of the cerebral circulation. Also, the position of the tip of the cannula may promote preferential flow down the aorta or induce a Venturi effect to steal flow from the cerebral circulation. This problem has been confirmed during CBF monitoring by the appearance of large discrepancies in flow between the right and left hemispheres after initiating CPB. The presence of large aortic-to-pulmonary collaterals, such as a large PDA, also may divert blood to the pulmonary circulation from the systemic circulation, thereby reducing CBF and the efficiency of brain cooling during CPB. The surgeon should gain control of the ductus either before or immediately after instituting CPB to eliminate this problem and, if possible, large aortopulmonary collaterals should be embolized in the cardiac catheterization laboratory before the operative procedure. Neonates with significant aortic arch abnormalities (e.g., aortic atresia, interrupted aortic arch) may require radical modifications of cannulation techniques, such as placing the arterial cannula in the main pulmonary artery and temporarily occluding the branch pulmonary arteries to perfuse the body via the PDA or even dual arterial cannulation of both the ascending aorta and main pulmonary artery. Such adaptations require careful vigilance to ensure effective, thorough perfusion and cooling of vital organs.
Once the aortic and venous cannulas are positioned and connected to the arterial and venous limb of the extracorporeal circuit, bypass is initiated. The arterial pump is slowly started, and, once forward flow is ensured, venous blood is drained into the oxygenator. Pump flow rate is gradually increased until full circulatory support is achieved. If venous return is diminished, arterial line pressure is high, or mean arterial pressure is excessive, pump flow rates must be reduced. High line pressure and inadequate venous return are usually caused by malposition or kinking of the arterial and venous cannulae, respectively. The rate at which venous blood is drained from the patient is determined by the height difference between the patient and the oxygenator inlet and the diameter of the venous cannula and line tubing. Venous drainage can be increased by using vacuum-assisted drainage under certain circumstances.
In neonates and infants, deep hypothermia is commonly used. For this reason, the pump prime is kept cold (18°-22°C). When the cold perfusate contacts the myocardium during the institution of CPB, heart rate slows immediately and contraction is impaired. The contribution of total blood flow pumped by the infant’s heart rapidly diminishes. Therefore, to sustain adequate systemic perfusion at or near normothermic temperatures, the arterial pump must reach full flows quickly.
CPB is initiated in neonates and infants by beginning the arterial pump flow first. Once aortic flow is ensured, the venous line is unclamped and blood is siphoned out of the RA into the inlet of the oxygenator. Flowing before unclamping the venous line prevents the potential problem of exsanguination if aortic dissection or misplacement of the aortic cannula occurs. Neonates and infants have a low blood volume–to–priming volume ratio, and intravascular volume falls precipitously if the venous drainage precedes aortic inflow. Once the aortic cannula position is verified, pump flow rates are rapidly increased to maintain effective systemic perfusion. Because coronary artery disease is rarely a consideration, the myocardium should cool evenly unless distortion caused by the cannulas compromises the coronary arteries. When a cold prime is used, caution must be exercised in using the pump to infuse volume before initiating CPB. Infusion of cold perfusate may result in bradycardia and impaired cardiac contractility before the surgeon is prepared to initiate CPB.
Once CPB is initiated, appropriate circuit connections, myocardial perfusion, and optimal cardiac decompression should be confirmed. Ineffective venous drainage can rapidly result in ventricular distention. This is especially true in infants and neonates, in whom ventricular compliance is low and the heart is relatively intolerant of excessive preload augmentation. If ventricular distention occurs, pump flow must be reduced and the venous cannula repositioned. Alternatively, the heart may be decompressed by placing a cardiotomy suction catheter or small vent in the appropriate chamber.
Pump Flow Rates
Recommendations for optimal pump flow rates for children have historically been based both on the patient’s body mass and on evidence of efficient organ perfusion as determined by arterial blood gases, acid-base balance, and whole-body O 2 consumption during CPB. At hypothermic temperatures, metabolism is reduced, and CPB flow rates can therefore be reduced and still meet or exceed the tissue’s metabolic needs (see the discussion of low-flow CPB in the following section).
Special Techniques
Deep Hypothermic Circulatory Arrest
A certain subset of neonates, infants, and children with CHD require extensive repair of complex congenital heart defects using DHCA. This technique facilitates precise surgical repair under optimal conditions, with no blood or cannulae in the operative field and providing maximal organ protection and often resulting in shortened total CPB time. The scientific rationale for the use of deep hypothermic temperatures rests primarily on a temperature-mediated reduction of metabolism. Whole-body and cerebral O 2 consumption during induced hypothermia decreases the metabolic rate for O 2 by a factor of 2 to 2.5 for every 10°C reduction in temperature. These results are consistent with in-vitro models, which relate temperature reduction to a decrease in the rate constant of chemical reactions, as originally described by Arrhenius using the equation k = Ae − RT. The reduction in O 2 supply during deep hypothermic low-flow CPB is associated with preferential increases in vital organ perfusion (e.g., to the brain) and increased extraction of O 2 . Therefore, to some extent, deep hypothermic low-flow CPB exerts a protective effect by reducing the metabolic rate for O 2 , promoting preferential organ perfusion, and increasing tissue O 2 extraction.
The duration of the safe period for DHCA has not clearly been delineated. Although all organ systems are at risk for the development of ischemic and reperfusion injury, as manifested by lactate and pyruvate production during DHCA, the brain appears to be the most sensitive to and the least tolerant of these effects. Brainstem and cortical evoked potentials as well as processed electroencephalographs are altered after DHCA. The abnormalities in evoked potentials appear to be related to the duration of DHCA and are attributed to altered metabolism. During reperfusion after the arrest period, CBF and metabolism remain depressed in neonates and small infants ( Fig. 78.9 ; also see Fig. 78.6 ). Importantly, during the use of these extremes of temperature, autoregulation is lost and cerebral perfusion becomes highly dependent on the extracorporeal perfusion and presumably postbypass hemodynamic performance.

The potentially deleterious effects of prolonged DHCA in infants and neonates are well described. In general, it is agreed that very prolonged periods of uninterrupted DHCA may have adverse neurologic outcomes. However, considerable disagreement exists if a “safe” period of DHCA exists and whether patient-specific, procedure-specific, or postoperative management strategies may attenuate or promote CNS damage from DHCA. Cases have been reported of detrimental effects of DHCA on a variety of outcomes regarding the CNS, while others have described an inconsistent effect or no effect. Three issues have become clear over time: (1) the effects of short durations of DHCA are inconsistently related to adverse outcomes, (2) the effect of DHCA is not a linear phenomenon, and (3) the effects are most likely modified by other patient-related, preoperative, and postoperative factors. A large-scale study of 549 subjects undergoing the Norwood stage 1 procedure with DHCA found duration of greater than 45 minutes to be a risk factor for 30-day mortality.
Regional Cerebral Perfusion
Some surgeons have developed innovative and challenging strategies to provide continuous cerebral perfusion during complex reconstruction of the aortic arch or intracardiac repair to avoid or minimize the use of DHCA. However, avoiding DHCA, the duration of CPB is necessarily lengthened, and longer durations of CPB have been shown to adversely affect both short- and long-term outcomes. The relative risks and benefits of longer CPB versus less (or no) DHCA remain a subject of continued controversy. In efforts to study this newer strategy, two recent studies have evaluated the technique of regional cerebral perfusion. In one non-randomized study, Wypij and colleagues followed 29 infants who underwent a stage 1 palliation, 9 of whom received regional cerebral perfusion at 30 to 40 mL/kg/min. The authors reported no difference in mental or psychomotor developmental indices at 1 year of age between the regional cerebral perfusion group and those who received DHCA as a primary strategy. A larger randomized trial of DHCA with or without regional cerebral perfusion at 20 mL/kg/min in patients with a functional single ventricle included 77 patients with similar survival to hospital discharge (88%) and at 1-year follow-up (75%). No significant difference was seen in either the psychomotor development index or the mental development index scores between the two groups at any time points, although the scores tended to be lower in the regional cerebral perfusion group.
A further innovation to the previously described technique is a three-region perfusion strategy for aortic arch reconstruction in the Norwood procedure. This strategy involves direct perfusion of the coronaries via a proximal aortic cannula, splanchnic beds via a distal thoracic aorta cannula, and cerebral perfusion via an innominate cannula. The arch repair occurs from distal to proximal at warmer patient temperatures and with a beating heart. This theoretically provides the potential for decreased coronary and splanchnic ischemic times, decreasing the risk of cardiac dysfunction and abdominal organ damage, and mitigating the negative hypothermic effects on the hematological system. Larger, long-term studies are needed to assess the efficacy of this technique in improving cardiovascular, renal, and other outcomes.
Glucose Regulation
The detrimental effects of hyperglycemia during complete, incomplete, and focal cerebral ischemia are well demonstrated. The role of glucose in potentiating cerebral injury appears to rest on two factors: adenosine triphosphate (ATP) usage and lactic acidosis. The anaerobic metabolism of glucose requires phosphorylation and the expenditure of two molecules of ATP before ATP production can occur. This initial ATP expenditure may result in a rapid depletion of ATP and may explain why hyperglycemia worsens neurologic injury. Lactic acidosis is also important in glucose-augmented cerebral injury, though its role may be as a glycolytic enzyme inhibitor: lactate slows anaerobic ATP production by inhibiting glycolysis immediately after ATP is consumed in the phosphorylation of glucose.
Although the detrimental effects of hyperglycemia during ischemia are clear, very little evidence supports a relationship between a worsening neurologic outcome and hyperglycemia during CPB or DHCA in children. A review of acquired neurologic lesions in patients undergoing the Norwood stage 1 procedure for HLHS suggested hyperglycemia as a significant associated finding in patients with extensive cerebral necrosis or intraventricular hemorrhage. A host of other potentially damaging factors (e.g., periods of hypoxia, low diastolic and systolic pressure, thrombocytopenia) were statistically associated with the observed neuropathology. Whether glucose directly contributes to neurologic injury or merely serves as a marker for a high-risk population that ultimately suffers neurologic insult as a result of other factors is not clear.
Hypoglycemia is also a frequent concern in neonates during the perioperative period. Reduced hepatic gluconeogenesis coupled with decreased glycogen stores places the newborn at increased risk for hypoglycemic events. In newborns with CHD, reduced systemic perfusion (e.g., critical coarctation, HLHS, critical aortic stenosis) may result in worsening hepatic biosynthesis, further impairing glucose production. These patients may be fully dependent on exogenous glucose; therefore, it is not uncommon for them to require 20% to 30% dextrose infusions to maintain euglycemia in the prebypass period. Older children are not immune to hypoglycemic events and are therefore susceptible to hypoglycemia-induced neurologic injury. Patients with low cardiac output states (cardiomyopathies, pre-transplant patients, critically ill postoperative patients) requiring reoperation and when on substantial inotropic support are at high risk for reduced glycogen stores and intraoperative hypoglycemia.
The impact of hypoglycemia during CPB is further complicated by the consequences of hypothermia, CO 2 management, and other factors that may modify normal cerebrovascular responses during bypass. In a dog model, insulin-induced hypoglycemia to 30 mg/dL did not alter the electroencephalographic findings. However, after 10 minutes of hypocapnic hypoglycemia, the electroencephalogram became flat. The loss of electroencephalographic activity from hypoglycemia alone does not normally occur above glucose levels of 8 mg/dL.
During deep hypothermic CPB and DHCA, CBF and metabolism are altered. The additive effect of hypoglycemia, even if mild, may cause alterations in cerebral autoregulation and culminate in increased cortical injury. The common practice of using hyperventilation to reduce PVR in neonates and infants during weaning from CPB and in the early postbypass period can further exacerbate hypoglycemic injury. Glucose monitoring and rigid maintenance of euglycemia are an important part of CPB management in the patient with CHD.
Renal Effects
After CPB, the combined effects of hypothermia, nonpulsatile perfusion, and reduced mean arterial pressure cause release of angiotensin, renin, catecholamines, and antidiuretic hormones. These circulating hormones promote renal vasoconstriction and reduce renal blood flow. However, despite the negative impact of CPB on renal function, low-flow, low-pressure, nonpulsatile perfusion has not been linked with postoperative renal dysfunction ( Table 78.9 ). The factors that best correlate with postoperative renal dysfunction are preoperative renal dysfunction and profound reductions in post-CPB cardiac output. Preoperative factors include primary renal disease, low cardiac output, and dye-related renal injury after cardiac catheterization.
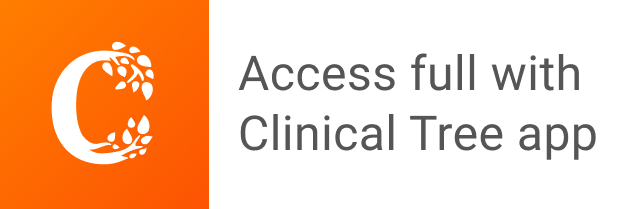