Acute respiratory distress syndrome (ARDS) is a diffuse inflammatory reaction of the lung to an insult and is characterized by increased pulmonary capillary permeability, lung edema, and atelectasis. The histological hallmark of ARDS is diffuse alveolar damage and its pathognomonic hyaline membranes. The associated radiographic feature is bilateral alveolar infiltrates.
The Berlin definition of ARDS published in 20121,2 has superseded the prior American-European Consensus Conference definition of 1994.3 The Berlin criteria updated the diagnostic requirements for ARDS, classified its severity based on the ratio of the arterial pressure of oxygen (PaO2) to the fraction of inspired oxygen (FiO2) (P/F ratio), and eliminated the term acute lung injury. The diagnostic criteria mandate that all of the following conditions are met before the syndrome is diagnosed: (1) In patients without known ARDS risk factors, cardiogenic or hydrostatic pulmonary edema as well as other alternative causes of bilateral pulmonary infiltrates need to be excluded; patients with a known ARDS risk factor are no longer required to undergo echocardiography or right heart catheterization to rule out cardiogenic pulmonary edema; (2) respiratory symptoms are of acute onset or are worsening significantly within 1 week prior to diagnosis; (3) imaging (either chest x-ray or computed tomography [CT]) shows bilateral alveolar infiltrates; and (4) oxygenation is impaired, the degree of which determines ARDS severity: P/F ratio greater than 200 but less than or equal to 300 defines mild, greater than 100 but less than or equal to 200 defines moderate, and 100 or less defines severe ARDS.
The incidence of ARDS is estimated to be approximately 86 cases per 100,000 person-years, which results in about 190,000 cases in the US every year.4 Both incidence and mortality are comparable to those of acute myocardial infarction.5
Acute respiratory distress syndrome is triggered by predisposing factors, with more than 60 possible insults having been identified. In clinical practice, the vast majority of cases are caused by pulmonary or extrapulmonary sepsis, aspiration, severe trauma or burns, or transfusion or drug reactions, or as a complication of hematopoietic stem cell transplantation.6,7
The pathobiology and pathophysiology of the syndrome are complex and evolving, and as a result are beyond the scope of this chapter. The reader is referred to several recent informative and well-written review articles on this topic.8-10
It has been recognized for decades that mechanical ventilation is capable of causing or exacerbating lung pathology through mechanisms known collectively as ventilator-induced lung injury (VILI).11 Gross barotrauma in the form of pneumothorax is the most clinically obvious example of VILI. On a microscopic level, multiple experimental models have demonstrated that ventilation with excessive tidal volumes, so-called volutrauma, produces inflammation and changes indicative of high-permeability pulmonary edema even in healthy lungs.12 Structural distortion caused by injurious ventilation inflicts damage on lung epithelial cells as well as their cytoskeletal framework and, by extension, on the extracellular matrix. This mechanical cascade is then converted to chemical signals through the release of pro-inflammatory cytokines, which promote pulmonary and extrapulmonary organ dysfunction. The adverse inflammatory response triggered by such damaging ventilation is termed biotrauma.13
Concurrently, pioneering CT examinations of the lungs of ARDS patients revealed that the aerated lung volume in these patients is significantly smaller than that predicted by their body frame, leading to the adoption of the term “baby lung” when referring to this discrepancy.14 Contrary to the diffuse infiltration apparent on plain radiography, on CT, the majority of patients exhibited lower-lobe predominant or patchy distribution of increased density.15 Whether the opacities present on CT represent edema or compressive atelectasis remains a subject of debate,16,17 but what seems clear is that there is great inhomogeneity in the degree of aeration among lung units in ARDS patients.18 Substantiating the baby lung hypothesis is the finding that although the small functional size of the ARDS lung translates to a decreased overall compliance (Δ volume/Δ pressure), the specific compliance (ie, compliance normalized for lung volume) is similar to that of healthy lung.19 From this concept, it follows that the administration of conventional tidal volumes to the small aerated component of the ARDS lung would result in excessive lung deformation or strain (tidal volume/initial lung volume) with each ventilator breath (ie, volutrauma), thereby risking barotrauma because of excessive force per unit of cross-sectional area, defined as stress and approximated by the following equation: Transalveolar Pressure (PL) = Alveolar Pressure (PA) – pleural pressure (Ppl). Under conditions of zero flow, the end-inspiratory airway pressure or plateau pressure (Pplat) is assumed to represent PA. The demonstration that healthy lungs incur VILI at a much higher strain and resultant stress than damaged lungs do has been suggested as evidence of so-called “stress raisers” in the ARDS lung.20,21 The now-classic experimental model proposed by Mead et al showed that the stress at the interface of open and collapsed spheres is significantly greater than the overall distending pressure applied to the structure containing those spheres.22 This situation is theorized to correspond to the boundary between inhomogeneous alveoli of ARDS, thus predisposing the nonuniform baby lung to VILI. In fact, the normal specific compliance of the aerated lung zones does not necessarily imply intact structure and function as demonstrated by nuclear isotope studies showing increased extravascular lung water in those regions.23
The conceptual framework described above prompted a number of investigators in the late 1990s to compare ventilation with lower versus higher tidal volumes (TV) in ARDS patients, and the results were mixed. A notable proof-of-concept study showing benefit was that by Amato et al, in which a 28-day mortality reduction was seen in 29 subjects randomized to lung-protective ventilation with low tidal volumes (tolerating “permissive” hypercapnia) and higher positive end-expiratory pressure (PEEP) levels as compared to 24 conventionally ventilated controls.24 The incidence of gross barotrauma was significantly reduced in the protective ventilation group despite higher PEEP, which was set based on the lower inflection point of the pressure-volume curve. Other investigators found a favorable effect of low TV ventilation on both lung and systemic proinflammatory cytokine levels in ARDS patients.25 These findings paved the way for the ARDS Network’s (ARDSNet) landmark ARMA multicenter randomized, controlled trial (RCT) of low (6 mL/kg predicted body weight [PBW]) versus high (12 mL/kg) TV ventilation in over 800 subjects with ARDS.26 In the low-tidal volume (LTV) arm, a goal Pplat of 30 cm H2O or less was targeted to limit stress in addition to limiting strain. Positive end-expiratory pressure was set according to a stepwise PEEP/FiO2 oxygenation table. Significantly fewer patients in the LTV group died on the ventilator prior to hospital discharge (absolute risk reduction ~ 9%), which was the primary endpoint, despite lower mean PEEP/FiO2 ratios early in their clinical course. Permissive hypercapnia was evident and well tolerated. In fact, it recently has been shown to have a potential attenuating effect on VILI in its own right.27 Subsequent RCTs, real-world observational studies, and meta-analyses upheld the mortality benefit of LTV ventilation.28-30 A later analysis demonstrated that survival improves with the LTV strategy even in quartiles of Pplat below 30 cm H2O, further emphasizing the importance of strain reduction even when stress is within traditionally accepted boundaries.31,32 Additionally, several studies have shown that Pplat may be a suboptimal surrogate for lung stress and one for which a ceiling of 30 cm H2O may not be universally applicable in ARDS ventilation.33-35 It is worth recalling that Pplat is only 1 of the 2 components of the PL calculation, which is the difference between Pplat and Ppl. Thus, the same Pplat may translate into very different magnitudes of stress depending on the Ppl, which could be much higher than average in patients with elevated chest wall elastance (reciprocal of compliance) and therefore Ppl (eg, morbid obesity, anasarca, chest wall trauma), and lower than average in patients with spontaneous respiratory effort. While it is not practical to measure pleural pressure directly at the bedside of ARDS patients, an estimate can be obtained using a balloon catheter to transduce an esophageal pressure (Pes), itself a flawed technique that has not reached widespread adoption. Even if the Pplat were an accurate estimate of PL in a given patient, the actual stress on a tissue level may be higher than expected, especially in severe cases manifesting the highest degree of heterogeneity, and thereby stress amplification, as discussed above.18 On the other end of the spectrum, low airway pressures carry their own price of potential recruitment failure if the high opening pressures of diseased ARDS lung units are not attained, though increasing the PEEP could mitigate the harm and in fact improve outcomes through lower driving pressure.36
An analogous caveat applies to the generalization that a TV of 6 mL/kg is associated with safe strain in all ARDS cases. Although it is adjusted for the patient’s body frame with the use of predicted body weight (PBW), it does not take into account the patient’s abnormal resting lung volume. This is significant because the same TV therefore could produce protective or injurious strain, depending on the size of that patient’s baby lung. Terragni et al showed that even at a TV of 6 mL/kg PBW, many ARDS patients exhibit CT evidence of tidal hyperinflation, and it is more pronounced in those with a smaller aerated lung compartment.37
Interest in the so-called driving pressure (Pplat-PEEP) reflects the desire to refine the generic TV and Pplat criteria that have characterized lung protective ventilation strategies inspired by the ARMA trial. The appeal of this idea becomes apparent after rearrangement of the formula for the static compliance (Crs) of the respiratory system (Crs = TV / [Pplat – PEEP]) to solve for driving pressure, which yields the quotient TV/Crs and can thus be considered a normalization of TV for compliance, which in ARDS is a function of the size of the baby lung. In a recent retrospective analysis of individual patient data from multiple randomized controlled trials of ARDS, Amato et al demonstrated that driving pressure predicted outcome independent of Pplat but that the converse is not true.38 Additionally, these investigators showed that the benefit of LTV ventilation in those trials was mediated by the concurrent reduction in driving pressure rather than the assignment to the LTV arm per se. Although driving pressure has since been shown to correlate reasonably well with lung stress, it is still not universally used.39
Cressoni et al have shown in an animal model that VILI develops if the mechanical power—that is, the breathing work performed by the ventilator per unit of time—applied to the lungs during mechanical ventilation is exceedingly high.40 Driving pressure, through its interconnection with respiratory system elastance, is one of the determinants of power in an exponential manner.41 Other components of power, namely TV, inspiratory flow rate, and respiratory frequency, likewise have a direct logarithmic relationship with it. The role of TV in the development of VILI has been discussed earlier, but it is noteworthy that a contribution of both inspiratory flow and respiratory rate to VILI has also been proposed.42,43 The concept of power is intriguing because of the potential that it represents the all-encompassing link between ventilator parameters and VILI in ARDS.44
The 2017 American Thoracic Society (ATS)/European Society for Intensive Care Medicine (ESICM)/Society of Critical Care Medicine (SCCM) Guidelines for Mechanical Ventilation in ARDS make a strong recommendation in favor of LTV ventilation, initially set at 6 mL/kg PBW (range 4–8 mL/kg), while targeting a plateau pressure of less than 30 cm H2O.45 This is a subtle departure from the ARDSNet recommendation of initially setting the TV at 8 mL/kg PBW and titrating down to 6 mL/kg46 (Fig. 11-1).
Concurrently with the realization that mechanical ventilation of healthy animal lungs with TVs above a certain threshold leads to pulmonary edema came the observation that VILI is attenuated by the application of PEEP.47 It was subsequently shown that PEEP blunts the pro-inflammatory cytokine release associated with high-TV ventilation and reduces the histological stigmata of high-permeability edema.48,49 These findings gave rise to the notion that cyclical opening and closing of inflamed, surfactant-depleted lung units—whether damaged by harmful ventilation themselves or by an antecedent insult—further propagates the injury by means of a type of VILI called atelectrauma. Additionally, by prevention of end-expiratory alveolar collapse, the application of PEEP can increase the number of lung units receiving the TV during the subsequent inspiration—a phenomenon known as recruitment—and thereby enlarge the size of the baby lung, making it less likely to undergo excessive strain (volutrauma). Recruitment also accounts for the improvement in oxygenation produced by PEEP thanks to the salutary effect on ventilation/perfusion (V̇/Q̇) matching that results from reduced shunting past nonparticipating alveoli.50 Rounding out the central role of PEEP in VILI prevention is the greater lung homogeneity that it creates via recruitment, which can offset the stress amplification that occurs at interfaces of well-aerated and poorly aerated alveoli.
Along with the theoretical benefits of PEEP, its potential drawbacks must also be recognized. Computed tomography studies have demonstrated that usually only a fraction of the ARDS lung consists of readily recruitable alveoli.51,52 In the case of the minimally recruitable patient, the PEEP-induced increase in end-expiratory lung volume risks disproportionally affecting the aerated lung zones, making them prone to overdistention (ie, excessive strain) and barotrauma during the inspiratory phase.53 Hyperinflation and a proinflammatory cytokine profile were observed despite low TVs when the ARDSNet stepwise table was used to set PEEP as in the ARMA trial.54 This is particularly salient in light of recent animal data suggesting a greater inflammatory response in the lung subjected to volutrauma as compared to atelectrauma.55 Additionally, PEEP can raise intrathoracic pressure sufficiently to cause a reduction in venous return and therefore ventricular preload, which could create or exacerbate hemodynamic compromise. In fact, if the decrease in cardiac output related to PEEP is significant enough, it could lead a drop in oxygen delivery despite the favorable impact of PEEP on the oxygen content of arterial blood.56
When higher PEEP levels were incorporated into a so-called “open lung” approach in comparison to non–lung-protective strategies, survival favored the groups receiving higher PEEP.24,28 Subsequently, when the control arm was ventilated according to the ARDSNet protocol from ARMA, a mortality difference was no longer seen.57 Higher versus lower PEEP settings as isolated interventions have been the subject of 3 multicenter randomized controlled trials (ALVEOLI 2004,58 LOVS 2008,59 Express 200860) and a patient-level meta-analysis (PLMA)61 combining data from these 3 studies, which have a number of features in common. They were conducted in the post-ARMA era of LTV ventilation in ARDS, and the mean P/F ratio was less than 200 in all the study groups. Positive end-expiratory pressure was titrated according to a stepwise PEEP/FiO2 oxygenation table analogous to the ARDSNet protocol in ALVEOLI and LOVS and maximized until reaching a Pplat ceiling of 28 to 30 cm H2O in Express. Statistically significant separation in average PEEP values was achieved between the control and experimental groups in all 3 trials, though reaching the target difference in ALVEOLI required an in-study protocol adjustment. Each trial had a mortality-based endpoint as its primary outcome measure, which did not reach statistical significance in any of them. Oxygenation was better in the higher PEEP arm of all 3 studies, and improvements in other outcomes (eg, LOVS: death with refractory hypoxemia; Express: ventilator-free days, organ failure–free days) did reach statistical significance in favor of higher PEEP. From a safety perspective, higher PEEP did not increase the incidence of gross barotrauma; while it did increase the need for intravenous fluid administration, vasopressor use did not differ between the groups. The subsequent meta-analysis largely recapitulated the results of the individual trials, except that in the population with worse gas exchange (P/F ratio < 200; ie, ARDS by the American-European Consensus Conference definition) there was a reduction in hospital mortality of borderline statistical significance (adjusted risk ratio [RR], 0.90; 95% confidence interval [CI], 0.81–1.00).
In the ensuing years, there has been much speculation about the reasons why the convincing theory and experimental evidence behind the lung protective properties of PEEP has not translated into a clear-cut survival benefit in clinical trials. Among the hypotheses are the variability in ventilator settings at the time of application of ARDS criteria for enrollment and the lack of consistency in regard to recruitment maneuvers (RMs) (see “Nontraditional Mechanical Ventilation Modes”).57 Furthermore, as suggested by the meta-analysis, a possible benefit to the patients with the most severe ARDS may have been masked by harm to those with milder disease. This notion is supported by CT data indicating that ARDS patients with the highest potential for recruitment are also those with the most lung involvement, the worst oxygenation, and greater mortality.40 When the data from LOVS and Express were retrospectively analyzed, it was found that an improvement in oxygenation in response to raising PEEP—a marker of underlying recruitable lung—was associated with a lower risk of death.62
Perhaps the most controversial clinical question to emerge from this debate is whether the generic PEEP/FiO2 table based entirely on oxygenation, as in ALVEOLI and LOVS, or the arbitrary Pplat-guided algorithm used in Express is the optimal approach to PEEP titration. To date, consensus on the best strategy for setting PEEP remains elusive, but there are many who favor basing the level on an individual patient’s physiological parameters. Pointing to the success of the trials by Amato et al24 and Villar et al,28 proponents have advocated determining the lower inflection point of the pressure-volume (P-V) curve and setting the PEEP at a fixed point above that value to prevent deflation into the zone of derecruitment. In a different take on the same concept, Talmor et al compared Pes-guided PEEP settings to the ARDSNet table as a control, targeting PEEP levels that would yield a positive transalveolar pressure (PEEP-Ppl) at end-expiration, with Pes serving as a surrogate for Ppl.33 Keeping the difference between PEEP and Ppl positive in principle ought to prevent end-expiratory derecruitment and minimize atelectrauma. In that study of 61 patients, despite higher average PEEP and P/F ratio in the Pes-guided arm, unadjusted mortality was no different in the 2 groups. A much larger multicenter EPVent-2 trial (Clinicaltrial.gov identifier NCT01681225) is further exploring this approach.
In clinical practice, constructing P-V curves and measuring Pes can be cumbersome tasks, and there is experimental evidence that recruitment (and consequently derecruitment) occurs along a continuum of pressure rather than as a threshold, all-or-nothing phenomenon demarcated by an abrupt P-V slope change.63,64 Grasso et al have proposed the so-called stress index as an alternative, which is a measurement reflecting the behavior of the pressure-time (P-T) waveform during constant flow, volume-targeted ventilation.65 A stress index less than 1 corresponds to a downward concavity of the curve, an indicator that effective elastance (the inverse of effective compliance) is decreasing with progressive inflation due to ongoing recruitment and increase in the size of the baby lung. Such a patient would be considered to have the potential for additional recruitment, and PEEP would be raised accordingly. On the opposite extreme is a stress index greater than 1, represented by an upward concavity in the P-T curve, that is indicative of a dynamically increasing elastance caused by overdistention. In this case, the PEEP would be reduced. The goal would be a linear P-T curve, signifying a constant elastance throughout TV delivery and therefore achievement of maximal possible recruitment without hyperinflation.
A study by Chiumello et al compares the ability of the above approaches to appropriately stratify the magnitude of PEEP according to the severity of ARDS.66 Ironically, only the PEEP/FiO2 table method ramped up the PEEP level in an incremental fashion across groups with mild, moderate, and severe ARDS.
The 2017 ATS/ESICM/SCCM Guidelines for Mechanical Ventilation in ARDS contain a conditional recommendation in favor of higher PEEP as opposed to lower PEEP for moderate or severe ARDS based on the mortality benefit in this subgroup, suggested by the PLMA discussed above.45
The use of unconventional modes of mechanical ventilation in ARDS has generally reflected the desire to achieve maximal safe mean airway pressure (mPaw) in order to, in turn, maximize inspiratory alveolar recruitment and, thereby, oxygenation. A classic example of such a strategy is inverse ratio ventilation (IRV), in which inspiratory time is set to exceed expiratory time, an unnatural respiratory pattern requiring deep sedation and neuromuscular paralysis. Inverse ratio ventilation fell out of favor when it was recognized that the oxygenation benefit was likely related to intrinsic PEEP from the short expiratory time rather than to the high mPaw, per se.67 Airway pressure release ventilation (APRV) is a modification of the IRV concept that was first described about 30 years ago. It is characterized by cyclical inflations to a set peak airway pressure (Phigh) separated by deflations to a much lower pressure (Plow) analogous to PEEP, but in practice frequently set to 0 cm H2O. The duration of both Phigh (Thigh) and Plow (Tlow) is determined by the clinician and programmed such that Thigh greater than Tlow to augment mPaw. Carbon dioxide elimination occurs during the deflations and is proportional to their frequency and the accompanying pressure drop (ie, Phigh – Plow). The unique feature of APRV in comparison to conventional IRV is that spontaneous patient breathing can be superimposed on ventilator activity at any point in the cycle. These efforts may or may not be pressure supported and, notably, they can have an additive effect on the TV generated by the ventilator. It is also important to recognize that without spontaneous respiration, APRV becomes indistinguishable from pressure-controlled IRV. Investigators have been able to demonstrate in animal68 and human69 studies that spontaneous breathing during APRV in ARDS leads to superior recruitment of dependent lung zones and V̇/Q̇ matching compared to APRV without spontaneous breathing. A recent observational study70 showed a salutary effect of APRV on oxygenation, a parameter of questionable prognostic significance in ARDS, whereas a contemporary small prospective RCT71 found no difference in ventilator-free days or mortality between APRV and synchronized intermittent mechanical ventilation. Robust clinical trial data supporting or refuting the utility of APRV in ARDS in the era of lung protective ventilation are not available.72 On theoretical grounds, concerns about the use of this mode are primarily 2-fold. One concern is that control over the TV is not straightforward, especially when spontaneous breathing is present, and so violations of the LTV strategy are likely in clinical practice.73 Average TVs in excess of 9 mL/kg PBW have been encountered in retrospective series of APRV use in the LTV era.70,74 Secondly, the demonstration by Papazian et al of a survival benefit of early neuromuscular blockade in severe ARDS75 has spurred inquiry into whether active patient effort is a contributor to lung injury and therefore mortality in ARDS.76 In an animal model, forceful spontaneous breathing was injurious when coupled with moderate TVs (7–9 mL/kg) and protective in combination with low TVs (6 mL/kg).77 Proposed mechanisms for harm include large swings in PL caused by drops in Ppl from vigorous negative-pressure efforts, as well as increased pendelluft), both of which are likely to be exacerbated by larger TVs.78 Thus, the potentially liberal TVs associated with APRV, together with the permitted spontaneous breathing could act synergistically to exacerbate VILI in ARDS patients ventilated with this mode.
Another unconventional approach to protective, “open-lung” ventilation in ARDS is high-frequency oscillatory ventilation (HFOV), in which a fundamentally different mechanical ventilator pumps small, nonphysiologic TVs into the trachea at a rate that can exceed 300 times per minute. The oscillator continually pushes a column of gas down into the lower airways and then back up again and out through an outflow valve. The individual TVs are smaller than the anatomical dead space, yet carbon dioxide elimination takes place effectively, so gas exchange is believed to occur by a number of unique mechanisms.79 One of them is pendelluft, which refers to the swinging back and forth of gas between adjacent lung units differing in their resistance and compliance (ie, having different time constants).80 Other phenomena at play include collateral ventilation and turbulent flow facilitating diffusion (so-called Taylor dispersion).81 Whereas the ultra-low TVs in theory minimize lung strain and thus risk of volutrauma, small-amplitude oscillations about a high mPaw are thought to protect against both excessive stress and cyclical derecruitment. This unnatural mode of ventilation also presents practical challenges such as poor patient tolerance without paralysis and inadequate cardiac filling due to maintenance of a high intrathoracic pressure.82 Not surprisingly, multiple animal experiments comparing VILI induced by HFOV to that of nonprotective conventional ventilation (CV) favored the former;83 a subsequent comparison to protective CV yielded no difference in clinically measurable parameters.84 Until recently, human clinical studies on the performance of HFOV in ARDS were limited to observational experience85 and 2 flawed RCTs in which CV was not protective.86,87 In aggregate, these data suggested an oxygenation benefit without an impact on mortality; a systematic review combining heterogeneous adult and pediatric studies available prior to 2010 resulted in a small survival advantage for HFOV that prompted further investigation.88 Answers emerged in 2013 with the simultaneous publication of 2 multi-center RCTs, OSCILLATE89 and OSCAR90 comparing HFOV and CV that together enrolled nearly 1000 ARDS patients in the LTV era. OSCILLATE was halted prematurely due to significantly higher mortality in the HFOV arm (47% vs. 35%), and OSCAR showed no survival difference. High-frequency oscillatory ventilation reduced the incidence of refractory hypoxemia in OSCILLATE but had no effect on death following such episodes. No significant difference in oxygenation was observed in OSCAR. Although both trials have been subjected to criticism ranging from lack of experience with HFOV in many centers to suboptimal HFOV settings,91 a number of subsequent meta-analyses have been performed,92-94 and none has demonstrated improved survival with HFOV in ARDS contrary to the original systematic review. A major prevailing conclusion stemming from OSCILLATE and OSCAR is that HFOV offers no incremental benefit over CV in ARDS when the latter conforms to current standards of lung protection.95
The reason why the inspiratory and expiratory limbs of the lung’s P-V curve are not superimposed is the presence of hysteresis, which is a property whereby the preceding inflation phase imparts a greater compliance to lung tissue for the subsequent deflation. This phenomenon results in a bigger lung volume associated with a given pressure on the expiratory limb compared to the inspiratory limb of the P-V curve. Theoretically, if the ARDS lung were to undergo an initial maximal tolerated inflation followed by the application of sufficient PEEP to prevent deflation back to the inspiratory limb, it would retain the higher aerated volume, and that would become the new, larger size of the baby lung. The expected benefit would then be improved oxygenation and lower risk of VILI. This is the principle behind so-called lung RMs: brief applications of a pressure that would be injurious if used consistently in order to achieve maximal alveolar opening in seconds to minutes and then consolidate the gains by raising the PEEP above the pre-RM level. An example of a commonly used RM is the application of a continuous positive airway pressure (CPAP) of 40 cm H2O for 40 seconds in a passive patient.96 A considerably longer version is the stepwise escalation of PEEP until a certain airway pressure ceiling is reached, followed by de-escalation from the peak to the new maintenance PEEP.97 Based on animal experiments98 and human CT data,49,50 the latter approach may be more effective in opening the lung, while being less destructive. In the course of the sustained high CPAP, it has been shown that further recruitment is minimal after the first 10 seconds, beyond which the pressurized thorax begins to impact hemodynamics in the susceptible patient.99 Despite the attractive physiological basis underlying RMs, the demonstration of improvement in gas exchange has been inconsistent at best.100-104 As expected, failure to adjust the PEEP upward following the RM leads to rapid dissipation of any oxygenation gains.105 Additionally, use of RMs later in the course of ARDS has been associated with failure to respond, probably because of progression to a more organized form of alveolar damage. Disappointing oxygenation data, however, do not necessarily negate a favorable impact of RMs on VILI, which could have survival implications. Markers of epithelial injury and inflammation have, in fact, been shown to decrease in ARDS subjects after an RM.106,107 Perhaps the most challenging question surrounding RMs is whether they affect ARDS mortality, in part because most trials have studied them as part of a multifaceted ventilation strategy.24,59,107 Studies in which RMs are an isolated variable are few and offer conflicting results.94,95 Between the 2 most recent meta-analyses of RCTs, 1 showed lower hospital mortality,108 while another showed lower ICU mortality but not hospital mortality.109 When the available data on hypoxemia were pooled, the 2 meta-analyses diverged. These reviews are handicapped by reliance on heterogeneous studies prone to bias. The totality of the evidence behind RMs in ARDS leaves room for provider discretion based on clinical circumstances; although, for both oxygenation and VILI reduction, delays in RM performance are likely to diminish utility.
The 2017 ATS/ESICM/SCCM Guidelines for Mechanical Ventilation in ARDS include a conditional recommendation in favor of RMs, with caution advised in patients who are hypovolemic or in shock.45
The observation that oxygenation can be improved by turning the patient with acute respiratory failure from the usual supine position (SP) to a prone position (PP) is not novel,110 but only recently has PP joined the short list of ARDS interventions associated with a mortality benefit in an RCT.111 Much of the research on this topic has focused on potential explanations for the salutary effect of PP on gas exchange. What has emerged is that the previously dependent degassed dorsal lung regions regain aeration in the PP, while the newly dependent ventral lung regions lose aeration.112,113 The aggregate volume of well-aerated alveoli thereby increases due to the relatively larger dimensions of the dorsally oriented lung and the relief of pressure from the abdominal cavity and the heart in the PP.114,115 Because of resultant improvement in lung geometry, the baby lung becomes not only functionally bigger but also more homogeneously inflated within the chest, thus attenuating the anteroposterior ventilation gradient normally present in the SP in ARDS.113 In that regard, PP can be considered analogous to an RM when combined with high PEEP.116 Concurrent gravitational redistribution of perfusion also occurs, but there is enough preservation of nondependent perfusion117 such that V̇/Q̇ uniformity increases in the PP relative to the SP.118 This mechanism of improved V̇/Q̇ matching is believed to underlie the oxygenation benefit of PP, which has been consistently present across animal and human studies.
As has been the case with PEEP and many other ARDS interventions, however, it has proven much more difficult to demonstrate a mortality reduction. None of the 4 initial RCTs119-122 published between 2001 and 2009 showed an improvement in survival with PP, whether conducted in the LTV era or not, though lessons learned from them suggested that the benefits of this strategy may be maximal when subjects with the most severe ARDS are proned early and for an extended period of time. In 2 of the trials,120,122 there was a significantly greater incidence of adverse events in the PP such as pressure ulcers and malpositioning of hardware. With this as background, Guérin and his experienced coinvestigators conducted the PROSEVA trial111 enrolling 466 ARDS patients with a P/F ratio less than 150 mmHg within 36 hours of initiation of mechanical ventilation and within an hour of randomization. Patients were in a prone position for an average of 17 h/d. There was strict adherence to lung protective ventilation protocols and extensive use of neuromuscular blocking agents (NMBA). Mortality at 28 and 90 days was significantly lower in the PP arm, accompanied by an increase in ventilator-free days and with no difference in complications. Notably, a post-hoc analysis determined that although, as expected, PP increased the P/F ratio in PROSEVA, the effect on oxygenation could not account for the survival benefit.123 This conclusion has prompted a closer look at the impact of PP on VILI reduction, which, as discussed, may be the thread linking all favorable ARDS interventions. In principle, the greater homogeneity of the baby lung afforded by PP should attenuate stress amplification while the greater overall aerated volume should reduce strain. These hypotheses are supported by animal studies of PP,124,125 which, along with scant human data,116,126 also demonstrate that PP mitigates against lung damage and inflammation caused by injurious ventilation.127 In fact, PP may require synergy with lung protective ventilation to improve outcomes in ARDS as suggested by a post-PROSEVA meta-analysis that showed a mortality benefit only when analysis was restricted to trials employing LTV.128 Another prism through which the role of PP in ARDS has been examined is that of cardiac performance, specifically as it relates to the right ventricle, whose function can be augmented by a PP-induced increase in preload and decrease in afterload.129,130 Such optimization could play an important role in the clinical course of patients with ARDS complicated by acute cor pulmonale.
The 2017 ATS/ESICM/SCCM Guidelines for Mechanical Ventilation in ARDS contain a strong recommendation in favor of prone positioning for more than 12 h/d for severe ARDS.45
Endogenous human corticosteroids (CS), cortisol being primary among them, are potent but nonspecific inhibitors of inflammation. They exert their effects in myriad ways, most notably by blocking the activity of a transcription factor known as nuclear factor-κB, which promotes the synthesis of numerous proinflammatory cytokines and has been implicated in the molecular biology of ARDS.131 Although adrenal production of CS is expected to increase in response to the insult that triggered ARDS, hormone levels may be insufficient despite the increase, or their effect may be dampened by various mechanisms of tissue resistance to CS.132 Therefore, supplementation with exogenous CS is a theoretically appealing option for abrogating the inflammatory cascade that drives lung injury in ARDS. Results of initial trials using high-dose therapy administered early in the course of ARDS and for brief periods (eg, methylprednisolone 30 mg/kg every 6 hours for 24 hours) were disappointing.133,134 Subsequent investigations proposed that the inflammation fueling the exudative phase of ARDS can follow 2 distinct trajectories: reduction over the course of the first week with more favorable clinical outcome as opposed to persistence leading to fibroproliferation and eventual fibrosis with inferior outcome.135,136 It was hypothesized that the transition to fibroproliferation and then to fibrosis is the consequence of protracted inflammation and the associated aberrant lung repair—a process that could be interrupted by CS.137-139 This theory led to a reorientation of CS trials toward targeting “unresolving” ARDS with longer courses but lower doses (eg, methylprednisolone 0.5 mg/kg every 6 hours). In a small RCT predating universal LTV ventilation, Meduri et al reported improved short-term survival and oxygenation in the group randomized to a 32-day methylprednisolone taper for unresolving ARDS by day 7 compared to placebo.140 ARDSNet then conducted a larger RCT in the LTV era that enrolled subjects with ARDS persisting 7 days or longer and randomized them to placebo or a 21-day course of methylprednisolone followed by a taper.141 Although secondary short-term endpoints such as ventilator-free and ICU-free days favored the CS arm, 60- and 180-day mortality rates were similar. Importantly, receipt of CS conferred a higher mortality on those who entered the study 14 days or more after the onset of ARDS. The treatment group also suffered more adverse events attributable to neuromuscular dysfunction, which is a known side effect of CS therapy. In fact, this and other potential harms of CS may account for the discrepancy between the short-term benefits demonstrated by these trials and the lack of a survival advantage in the long run. Starting CS beyond 14 days may likewise have accentuated the drawbacks of CS because at that stage suppression of inflammation may no longer be beneficial or possibly may be detrimental. Meduri et al subsequently investigated the role of prolonged low-dose CS therapy (methylprednisolone 1 mg/kg/d) in early (≤ 72 hours) severe ARDS in a placebo-controlled RCT.142 By day 7, there was a significant difference in the degree of lung injury and extubation rate favoring the CS arm. Other short-term outcome measures such as ICU stay and hospital mortality were also reduced. The extreme heterogeneity of the available CS RCTs has made the results of published meta-analyses difficult to interpret. Overall, they seem to parallel the 3 RCTs discussed above in showing improvement in 28-day metrics,141 which then disappears when 60-day mortality is considered.144 The systematic reviews support the notion, however, that CS can be administered to ARDS patients without an increase in adverse events such as new infections or neuromyopathy.145,146 In addition to heterogeneity among studies, as a clinically defined entity, ARDS patients within a given trial invariably encompass a spectrum of pathology that includes not just diffuse alveolar damage but also other substrate that is more (eg, organizing pneumonia) or less (eg, influenza pneumonitis) responsive to CS therapy.147,148 It is not difficult to envision how CS could have a differential effect on individual subjects within such a variable study population. Many questions about the efficacy of CS in ARDS remain unanswered. For example, should mortality be the dominant outcome measure or could protection against lung fibrosis and therefore eventual disability among survivors be viewed as a comparably important endpoint? Despite the uncertainty, CS is currently one of the most commonly used adjunctive therapies in the management of ARDS.149
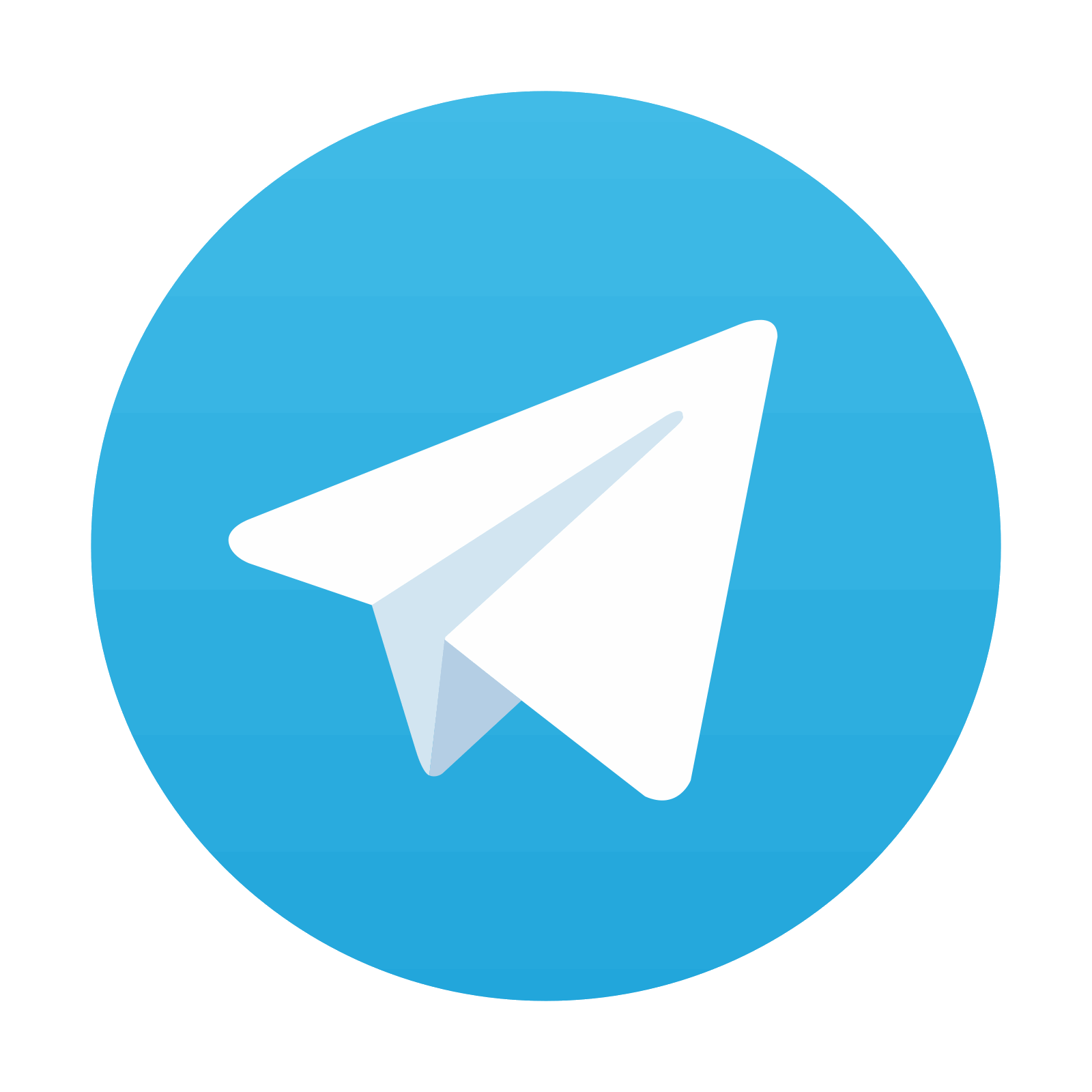
Stay updated, free articles. Join our Telegram channel
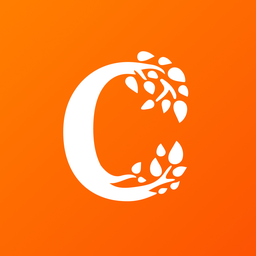
Full access? Get Clinical Tree
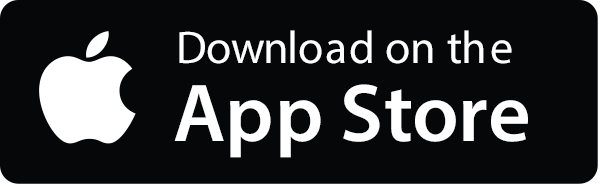
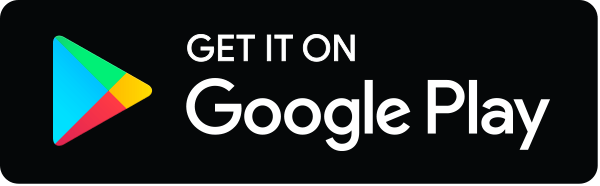