Abstract
In a recent review of the performance of closed-loop systems in anaesthesia, Brogi et al [2] defined a closed-loop system as an automated control system designed to maintain a given variable around a desired set point. The key components of these systems, sensors, controllers and actuators, are outlined in Table 6.1.
In the not too distant future the anaesthesiologist, surgeon and patient may arrive at the hospital in self-driving cars. It is hard to imagine they will be content to interact in the operating room with largely manually operated medical equipment
Introduction
In a recent review of the performance of closed-loop systems in anaesthesia, Brogi et al [2] defined a closed-loop system as an automated control system designed to maintain a given variable around a desired set point. The key components of these systems, sensors, controllers and actuators, are outlined in Table 6.1.
Table 6.1. Components of a closed-loop system (after Brogi et al [2]).
Input | Function | Output | |
---|---|---|---|
Sensor | Appropriate monitors and sensors | Measures the target parameter | Produces a feedback signal |
Controller | Set point Feedback signal | Compares feedback signal and desired set point | Instructions to delivery device |
Actuator | (Digital) signal for controller | Controls physical device (e.g. syringe driver) |
To this list we can add a ‘supervisor’ component. The supervisor (clinician) determines the set point for the various parameters being controlled. These set points include physiological and pharmacological targets. During anaesthesia the ‘inputs’ for the supervisor include the progress of the procedure, the response of the patient to interventions thus far, and a concept of the way in which the patient and the controller/actuator system will respond to a change in set point. The output of the supervisor is updated set points for one or several controllers. This supervision, or integration, is part of the function of the anaesthesia provider.
Brogi et al reviewed the performance of automated control of a single variable [2]. This general classification can be extended to fully automated anaesthesia with an algorithmic supervisor overseeing a wide range of inputs (sensors and monitors) and controlling multiple outputs (drug delivery systems). The anaesthesia provider now moves outside the system to gain a general oversight (supervision) of the proper functioning of the system in a similar manner to the pilot of a modern airliner monitoring the function of the autopilot.
While the schema in Table 6.1 was developed to describe fully automated systems, the same terminology can be used to describe most methods that maintain a particular drug concentration or component of anaesthesia. In many parts of the world, and within the working lifetime of many practising anaesthetists in the most developed economies, the anaesthesia provider, perhaps aided by some basic monitoring, fills all four roles, i.e. acting as sensor, supervisor, controller and actuator. Clinical signs (feeling the pulse, sweating, pupil size, breath sounds etc.), possibly aided by very basic monitors, make up the ‘sensors’. The ‘actuator’ directly injects drugs and adjusts the vaporizer The supervisor and controller functions might appear similar in this model, but it is useful to think of them as separate, with, for instance, the controller focussing on maintaining a given vapour concentration while the supervisor is thinking about the overall progress of the procedure.
As available technology improves, various tasks are handed off from the provider to various devices. Devices such as automated blood pressure measurement and pulse oximetry are generally more reliable because the measurement is not dependent on the user physically taking the measurement. In addition, these devices can be particularly useful at times of high workload. Finally, they can give information that is not otherwise available such as SpO2, respiratory gas composition and the degree of neuromuscular blockade.
Target controlled infusion (TCI) is specifically discussed below. A concept underlying this chapter is that every anaesthetic represents some form of target control with the anaesthesia provider performing a variable number of the roles described above. There is always a desired set point (or target) for each component of the anaesthetic mix. These targets may be clinical end-points, such as keeping the blood pressure within a range, or intermediate targets relating to anaesthesia delivery, such as vapour or O2 partial pressure. Different providers and providers in different settings use a variety of approaches to achieve and maintain similar targets.
The aim of this chapter is to briefly discuss emerging tools available to the clinical anaesthetist and how they can help optimize delivery of anaesthesia to the individual patient using the concept of a closed-loop system as a framework. While recognizing and touching on the importance and increasing role of an increasing number of sensors, the primary focus of this chapter is on tools that aid delivery of drugs to achieve desired set points and on the improved understanding of the concepts underlying these tools. The emphasis is on commercially available devices with occasional reference to research systems and those available to the enthusiast to illustrate various concepts. The examples chosen are representative of the various concepts and technologies rather than representing a comprehensive review.
The following abbreviations will be used: end-expired partial pressure = FA; inspired partial pressure = FI; fresh gas flow = FGF; effect site partial pressure (inhaled agents) or effect site concentration (IV drugs) = Ce. When discussing the kinetics of inhaled anaesthetics, the term ‘partial pressure’ rather than concentration is used because it is the partial pressure gradient that drives transport (concentration = partial pressure × solubility according to Henry’s Law).
The Problem of Achieving a Desired Set Point
Although most anaesthesia providers would think of the set point for these control systems as a given drug concentration in a particular compartment, in fact the objective of the supervisor is a given anaesthetic state. Glass provides a useful definition of the objectives of anaesthesia [3]. In addition to providing optimal operating conditions and minimizing physiological trespass, the anaesthesia ‘state’ is (1) absence of recall and (2) absence of both motor and sympathetic response to noxious stimulus [3]. These effects are primarily mediated by hypnotics in the brain and opioids in the spinal cord, respectively. While volatile anaesthetics are frequently used primarily for their role as hypnotics, they also have direct effects in the spinal cord [4, 5] allowing them to be used to provide single agent anaesthesia. There is considerable synergy between these drug groups on these effects [6, 7] as illustrated in Fig. 6.2 (also see Chapter 3).
Fig. 6.2 A stylized isobologram. The concentration of hypnotic is on the y-axis and the opioid concentration on the x-axis. The solid curve represents the combination at which 50% of a population will (or will not) respond to a standard noxious stimulus. The dashed line is the 90th centile where 90% will not respond (but 10% will). The y-intercept, with no opioid, represents the effect of hypnotic alone and is the MAC fraction for the given effect. The early part of the curve is relatively steep and, in a standard 70 kg person, 100 μg of fentanyl will reduce volatile requirement by 50% for five to ten minutes. Note that the curve does not intercept the x-axis. Early studies used the interaction between single hypnotic opioid pairs. Data on the relative potencies of the volatile agents (MAC equivalents) and synthetic opioids allow different combinations and mixtures to be plotted on the same set of axes.
During surgery the degree of stimulus and hence anaesthetic requirement changes frequently. Once surgery is completed, we want minimal residual effect of our drugs beyond a suitable level of analgesia. This requirement to continually adjust and titrate anaesthesia is made more complex because most drugs used in anaesthesia do not reach equilibrium during the course of a typical procedure, which implies that maintaining a constant plasma (or effect-site) concentration requires a continually decreasing delivery rate (the exponential decreasing transfer component of bolus–elimination–transfer, or BET, scheme) [8]. Increasing the effect of a given drug is relatively straightforward, although as will be discussed below, simply increasing an infusion rate or vaporizer setting does not produce an immediate change in effect.
Anticipating the rate of decrease in drug effect is more complex and in particular has very little to do with conventional pharmacokinetics (PK) based on elimination half-times and equilibrium volumes of distribution. A good illustration of this disconnect is the concept of context sensitive half-time, the time for a drug concentration to decrease by 50%, which is influenced by the duration of infusion (‘context’ refers to the duration of administration) [9, 10].
Many drugs used in anaesthesia that we consider rapidly acting, including volatile anaesthetics [10] and opioids such as fentanyl, alfentanil and sufentanil, continue to redistribute for many hours [11]. Although the rate of elimination of inhaled agents via the lungs can be manipulated, redistribution still plays a role and is the basis for the influence of duration of delivery [10] and differing physico-chemical characteristics of different agents on the rate of elimination [12].
The combination of changing stimulus intensity, hysteresis, context sensitive half-times and drug interactions makes drug delivery in anaesthesia surprisingly complex and makes the conventional approach to drug dosing and delivery which considers just amount and timing of drug delivered to the patient less useful. In anaesthesia we have a target that is changing and are working with drug kinetics that change over time. Measuring or modelling drug levels that are more closely related to drug effect considerably simplifies this control task.
The Volatile Cascade
To illustrate the issues with drug delivery during anaesthesia, consider the ‘cascade’ in volatile anaesthetic partial pressure from the vaporizer of a conventional anaesthetic machine to the effect site. In some ways, this is analogous to the oxygen cascade with a partial pressure gradient from outside the body to the final site of action.
The major steps to consider are: (1) fresh gas mix and FGF; (2) the breathing circuit; (3) the lung (including FRC); (4) arterial blood; (5) brain (or effect site). These are illustrated in Fig. 6.3.
The effective volume of each compartment is determined by the physical volume of the compartment and (for the blood and brain) the solubility of that drug in the compartment.
Although we cannot physically observe the individual steps, each causes an additional delay in reaching a new target (effect site) partial pressure after making a change in the vaporizer setting, with the total delay being a composite of these steps. The anaesthesia provider can influence some of these steps (e.g. by manipulating FGF, ventilation, and theoretically cardiac output), but much of the process is determined by the physical characteristics of the system.
Monitoring FA provides information from part-way along the cascade and approximates the partial pressure in blood [13] but is still several steps removed from the effect site. Even with less soluble agents, there is a delay (hysteresis) at each step, which is discussed in detail in the next section. The half-time for transfer from end-tidal to effect site for the halogenated volatile anaesthetics is two to three minutes [14, 15]. The blood to effect-site transfer half-time for propofol is 1.5–2.5 minutes [16].
To overcome the effect of these compounding delays, we tend to overdose our patients: the initial bolus dose of intravenous hypnotic is usually chosen for speed of onset and duration rather than peak effect. Consequently, the desired change in effect may occur before the maximum Ce change has occurred. Thus we risk overshooting the target. For example, after an induction bolus of propofol, the majority of patients will become unarousable long before the peak drug levels are reached, which may contribute to post-induction hypotension (see Fig. 6.4). Similarly an initial bolus dose of NMB is usually chosen for speed of onset and duration rather than peak effect.
Fig. 6.4 The time course of the effect-site concentration (Ce) of propofol following a 150 mg bolus in a 70 kg 40-year-old male using Schnider’s parameters [17]. For this discussion assume the patient will stay unarousable until they return to the Ce at which they became unconscious. The dashed horizontal line is at a propofol concentration of 3 ng/mL and the dotted line is at 5 ng/mL. If loss of consciousness occurs at 3 ng/mL (dashed horizontal line) this will be about 20 seconds after injection with recovery occurring at 5.5 min. If 5 ng/mL is required for loss of consciousness (dotted horizontal line), this will occur at 35 seconds and last for 3.5 min. Peak hypnotic effect of propofol in this model is 8 ng/mL and occurs at 1.6 min (vertical solid line), well after the time of loss of conscious for the 3 and 5 ng/mL scenarios.
Equilibrium versus Dynamic Changes
With volatile anaesthetic agents, studies of minimum alveolar concentration, or ‘MAC’ studies, give us some idea of the Ce required for a given effect [18, 19, 20]. These studies are performed using step changes of FA, and following each step, FA is kept constant for 10–20 minutes to allow time for equilibrium to occur between compartments. While these studies give us an estimate of partial pressure required to block the response to the given stimulus, use of MAC derivatives is much less useful during periods of change if hysteresis is not taken into account, that is unless Ce is considered. Anaesthesia providers need to recognize that FA and Ce can differ significantly during periods of rapid change [21].This is illustrated in Fig. 6.5. This difference between FA and Ce is particularly relevant at the conclusion of anaesthesia, where 50% of the patients are responsive by the time Ce falls to MAC-awake (around 0.3MAC), but the FA at the same time will be significantly lower and is thus a much less useful guide to expected recovery time than Ce (Fig. 6.6).
Fig. 6.5 A plot of inspired and expired sevoflurane (yellow band) and calculated effect-site (‘brain’, grey line) sevoflurane partial pressures using an equilibrium half-time for the effect site (t1/2keo) of 3.2 min [14]. Cross-hatching of the sevoflurane band signifies inspired < expired, or wash-out. This record covers 160 min with solid blocks on the x-axis occuring every five minutes. The lag, or hysteresis, between FA and Ce is clear in this example.
Fig. 6.6 The decline in FA and Ce sevoflurane after nearly 3 h of anaesthesia. At around 168 min the sevoflurane vaporizer (blue line) is changed from 2.8 to 0. At this time, measured FA and calculated effect-site (Ce) sevoflurane are similar. Once the vaporizer is turned off FA falls rapidly towards 0.5% but the decline in Ce is much slower. In this (clinical) example FA sevoflurane falls below MAC-awake (0.6 vol%) 4 min after the vaporizer is turned off. This delay between FA and Ce is called ‘hysteresis’. Eight minutes later, at the right edge of the graph, Ce is still almost 1%. This gives the impression the MAC-awake concept ‘does not work’ – but it does, provided Ce rather than FA is considered.
Similar concepts apply with IV agents. The classic Roberts 10/8/6 regime for propofol [22] rapidly achieves and maintains a plasma concentration of around 3 ng/mL but does not guide us if the target needs changing. A common clinical approach is to make a step change in the infusion rate, but it can take a surprisingly long time to approach a new equilibrium. This delay in plasma concentrations after a change in agent delivery, with Ce lagging behind even more, is illustrated in Fig. 6.7. The figure also demonstrates how the effect of a step change in the rate of delivery of a drug continues long after the desired change in effect has been achieved. A similar pattern is seen after a step change in vaporizer dial setting, even at moderately high FGFs.
Fig. 6.7 The Roberts regime [22] modelled for a 70 kg 44-year-old 174 cm male using the Schnider model parameters [17]. A 1 mg/kg bolus is followed by an infusion run at 10 mL/kg/h for 10 min, then 8 mL/h for a further 10 min, then 6 mL/h.The upper panel shows calculated plasma (dashed line) and effect-site (solid line) concentrations. The lower panel is the same simulation, but at 40 min from the start of the infusion the rate is increased (blue) or decreased (green) by 20%. After 5 min, Ce has changed by 10% and a further 5% change from baseline has occurred by 20 min. This graph illustrates the way in which the effect of a step change continues for some time. We also see that maintaining a constant infusion (6 mL/kg/h) results in a gradual increase in Ce of about 5%/h.
Is All of Anaesthesia about the Control of Targets?
This chapter has, thus far, developed a number of broad concepts.
Firstly, we can think of all anaesthesia drug delivery in terms of a closed-loop controller, with the anaesthesia provider (or wetware1) performing more or fewer of the functions of the controller, especially in the supervisor/controller/actuator domains.
Secondly, for any drug there are several steps between changing the setting on the delivery device, and a change in effect occurring and reaching equilibrium at the effect site. Any of these steps can be the ‘target’ for the control systems.
Together these concepts suggest that all of anaesthesia is ‘target controlled’: the anaesthesia provider always has some idea of the target they are aiming to achieve. The target could be a specific drug level in a given compartment or a given clinical state (unconsciousness, loss of response to a noxious stimulus, control of a physiological parameter …).
Finally, adjusting drug delivery to match changing patient requirements can be complex, even with drugs considered relatively short acting. Given these issues, we appear to do surprisingly well at matching delivery to need in everyday clinical practice.
There is a place for tools that allow more precise control of the various anaesthetic end-points. In the remainder of this chapter we consider a range of approaches to control ‘anaesthesia’ in the context of these issues.
Controlling Anaesthetic Drug Delivery
Recipes
A typical basic recipe is a mg/kg dosing regime, such as ‘induce with 2 mg/kg of propofol’. A more sophisticated example is the Roberts propofol regime [22] which rapidly achieves and then maintains a predetermined target.
Similar regimes have been described for volatile anaesthetics. In 1972 Cowles et al [23] used computer modelling to develop a series of step changes in dial concentration to achieve and maintain a brain concentration of around 1.3%. For halothane this sequence is 3% for 12 min, 2% for 3 min, 1.5% for 100 min and 1.25% thereafter. Mapleson [24] refined this approach to incorporate changes in flow rates and include all modern halogenated agents. The ultimate in recipe-based low-flow volatile anaesthetic delivery is described in ‘The Qualitative Practice of Anesthesia: Use of Closed Circuit’ by Lowe and Ernst [25]. This describes in detail the injection of fixed amounts of liquid agent into a closed circuit at increasing intervals following a square root of time rule. All of these approaches require considerable diligence and attention to detail while managing other aspects of induction of anaesthesia and the start of surgery.
Da Silva et al [26] analysed the performance of the ‘square root of time model’ and found the rate of uptake declined more slowly than predicted with isoflurane and especially with enflurane. This finding was duplicated by Bangaari et al in 2013 [27]. These groups concluded that this approach was not sufficiently accurate for use when agent monitoring is not available, such as in less developed economies [26, 27].
In addition to the intrinsic inaccuracies, these recipes for volatile anaesthetic delivery share the basic weakness of the Roberts propofol regime: they do not allow for a smooth change in effect either because of inter-individual variability in requirement or with a changing surgical stimulus.
These fixed recipe examples are not strictly closed-loop control because there is no feedback (or modification) of the (predetermined) drug dosing. Conversely, the ‘control system’ is working to control the delivery. These approaches are based on average requirements and do not incorporate any acknowledgement of the considerable inter-patient variability.
More recently Hendrickx et al [28–30] have described simple one or two step sequences to rapidly achieve an approximate end-tidal target and low total FGFs. The vaporizer setting can then be modified based on measured end-tidal concentration and adapted to individual requirements. The important difference between these and earlier regimes is the assumption that the recipe is approximate and will be modified by measurement (or feedback).
Monitoring, Sensors and User Controlled Targets
The preceding paragraph introduces a monitor of drug levels (expired agent partial pressure) as a control variable. Various monitors of the patient state, to be considered in this section, extend this concept.
The simplest form of patient monitoring is observation of vital signs such as palpation of pulses, observation of respiratory rate and pattern, pupillary responses and looking for sweating. These are the basis of the classic signs of anaesthetic depth. Beyond that are simple monitors of pulse, ECG and blood pressure, supplemented by pulse oximetry [31]. Another layer of monitoring is provided by respiratory gas monitoring including O2, and CO2. These parameters are general indicators of the patient’s physiology rather than specifically related to anaesthesia.
Monitoring anaesthetic agent partial pressures (both volatile and N2O) in respiratory gases starts to allow more detailed mapping of drug delivery to patient response since it gives some idea of drug levels beyond the rate of delivery. With this technology, the user can adjust the delivered vapour partial pressure and FGF rates to achieve a given FA and also adjust the target FA to meet individual patient requirements. Anaesthetic gas monitoring also facilitates different approaches to delivery such as low-flow and closed-circuit.
Despite some initial debate about the value and safety of gas monitoring [32], the place of the monitors listed above is well established. Physiological monitors give an indication of the general well-being of the patient. More recently attention has focussed on developing monitors of the specific components of the anaesthetic state.
An increasing number of monitors use an EEG signal to give an indication of the degree of hypnosis. These include bispectral index (BIS), qCON, Spectral Entropy, Narcotrend, Patient State Analyser and many others [33]. BIS is the most widely studied of these, but most studies suggest equivalence between devices. These devices can be used to guide anaesthesia, with a BIS in the range of 40–60 a commonly reported target.
A number of devices attempt to quantify the degree of response to noxious stimulus with varying success. Monitors include SPI (surgical plethysmographic index), CVI (composite variability index, based on the BIS signal), qNOX, the analgesic nociception index (ANI) and pupillometry. This is an evolving area with many of these approaches showing promise [34, 35]. An alternative approach based on drug levels alone also predicts response to noxious stimulus as well as several monitors [36].
Target Controlled Infusion
With a target controlled infusion (TCI) system, the user enters a target drug concentration and the computer system delivers drug at a rate to achieve and maintain the specified target. Infusion rates are based on combined PKPD models which incorporate patient characteristics (‘covariates’) such as gender, age, height and weight (see Chapter 4). Although some systems and models target plasma concentrations, increasingly the target used is the effect site.
The unique advantage of TCI is the ability to change the target concentration, not just the delivery rate. A recipe based approach can achieve and approximately maintain a predetermined concentration for a period of time, but changing to a new target is difficult as discussed above. A TCI system will achieve this change rapidly. For example, to decrease the target, a TCI system will stop the infusion for a period of time and then restart it at an appropriate rate, producing a step change as rapidly as drug kinetics allow. TCI systems will maintain a given level (FA, Cp, or Ce) over a long period of time by gradually decreasing the infusion rate as compartments fill up.
Although several hypnotics and opioids were investigated as prototype TCI systems developed from 1979 onwards [37], the first commercial TCI system, the Diprifusor [38], was introduced in 1996 and specifically designed for propofol. ‘Open TCI’ systems became available from 2002 allowing the use of a range of drugs including generic brands of propofol, remifentanil, alfentanil and sufentanil [39]. Fentanyl and midazolam are also modelled in some devices. The performance of models used in TCI systems is often described using Varvel’s criteria: median performance error (MDPE, a measure of accuracy) or ‘togetherness’, median absolute percentage error (MDAPE, a measure of bias or ‘offset’), divergence (drift over time) and wobble (a measure for stability/oscillations) [40].
The theory, history and development and the contrasting place of TCI systems in the USA and the rest of the world has been reviewed in detail recently [37, 39, 41, 42]. The software for many systems is available for download from the OpenTCI Initiative (www.opentci.org). Several systems, including STANPUMP2 and RUGLOOP11,3 continue to be maintained and used in studies where TCI administration is advantageous.
Automated Vapour Control
As with the development of TCI, the components of the control loop including the technology (microprocessors) and the understanding of the underlying kinetics were developed long before these systems became commercially available. Automated feedback control of anaesthetic vapour was first described by a UK group in 1983 for halothane [43] and isoflurane [44]. The key to this system was the availability of an accurate rapid-response monitor of the agent’s partial pressure. This system used a computer controlled syringe driver to inject liquid agent into a conventional closed-circle system. This group was able to accurately maintain FA and quantify the uptake rate of these agents [43, 44]. They found that although the overall rate of uptake followed an exponential washing pattern, there was poor correlation with various anthropometric measurements and there was considerable inter-patient variability [44]. These results support the need for drug delivery to be based on measurements from the individual rather than a population based recipe.
In 1986 Westenskow et al [45] described a custom made closed-loop system for vapour which maintained the halothane FA and also adjusted O2 delivery to keep the circuit volume constant, thus allowing vapour and oxygen uptake to be measured [46]. This controller incorporated aspects of modelling as well as information from the sensor. This group demonstrated more accurate and stable control of vapour partial pressure with the controller than with manual control.
The first commercial anaesthesia delivery system incorporating target controlled vapour delivery was the Physioflex™ (Dräger) introduced in the late 1990s, and followed by the Zeus™ (Dräger) in 2003. More recently existing anaesthetic delivery systems have been enhanced to include automated vapour control. End-tidal control was added to the GE-Aisys in 2010 and more recently a modified Maquet ICU ventilator, the FLOW-i, was released. These devices all allow the user to directly set a target FA. These machines adjust both the FGF and rate of delivery of vapour to the system to achieve and maintain the desired target. The maintenance, or minimum, FGF can also be set by the user, with the lowest FGF being 0.3 L/min, 0.1 L/min and closed circuit with the Aisys, FLOW-i and Zeus, respectively; all are likely to evolve to (near) closed-circuit conditions in the very near future.
By targeting FA, automated vapour control systems offer several features in addition to the ability to achieve and maintain the target FA. These systems minimize FGF and consequently reduce the amount of volatile agent used [47, 48, 49]. With modern inhalational agents, this leads to considerable cost savings and decreases the potential environmental impact of these agents [50, 51]. The control point is moved further down the ‘volatile cascade’, closer to the end-point of the cascade, the theoretical Ce, which reduces the delay between a change in delivery initiated by the user and a change in effect, improving the ability to match drug delivery and effect.
Unlike systems for IV drugs which rely on models only, end-tidal agent controllers are able to directly measure the end-point being targeted. This allows the system to compensate for physiological changes affecting the uptake and distribution of volatile anaesthetics. For instance, changes in cardiac output (and hence blood flow through the lungs) affect the rate of removal from the gas phase into the blood and thence to the rest of the body. If cardiac output falls, the partial pressure in the lungs rises (and so will that in the blood leaving the lungs). These changes and the adaptation of the controller can be clearly seen and are illustrated in Fig. 6.8 and Fig. 6.9. Zbinden et al [46] were able to demonstrate this interaction using a closed-loop control system in sheep in 1986. Although some argue that true manual control of agent delivery requires a detailed understanding of agent kinetics, we have suggested that observing and understanding how such a controller reacts to these changes can provide much better insights into the underlying process [52].
Fig. 6.8 This figure illustrates the decrease in uptake of sevoflurane over a 40-min period as an example of how automated control of anaesthetic agent can illustrate the underlying kinetics. In this sample, an FA of 2.5% sevoflurane (ET Agent) was set on a GE-Aysis machine. The lower solid line is the measured FA sevoflurane which remains relatively constant. The inspired sevoflurane partial pressure (Fi Agent, with measured = upper solid line, linear best fit = dashed) decreases from around 3.4% to 3.2%, or approximately 3%/h.
Fig. 6.9 A demonstration of how the response of the end-tidal controller to physiological perturbation can in and by itself illustrate the kinetics of inhalation anaesthetics. During off-pump CABG, the end-tidal target was set at 1.8% on a GE-Aisys machine. The top trace is FACO2 (ETCO2 on right Y-axis), middle and lower traces are inspired and expired sevoflurane partial pressures (left Y-axis), respectively. At 61 min, the surgeon manipulates the heart, resulting in a fall in cardiac output and pulmonary blood flow. As a result, at about 61.5 min, FACO2 starts to fall and FAsevo (ETsevo) rises slightly. This is followed by a large fall in FI sevo as the controller works to maintain the target FA sevo. This example again shows the utility of automated control; if FI sevo had not changed, FA sevo would have increased by about 0.25% (equivalent to the fall in FI sevo). Furthermore, the change in FI sevo illustrates the changes in uptake because uptake is proportional to the inspired – expired gradient and FA remains nearly constant.
Most TCI and automated vapour controllers manipulate drug delivery to achieve the new target as quickly as possible within the physical limits of the delivery system and the kinetics of the drug. Although the electronic delivery systems have the potential to achieve any vapour concentration up to saturated vapour pressure, current systems limit the rate of delivery to that achievable with an out of circuit vaporizer with high flows. The Maquet FLOW-i has the ability to set a rate of change that is slower than the maximum, which allows changes in FA to occur gradually [53]. This may be useful for instance in anticipation of the start of surgery or to allow FA to gradually fall towards the end of surgery.
These automated systems also allow the user to target the control O2 partial pressure. For the Zeus and FLOW-i the target is the inspired O2 partial pressure, for Aisys it is the end-expired partial pressure. The FGF rates of O2 and either air or N2O are continually adjusted to ensure delivery of adequate amounts of O2, facilitate changes in vapour partial pressure, and to minimize FGF and thus agent usage, all while ensuring the desired O2 target partial pressure is maintained.
These systems have been used to provide anaesthesia in millions of patients over the past decade [1]. They have been shown to reduce vapour consumption and more accurately achieve and maintain the chosen target when compared to manual control [54]. The number of actions required by the anaesthetist is significantly reduced [49]. This can be especially advantageous at the start of a complex case since the user is freed from the task of controlling drug delivery [55] and the risk of unintended overshoot is reduced. The versatility of these systems is demonstrated by a report of an automated targeted vapour control using an adaptive algorithm based on direct injection of liquid isoflurane into a closed circuit used in horses of up to 630 kg [56].
Guided Targeting
Guided targeting is a step back from automated target control (both for IV drug infusion and vapour delivery). In these systems, a computer assembles information on drug delivery and, using the same models used in automated systems, displays current, past and future drug levels. The anaesthesia provider can use this information and adjust the rate of drug delivery to maintain a constant concentration or to have the concentration change over time or to see the effect of various dose regimes over time. Shafer and Egan describe this as ‘passive TCI’ [41].
Many TCI infusion pumps can be operated in this mode. This approach can be useful during induction with propofol. Stopping or reducing a fixed rate infusion when the patient becomes unarousable minimizes (and optimizes) the dose delivered, gives some idea of the concentration required to reach a certain clinical end-point in the individual patient and potentially reduces side effects. This technique avoids the overshoot commonly seen with bolus induction given manually or after setting a high initial target.
Commercial interaction displays (see below) provide real-time Cp and Ce information for a wide range of drugs, including many hypnotics, synthetic opioids and neuromuscular blocking agents. These systems allow ‘manual targeting’ of many drugs not included in TCI systems.
Manually targeting a drug towards a clear measure of effect, such as neuromuscular blocking drugs, illustrates many of the principles discussed in the opening section of this chapter. Furthermore, combining the time course of Ce of a drug with a measure of effect allows the drug amount to be titrated in such a manner that the effect (and by definition Ce) is kept constant, or that the effect can be adjusted in a predictable way, for instance by giving a small additional dose of an NMB agent a minute or two before the start of closure of an abdomen.
Manual targeting also demonstrates the time course of the effect of a bolus. In the abdominal closure example, it becomes clear that a dose of neuromuscular blocking agents given when the surgeon complains 1/4 of the way along the muscle closure will be having its maximal effect about the time the wound is closed (Fig. 6.10). In the same way, the relationship between propofol Ce and loss of consciousness becomes explicit by having it displayed and gives an indication of the likely duration of effect of the induction propofol bolus as illustrated in Fig. 6.4 above.
Fig. 6.10 Redrawn from a screen shot of the ‘relaxation’ component of the Navigator display. Calculated effect-site rocuronium concentration is plotted over time. After an initial 40 mg bolus of rocuronium at 10:45, five incremental 10 mg doses are given when the response to a train-of-four reaches one or two twitches. In any individual, this return occurs at a relatively consistent rocuronium concentration. Towards the end of surgery one can take proactive (blue line) or reactive (green line) approach. In the proactive scenario, at approximately 12:20, a 5 mg bolus of rocuronium is administered in anticipation of abdominal closure. This allows reasonable surgical conditions but does not delay reversal of residual blockade. In the reactive scenario, an additional 10 mg of rocuronium is administered at about 12:25 in response to surgical request. Although closure will be rapidly completed (say by 12:30) it will be 12:40 before 1–2 twitches of the train-of-four return.
Guided targeting is also possible with inhaled agents. We developed a model-based system which provided the user with forward projections of both end-tidal and effect-site agent partial pressure as shown in Fig. 6.11 (and also Fig. 6.5 above) [57, 58]. A similar display is included in the Dräger Perseus machine.
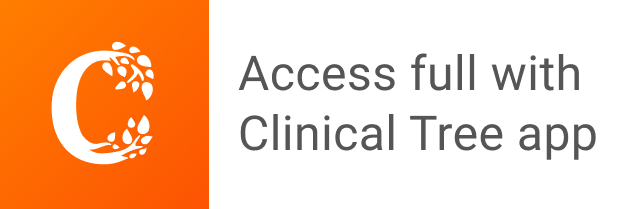