Gary Dhillon1, Elizabeth Herrup2, Paula Holinski3, Peter C. Laussen4, V. Ben Sivarajan5, Stephen J. Roth1, and Justin C. Yeh6 1 Department of Pediatrics (Cardiology), Cardiovascular Intensive Care, Lucile Packard Children’s Hospital Stanford, Stanford University School of Medicine, Palo Alto, CA, USA 2 Pediatric Cardiac Critical Care Medicine, UPMC Children’s Hospital of Pittsburgh, Pittsburgh, PA, USA 3 Department of Pediatric Anesthesia and Pediatric Cardiac Intensive Care, Stollery Children’s Hospital, University of Alberta, Edmonton, Alberta, Canada 4 Harvard Medical School, Boston Children’s Hospital, Boston, MA, USA 5 Pediatric Cardiac Intensive Care Unit, Division of Pediatric Critical Care, Department of Pediatrics, Faculty of Medicine and Dentistry, Stollery Children’s Hospital, University of Alberta, Edmonton, Alberta, Canada 6 Cardiac Intensive Care, Heart Institute UPMC Children’s Hospital of Pittsburgh, Department of Critical Care Medicine, University of Pittsburgh School of Medicine,, Pittsburgh, PA, USA The pediatric cardiac intensive care unit (CICU) serves as the primary destination for critically ill children with congenital and acquired heart disease. It provides an expert model of care by bringing the broad range of specific expertise to the bedside of every CICU patient. Ideally, this allows the cardiac intensivist to engage in collaborative and dynamic decision‐making that involves anesthesiologists, cardiac surgeons, interventionalists, imaging specialists, electrophysiologists, and heart failure/advanced cardiac therapies specialists, thus serving to provide highly effective and efficient care delivery to patients. In contrast to general pediatric intensive care, the majority of CICU admissions are planned. With preoperative and precatheterization review and fetal echocardiography, it is possible and necessary to plan most admissions and their potential need for CICU resources. It is important to recognize that as survival outcomes have improved in children with congenital or acquired heart disease as a result of the current paradigms in management, the proportion of medical cardiac (MC) and unplanned CICU admissions will be expected to increase over time [1, 2]. Collaboration among CICUs and increased emphasis on quality improvement have significantly improved the outcomes of children with critical heart disease in the CICU setting. Efforts from multi‐institutional collaborations (e.g., the Pediatric Cardiac Critical Care Consortium [PC4], which currently has over 65 CICUs submitting data to its registry) has provided a better understanding of case‐mix and outcome variability among CICUs. Figure 36.1 shows multi‐institutional data from PC4 summarizing CICU admissions by age group, illness category (MC, surgical cardiac [SC]), and admission indication from 2018 to 2020. Figure 36.1 Pediatric intensive care unit admission categories from all centers that contribute data to the Pediatric Cardiac Critical care Consortium (PC4), including 66 centers at time of data collection. Categories evaluated: age group, illness category, indication for admission. Timeframe: January 1, 2018 to December 31, 2020. PC4, Pediatric Cardiac Critical care Consortium. (Source: Data obtained from the Pediatric Cardiac Critical care Consortium (PC4).) A thorough understanding of the pathophysiology of congenital cardiac defects is essential when managing these patients in the CICU. This will influence not only the preoperative management strategy for stabilization and/or resuscitation prior to surgery, but also preparations for the impact of preexisting physiologies such as cyanosis, pressure overload, and volume overload on myocardial performance and recovery after surgery. Understanding the changes in physiology achieved intraoperatively in SC patients is equally important for identifying expectations and the anticipated course in the CICU. In the presence of hemodynamically‐significant residual intracardiac lesions after surgery, the accompanying alterations in pulmonary blood flow, systemic perfusion, and ventricular compliance may significantly affect recovery and length of stay (LOS) in the CICU. Confusing terminologies are often used interchangeably to describe common preoperative and postoperative physiologies. It is important for the care provider to understand the essential elements of the circulation in order to react appropriately to perturbations. Patients with D‐transposition of the great arteries (D‐TGA) have parallel circulations: the deoxygenated systemic venous blood returns to the right ventricle (RV) and is ejected through the aorta while oxygenated pulmonary venous blood is returned to the left ventricle (LV) and is ejected into the pulmonary artery (PA) (Figure 36.2). Although the figure depicts simple transposition anatomy, transposition physiology can occur with other anatomic defects such as “Taussig‐Bing” type double‐outlet right ventricle, where a subpulmonary position of the ventricular septal defect (VSD) and malposition of the great arteries create an unfavorable streaming pattern resulting in parallel intracardiac circulation similar to D‐TGA. Transposition physiology is the term used for this parallel circulatory pattern where PA oxygen saturation is greater than the aortic oxygen saturation. Such a circulation is not compatible with survival over time unless there is adequate intercirculatory mixing allowing for the deoxygenated systemic venous blood into the pulmonary circulation (PA), as well as the oxygenated pulmonary venous blood into the systemic circulation (aorta) [3]. Although 25–50% of patients with D‐TGA have a VSD, the presence of a VSD does not guarantee adequate intercirculatory mixing, as mixing occurs most effectively at the atrial level. Newborns with D‐TGA, irrespective of whether they are diagnosed antenatally or postnatally, can present with profound cyanosis in the absence of respiratory distress due to inadequate mixing. Such patients should be transported to the CICU after commencing intravenous (IV) prostaglandin E1 (PGE1) to maintain a patent ductus arteriosus (PDA) as an additional location for mixing. After confirmation of the diagnosis of D‐TGA with a restrictive or intact atrial septum, a balloon atrial septostomy (BAS) can be performed at the bedside or in the cardiac catheterization laboratory to improve intercirculatory mixing and systemic oxygen delivery. If the atrial communication is restrictive, administration of usual therapies to improve pulmonary blood flow (e.g., supplemental oxygen, inhaled nitric oxide) are often detrimental if used as ongoing preoperative management strategies. These therapies can generate left atrial hypertension, leading to increasing pulmonary congestion and worsening pulmonary compliance with further impairment of atrial‐level mixing. Once an adequate atrial septal defect (ASD) is created, systemic oxygen delivery improves as mixing increases from left to right (saturated pulmonary to desaturated systemic) across the ASD. Subsequently, patients can have the PGE1 infusion discontinued and wean and extubate to await an elective repair with the arterial switch operation within the first week of life [4]. Some patients with D‐TGA may remain hypoxemic despite creation of an unrestrictive atrial communication; such patients are often dependent on PGE1 infusion in the preoperative period to maintain systemic saturations >65% [5]. The failure of adequate mixing at the atrial level may reflect not only differences in ventricular function, ventricular filling, and/or ventricular end‐diastolic pressures (ventricular compliance), but may also be secondary to unfavorable streaming effects. There may also be an element of rebound pulmonary hypertension from PGE1 discontinuation that reduces the volume of pulmonary blood flow without affecting the amount of intercirculatory mixing. Some investigators have suggested a protracted weaning protocol for PGE1 to mitigate this effect [6]. See Chapter 29 for further discussion of D‐TGA. Figure 36.2 The anatomy and physiology of D‐TGA. The diagram shows the typical stable circulation in unoperated D‐TGA. The desaturated systemic venous (SV) blood (blue arrows) and fully saturated pulmonary venous (PV) blood (red arrows) require free mixing at the atrial level (often after a BAS) resulting in anatomically equivalent, left‐to‐right and right‐to‐left shunting. As a result, the saturations show the pattern where SVsat < Aosat < PAsat < PVsat. Ao, aorta; BAS, balloon atrial septostomy; D‐TGA, D‐transposition of the great arteries; LA, left atrium; LV, left ventricle; PA, pulmonary artery; RA, right atrium; RV, right ventricle; sat, O2 saturation. Complete mixing lesions represent a variety of anatomic entities that have a common chamber where pulmonary and systemic venous return completely mix before exiting. The conventional terminology for this is single ventricle physiology because it refers to a physiology in which the PA saturation and aortic saturation are equal. These lesions can have either ductal‐dependent pulmonary blood flow (e.g., tricuspid atresia, pulmonary atresia with intact ventricular septum, or pulmonary atresia with VSD) or ductal‐dependent systemic blood flow (e.g., hypoplastic left heart syndrome [HLHS], double‐inlet left ventricle [DILV]). Although the majority of these lesions will require a palliative single ventricle surgical strategy, some patients with single ventricle physiology have two anatomically‐adequate ventricles, allowing for an initial septation procedure (e.g., truncus arteriosus, tetralogy of Fallot [TOF] with pulmonary atresia). This is important when considering the systemic oxygen saturation (SaO2) targets in managing these patients. The traditional targets of SaO2 of 75–85%, partial pressure of O2 (PaO2) of 35–45 mmHg, mixed venous O2 (MvO2) saturation, and/or near‐infrared spectroscopy (NIRS) of 50–60%, along with oxygen extraction fraction of ~25% together with appropriate blood pressure for age, systemic vascular resistance (SVR) state, and adequate markers of end‐organ function (e.g., lactate level, urine output, clinical examination), are important when trying to balance pulmonary blood flow (Qp) and systemic blood flow (Qs) in a preoperative or postoperative patient with limited combined ventricular output. When combined ventricular output is not a limiting factor (e.g., preoperative patients with tricuspid atresia, those with truncus arteriosus), it is important to recognize that strict management of Qp/Qs balance is unnecessary in the absence of respiratory embarrassment or signs of inadequate systemic oxygen delivery (DO2). See Chapter 30 for further discussion of single ventricle lesions. Streaming is the phenomenon where systemic venous blood (or pulmonary venous blood) can pass through to the systemic circulation (or pulmonary venous blood to the pulmonary circulation) due to specific anatomic abnormalities that result in an anatomic chamber that is common to both circulatory systems (e.g., a common atrium, the absence of common arterial septum, truncus arteriosus, double‐outlet right ventricle). This streamed blood may or may not be completely mixed before it reaches the arterial outlet. If it is incompletely mixed, the physiology is likely to be that of transposition physiology (PAsat > Aosat), whereas streaming that is completely mixed will be single ventricle physiology (PAsat = Aosat) [3]. Shunting between the pulmonary and systemic circulations can be intracardiac (across an ASD or a VSD) or extracardiac (across a PDA, aortopulmonary window, decompressing venovenous collaterals, aortopulmonary collateral, or arteriovenous malformation). Depending on the size of the communication and the differential pressures and resistances between the pulmonary and systemic circulations, patients may have decreased or increased pulmonary blood flow and may be either cyanotic or acyanotic, respectively. Shunts that increase pulmonary blood flow may occur either between the atria, ventricles, or the great arteries, and they can be simple or complex. The degree of restriction across these shunts also affects their potential clinical significance. The most common clinical signs seen with increased pulmonary blood flow are tachypnea, respiratory distress, tachycardia, difficulty feeding in neonates/infants, failure to thrive, and diaphoresis. The amount of flow across a “simple” left‐to‐right shunt depends on the size of the defect and the balance between pulmonary vascular resistance (PVR) and SVR. It is important to understand that this is a physiologic term and has no direct relationship to specific diagnoses (Table 36.1). Therefore, patients who have a simple shunt may have: If the simple shunt is “unrestrictive,” the physiologic consequence for all the above mentioned diagnoses will be the same, i.e. excessive pulmonary flow and volume overload to the ventricle receiving the increased pulmonary venous blood flow plus secondary respiratory embarrassment. The degree of shunt will be determined not by the size of the defect, but rather by the relationship between SVR and PVR [7, 8]. The clinical manifestation will also be the same, namely congestive heart failure (CHF) and pulmonary hypertension, although some patients will be cyanotic and others acyanotic, depending on the degree of intracardiac mixing and streaming. On the other hand, for a simple “restrictive” shunt, when the orifice or size of the shunt is small, the size of the communication is the limiting factor determining the increase in pulmonary blow flow; relative SVR and PVR may have little or no impact in this situation. In this circumstance, there is minimal overload to the ventricle receiving pulmonary venous flow, and the pulmonary circulation is protected from excess pressure and flow; as a result, patients may be relatively asymptomatic and present at an older age with nonspecific symptoms such as recurrent respiratory infections or failure to thrive. Table 36.1 Simple shunts: defects or surgical procedures contributing to an increased pulmonary blood flow/systemic blood flow (Qp/Qs) AP, aortopulmonary; ASD, atrial septal defect; BT, Blalock–Taussig; CAVC, complete atrioventricular canal; D‐TGA, dextro‐transposition of the great arteries; DORV, double‐outlet right ventricle; HLHS, hypoplastic left heart syndrome; MA, mitral atresia; MAPCA, major aortopulmonary collateral artery; PA, pulmonary atresia; PDA, patent ductus arteriosus; TA, tricuspid atresia; VSD, ventricular septal defect. In the presence of additional pulmonary or systemic outflow obstruction, the ratio of pulmonary‐to‐systemic blood flow (Qp/Qs) is determined by the size of the orifice and the outflow gradient as well as SVR and PVR. The obstruction may be fixed (valvular aortic or pulmonary stenosis) or dynamic (some forms of TOF, tricuspid atresia with normally related great arteries and a dynamically restrictive muscular VSD). If the increase in pulmonary blood flow and pressure persist over months to years, structural changes occur within the pulmonary vasculature until eventually PVR becomes irreversibly elevated [7–9]. The time course for developing this pathology, termed pulmonary vascular obstructive disease (PVOD), depends in part on the degree of volume overload to the pulmonary circulation, degree of pressure overload to the pulmonary circulation, exposure of the pulmonary vascular bed to cyanosis, and duration of exposure to these factors in the setting of a shunt [10]. Changes may be evident by 4–6 months of age in some lesions (e.g., large VSD or CAVC). The progression can be more rapid when the volume and pressure overload to the pulmonary circulation are increased, such as with truncus arteriosus. When pulmonary blood flow is increased in the absence of pressure overload, such as in simple secundum ASD, PVOD typically develops much more slowly, if at all during childhood. As PVR decreases in the first days to weeks after birth, and the hematocrit falls to its lowest physiologic value, the increased left‐to‐right shunt and anemia‐related high‐output state result in volume loading on the systemic ventricle along with CHF. A typical pressure–volume loop for a volume‐loaded ventricle is shown in Figure 36.3. The end‐diastolic volume is increased and the end‐diastolic pressure–volume line displaced to the right indicating reduced contractility. The time course over which irreversible ventricular dysfunction develops is variable, but if surgical/catheter intervention to correct the volume overload is undertaken within the first 2 years of life, residual dysfunction is uncommon [11]. The volume load on the systemic ventricle and increased end‐diastolic pressure contribute to increased pulmonary edema by increasing pulmonary venous and lymphatic pressures. Decreased lung compliance and increased airway resistance occur secondary to small airways compression by distended vessels [12, 13]. This manifests clinically in infants with both tachypnea and increased work of breathing. If tracheally intubated for mechanical ventilation, the lungs may feel stiff on hand ventilation, deflate slowly, and exhibit an obstructive pattern; poor compliance may be apparent during mechanical breaths. Besides cardiomegaly on chest radiograph, the lung fields are usually congested and hyperinflated. Ventilation/perfusion mismatch contributes to an increased alveolar‐to‐systemic arterial (A‐aO2) gradient and dead space ventilation [14]. Minute ventilation is, therefore, increased, primarily by an increase in respiratory rate. PA and left atrial enlargement may compress mainstem bronchi causing lobar collapse. PA and left atrial dilation may be seen on chest radiograph in the form of widening at the hilum from PA contour enlargement and upward splaying of the left mainstem bronchus from distortion due to left atrial dilation. Furthermore, tachycardia, increased fussiness/agitation, and poor weight gain may be seen secondary to increased myocardial oxygen demand in response to pulmonary overcirculation and ventricular volume loading. It is important to appreciate that such clinical scenarios can be present even after surgery in patients who have significant residual intracardiac shunts that cause an increase in Qp/Qs. This may manifest during the early postoperative course as low cardiac output syndrome (LCOS) (see the “Myocardial Dysfunction and Hemodynamic Monitoring” section) or become apparent some days after surgery with an inability to wean from mechanical ventilation or a persistent requirement for vasoactive support. Reductions to pulmonary blood flow from structural heart disease are ultimately related to intracardiac right‐to‐left shunting. The basis for this may be pulmonary outflow obstruction (supravalvar, valvar, subvalvar, or a combination of these), subpulmonary ventricle inflow obstruction (tricuspid stenosis or atresia), or the inability to generate adequate antegrade pulmonary blood flow (Ebstein anomaly, tricuspid valve dysplasia). In patients with noncardiac diseases, such as persistent pulmonary hypertension of the newborn (PPHN), sustained elevations in PVR result in intracardiac (or ductal) right‐to‐left shunting. Pulmonary mechanics and lung volumes are generally normal in patients with reduced pulmonary blood flow. Dead space ventilation is increased, although minute ventilation is only slightly increased to maintain normocapnia, and the A‐aO2 gradient may be increased as well. The lung fields appear oligemic on chest radiograph. Some patients may have impairments in pulmonary mechanics due to anatomic interactions between cardiovascular structures and the tracheobronchial tree. One such group are patients with TOF and absent pulmonary valve (APV) syndrome who can have massively dilated branch PAs; in the setting of a freely regurgitant pulmonary valve orifice, the RV and branch PAs become volume loaded and dilate, leading to compression of the large and small airways. These patients have significant gas‐trapping and expiratory flow limitations, which is a frequent cause of their respiratory failure. Surgical repair during the newborn period is often required to treat respiratory failure in this scenario. Often in neonates with TOF with APV, early prone positioning with positive pressure ventilation (PPV) is required to alleviate bronchial compression and achieve adequate gas exchange; however, emergent sternotomy in unrepaired patients may still be required in some cases to alleviate airway compression despite mechanical ventilation and proning [15, 16]. Figure 36.3 Pressure–volume relationships. In a gradual afterload increase on a ventricle that can compensate (orange line moving from 1 to 3), the stroke volume is maintained at the cost of higher intracavitary pressure (wall stress) and lower ejection fraction. Generally, this does not require a higher EDP (dotted green line). In the failing myocardium (blue line), stroke volume can only be maintained (initially) at a higher EDP (dotted red line); ultimately stroke volume will be compromised (moving from 1v to 3v). An acute afterload increase on a ventricle (pink line) results in a lower stroke volume (moving from 1 to 1P to 2P), despite compensatory mechanisms to augment stroke volume with a higher EDP (dotted purple line). EDP, end‐diastolic pressure; EDV, end‐diastolic volume. The postoperative target SaO2 level is important to establish, as it will guide assessment and therapeutic intervention (Table 36.2). Potential causes of abnormally low SaO2 include: Severe LV or RV outflow obstruction in the newborn may be associated with ventricular hypertrophy and vessel hypoplasia distal to the level of obstruction. If there is complete or near‐complete outflow obstruction, mixing or shunting at the ASD and/or VSD level is required to maintain systemic cardiac output (CO) with adequate arterial oxygen content. A typical pressure–volume loop from a chronic pressure load on the ventricle is shown in Figure 36.3. The end‐diastolic pressure is elevated and the end‐systolic pressure volume line is shifted to the left, reflecting increased contractility in the compensated state (purple line), with an upwards shift along the new slope reflecting a higher afterload state (from 1P to 2P). Maintenance of preload, stability in afterload, adequate contractility, and normal sinus rhythm are important to prevent a fall in CO or coronary hypoperfusion in states of increased ventricular afterload. Since the time course to develop significant ventricular dysfunction is longer in patients with chronic pressure overload compared to chronic volume overload, symptoms of CHF are uncommon, unless obstruction is severe or prolonged. Table 36.2 Factors to consider in a postoperative cardiac surgery patient with an arterial oxygen saturation lower than the anticipated range AVM, arteriovenous malformation; FiO2, fractional inspired concentration of oxygen; O2, oxygen; PA, pulmonary artery; PV, pulmonary vein; PVR, pulmonary vascular resistance; RDS, respiratory distress syndrome; RV, right ventricle; SV, single ventricle; TGA, transposition of the great arteries; VSD, ventricular septal defect. In the immediate postoperative period, it is important to evaluate both systolic and diastolic ventricular function in a previously obstructed but still hypertrophied ventricle. In all of the examples of mixing, shunting, and outflow obstruction described earlier, the mode and method of mechanical ventilation may have a substantial impact on hemodynamics and systemic perfusion. Particularly for neonates and infants, cardiorespiratory interactions are essential to recognize during postoperative management. While changes in the PaO2, partial pressure of carbon dioxide (PaCO2), and pH are well recognized to affect PVR, the mean airway pressure and changes in lung volume during PPV will also affect PVR, preload, and ventricular afterload. In addition to evaluating the adequacy of mechanical ventilation settings by arterial blood gas and chest radiography, it is important that ventilator settings be continually evaluated and adjusted according to hemodynamic response. Intubation of the trachea in a neonate or young infant with CHD may elicit undesirable hemodynamic and metabolic responses. Appropriate anesthetic techniques are thus desirable to secure the airway. Traditionally, uncuffed endotracheal tubes (ETTs) were recommended for children younger than 8 years given the narrowest part of the airway is at the level of the cricoid cartilage. However, more recent studies suggest there is no difference between airway complications with cuffed and uncuffed tubes, particularly in the era of microcuff tubes (high‐volume, low‐pressure cuff) [17, 18]. If a significant air leak exists around the ETT, lung volume, and in particular functional residual capacity (FRC), will not be maintained and fluctuations in gas exchange can occur. During the ventilator weaning process, a significant leak will also increase the work of breathing for some neonates and infants. In these situations, it is, therefore, preferable to exchange the ETT for a larger‐sized tube or to change to a cuffed tube. In contrast, an ETT that is smaller than usual may be necessary in certain situations. This is particularly the case in patients with other congenital defects such as Down syndrome (trisomy 21). Tracheal stenosis may also occur in association with some congenital cardiac defects such as a PA sling. Extrinsic compression of the bronchi may occur by the PA or a dilated left atrium. This may be suspected by persistent hyperinflation or lobar atelectasis on a chest radiograph. Altered lung mechanics and ventilation/perfusion abnormalities are common problems in the immediate postoperative period [14]. Patients who have Qp/Qs > 2 : 1 may have cardiomegaly and congested lung fields on chest radiograph. Patients who have an elevated left atrial pressure may demonstrate signs of pulmonary venous hypertension and pulmonary edema. Additional considerations include the surgical incision and lung retraction, increased lung water following cardiopulmonary bypass (CPB), possible pulmonary reperfusion injury, CPB‐induced surfactant depletion in neonates, and restrictive defects from atelectasis and pleural effusions. In general, patients with limited physiologic reserve should not be weaned from mechanical ventilation until hemodynamically stable and any problems contributing to an increase in intrapulmonary shunt and altered respiratory mechanics have improved. Cardiorespiratory interactions vary significantly between patients and throughout the postoperative course. Consequently, the mode of ventilation must be matched to the hemodynamic status of each patient to achieve an adequate CO and gas exchange. The influence of PPV on preload and afterload is shown in Table 36.3. Frequent modifications to the mode and pattern of ventilation may be necessary during recovery after surgery, with attention to changes in lung volume, compliance, and airway pressure. Changes in lung volume have a major effect on PVR, which is lowest at FRC. Both atelectasis and hyperinflation may result in a significant increase in PVR, as shown in Figure 36.4 [19–21]. At low‐tidal volumes, alveolar collapse occurs because of reduced interstitial traction on alveolar septae. In addition, radial traction on extra‐alveolar vessels such as the branch PAs is reduced, therefore reducing the cross‐sectional diameter. Conversely, hyperinflation of the lung may cause stretching of the alveolar septae and compression of alveolar vessels. An increase in PVR increases the afterload or wall stress on the RV, compromising RV function and contributing to decreased LV compliance secondary to interventricular septal shift (from right to left). In addition to low CO, signs of RV dysfunction, including tachycardia, tricuspid regurgitation, hepatomegaly, ascites, and pleural effusions, may be observed. Table 36.3 Effect of a positive pressure mechanical breath on afterload and preload to the pulmonary and systemic ventricles LAp, left atrial pressure; LVEDp, left ventricle end‐diastolic pressure; LVEDV, left ventricle end‐diastolic volume; PBF, pulmonary blood flow; PR, pulmonary regurgitation; RAp, right atrial pressure; RVEDp, right ventricle end‐diastolic pressure; RVEDV, right ventricle end‐diastolic volume; RVp, right ventricle pressure; TR, tricuspid regurgitation. Figure 36.4 Lung volume impacts pulmonary vascular resistance. In the diagram above, there is a marked decrease in pulmonary vascular resistance when lung volume increases from RV (residual volume) to FRC (functional residual capacity). This is primarily a function of alveoli strutting open extra‐alveolar vessels as they are expanded (drop in extra‐alveolar resistance; dotted line). Further increases in lung volume from FRC to TLC (total lung capacity) cause increases in pulmonary vascular resistance. This is mainly due to compression of intra‐alveolar vessels by air‐trapping (alveolar component; dashed line). Total pulmonary vascular resistance is minimized at FRC (solid line). An increase in mean intrathoracic pressure during PPV decreases preload to both pulmonary and systemic ventricles, but has opposite effects on afterload of each ventricle [22–24]. The increase in pressure in the right atrium and reduction in RV preload that occurs with PPV may reduce CO. Normally, RV diastolic compliance is high and the pulmonary circulation is able to accommodate changes in flow without a large change in pressure. An increase in mean intrathoracic pressure increases the afterload on the RV from direct compression of extra‐alveolar and alveolar pulmonary vessels. This has a number of clinical consequences (Table 36.3). An increase in afterload causes an increase in RV end‐diastolic pressure and myocardial work, which may lead to myocardial ischemia in a patient with limited coronary perfusion. An example of the increase in RV pressure during a positive pressure breath is demonstrated in Figure 36.5. Patients with normal RV compliance and no residual volume or pressure load on the ventricle following surgery usually show little change in RV function from the alteration in preload and afterload that occurs with PPV. However, these effects can be magnified in patients with RV hypertrophy and those with restrictive RV physiology. At particular risk are neonates requiring a right ventriculotomy for repair of TOF with pulmonary atresia or truncus arteriosus; concentric RV hypertrophy poses additional risk. While systolic RV function may be preserved in these situations, the ventricle often demonstrates diastolic dysfunction with increased RV end‐diastolic pressure and impaired RV filling. Figure 36.5 Positive pressure ventilation effects on the right ventricle. Simultaneous tracings of aortic (red) and right ventricle (blue) pressure waveforms during positive pressure ventilation (PPV) in a child with pulmonary artery stenosis. Note the increase in right ventricular pressure to approximately systemic (aortic) level during mechanical inspiration when the afterload on the right ventricle is increased. It is important to emphasize the potentially deleterious effects of mechanical ventilation on RV function. The aims should be to ventilate with a mode that enables the lowest possible mean airway pressure while maintaining adequate lung volume and limiting potential secondary injury to the lung. In patients with lung disease, such as pneumonia, adult respiratory distress syndrome, or lung disease of prematurity, a low tidal volume strategy (4–6 mL/kg) using a low peak inspiratory pressure, short inspiratory time, increased intermittent mandatory ventilation (IMV) rate, and higher levels of positive end‐expiratory pressure (PEEP) is recommended. However, this strategy is also associated with a higher mean intrathoracic pressure and afterload on the RV, which reduces antegrade pulmonary blood flow. In contrast, a strategy utilizing larger tidal volumes (10–12 mL/kg), lower IMV rates (15–20 breaths/min), and larger peak inspiratory pressure–PEEP difference with a shorter inspiratory time (0.4–0.5 seconds) may be preferential in patients with restrictive RV. LV preload is also affected by lung volume. Pulmonary blood flow and, therefore, preload to the systemic ventricle may be reduced by an increase or decrease in lung volume secondary to alteration in radial traction on alveolar and extra‐alveolar vessels. The systemic arteries are under higher pressure and not exposed to radial traction effects during inflation or deflation of the lungs. Therefore, changes in lung volume will affect LV preload, but the effect on afterload is dependent on changes in intrathoracic pressure alone. In contrast to the RV, a major effect of PPV on the LV is a reduction in afterload. Using the law of Laplace, wall stress is directly proportional to the transmural LV pressure and the radius of curvature of the LV and inversely proportional to the thickness of the ventricle. The transmural pressure across the LV is the difference between the intracavity LV pressure and the surrounding intrathoracic pressure. Assuming a constant arterial pressure and ventricular dimension, an increase in intrathoracic pressure, as occurs during PPV, will reduce the transmural gradient and, therefore, wall stress on the LV (Figure 36.6). Therefore, PPV and PEEP can have significant beneficial effects in patients with LV systolic dysfunction or failure (Table 36.3). Patients with LV dysfunction with an increased end‐diastolic volume and pressure can have impaired pulmonary mechanics secondary to increased lung water, decreased lung compliance, and increased airway resistance. The work of breathing is increased, and neonates can fatigue early because of their limited respiratory reserve. A significant proportion of total body oxygen consumption is directed at the increased work of breathing (especially the diaphragm) in neonates and infants with LV dysfunction, contributing to poor feeding and failure to thrive. Therefore, PPV has an additional benefit in patients with significant volume overload and systemic ventricular dysfunction by reducing the work of breathing and oxygen demand. The use of PEEP in patients with CHD has two important effects: recruiting FRC and O2 reserve capacity and redistributing lung water from alveolar septal regions to the more compliant perihilar regions. Gas exchange is improved, and the work of breathing reduced. This is particularly important in patients with a volume load, ventricular dysfunction, and elevated end‐diastolic volume. PEEP should, therefore, be used in all mechanically ventilated patients following congenital heart surgery. However, excessive levels of PEEP can be detrimental by increasing afterload on the RV. Usually 3–5 cmH2O of PEEP will help maintain FRC and redistribute lung water without causing hemodynamic compromise. The use of PPV and PEEP in patients who have undergone either a Glenn or Fontan procedure (cavopulmonary anastomosis) has also been debated. In this group of patients, pulmonary blood flow is nonpulsatile and depends on the pressure gradient between the superior vena cava and the pulmonary venous atrium. During PPV, pulmonary blood flow can be diminished, and during a Valsalva maneuver and at high levels of PEEP, retrograde pulmonary blood flow may be demonstrated by Doppler echocardiography. Nevertheless, the beneficial effects of using low‐pressure PPV and PEEP to achieve desired lung volume, minute ventilation and gas exchange following the Fontan procedure are apparent at the bedside. This strategy rarely contributes to a clinically significant decrease in effective pulmonary blood flow and, in fact, may be more than offset when compared to the elevations in PVR related to a strategy of low‐volume spontaneous ventilation [25, 26]. The use of high‐frequency oscillatory ventilation (HFOV) has been beneficial in the management of hypoxemic and hypercapneic respiratory failure where the goal is to optimize systemic oxygen delivery while minimizing lung injury. The mean airway pressure may be higher than when using conventional ventilation, but can be well tolerated hemodynamically, provided preload is maintained. There is no defined and agreed‐upon indication for HFOV in the literature, but it should be considered in the setting of ventilatory failure with a rising PaCO2 and mean airway pressures >14 cmH2O in neonates and >16 cmH2O in older children using conventional ventilator modes. Recent data using propensity matching suggest that HFOV might reduce the length of ventilation after CHD surgery in neonates and infants [27]. Figure 36.6 Transmural aortic pressure affects left ventricular afterload. By the law of Laplace, the transmural aortic pressure (PTM) is affected by intrapleural pressure. During normal breathing (A), there is very little effect of the intrapleural pressure on PTM and thus left ventricular afterload. Generation of pathologic degrees of negative intrapleural pressure (as with severe airway obstruction or decompensated heart failure) significantly increases left ventricular afterload (B). Provision of positive pressure ventilation (PPV) (C) or pharmacologic afterload reduction (D) can both independently reduce left ventricular afterload. ITP, intrathoracic pressure. Noninvasive ventilation (NIV) using either mask CPAP, bilevel positive airway pressure (PAP), or high‐flow nasal cannula (HFNC) can be a helpful adjunct in patients who are tachypneic with an increased work of breathing and those requiring improved oxygenation through maintenance of FRC. In addition, it can potentially improve CO and ventricular function by reducing wall stress and afterload. NIV also avoids the attendant risk of endotracheal intubation, including escalating sedation requirements and ventilator‐associated pneumonia and tracheitis [28]. HFNC with humidified oxygen at flow rates of between 3 and 10 L/min can be used to help minimize work of breathing and caloric expenditure in patients recently weaned from mechanical ventilation and/or those awaiting surgery. Although the PEEP delivered through this mode is variable, this mode is a helpful adjunct, especially in small patients with resting lung volumes approaching closing capacity [29]. Early extubation, generally defined as extubation within 6 hours of surgery, has been described for various cardiac lesions. Possible advantages of this strategy include fewer pulmonary complications, reduced sedative and analgesic use, shorter ICU and hospital LOS, and reduced resource utilization. Early extubation can be especially beneficial in patients with restrictive RV physiology or absent subpulmonary ventricle (cavopulmonary connection with the Fontan procedure). Some centers have employed collaborative learning models to develop clinical guidelines for early extubation practices. Although this improved rates of early extubation, ICU LOS did not change [30]. One notable exception is in the Fontan patient; not only will spontaneous ventilation improve pulmonary blood flow and CO, fast‐track extubation in the operating room (OR) is an important predictor of postoperative LOS [31, 32]. Furthermore, rates of successful early extubation vary across institutions, but increased specialized nursing in the CICU may improve outcomes [33]. NIV, including HFNC or PAP, has also been utilized, particularly in high‐risk, postcardiac surgical patients, to mitigate the risk of extubation failure. However, there is limited evidence regarding NIV utilization in pediatric CICUs to inform best practice [34]. Weaning from mechanical ventilation is a dynamic process that requires continued reevaluation. While most patients following uncomplicated congenital cardiac surgery will wean without difficulty, some patients with borderline cardiac function and/or residual defects may require prolonged mechanical ventilation and a slow weaning process. As spontaneous ventilation increases during the weaning process, swings in mean intrathoracic pressure may substantially alter afterload on the systemic ventricle. The transition to spontaneous ventilation may cause a sudden increase in wall stress, which then contributes to an increase in end‐diastolic pressure and volume, leading to pulmonary edema and low CO. Inotropic agents, vasodilators, and diuretics should be continued throughout the ventilator weaning process and early after extubation to maintain stable ventricular function as mechanical respiratory support is reduced. The method of weaning is variable. Most patients can be weaned using either a volume‐limited or pressure‐limited mode by simply decreasing the IMV rate. Guided by physical examination, hemodynamic criteria, respiratory pattern, and arterial blood gas measurements, the mechanical ventilator rate is gradually reduced. Patients with limited hemodynamic and respiratory reserve may demonstrate tachypnea, diaphoresis, and reduced tidal volumes as they struggle to breathe spontaneously against the resistance of the ETT. The addition of pressure‐ or flow‐triggered pressure or volume support breaths above PEEP at a level related to the size of the ETT decreases this resistance and is often beneficial in reducing the work of breathing. A flow‐triggered mode of pressure or volume support, with a backup ventilator rate if the patient becomes apneic (assist‐control mode), is particularly useful for neonates and infants who received prolonged ventilation following surgery or have a residual volume or pressure load compromising ventricular function. Patients are often more comfortable weaning in this mode and have reduced work of breathing; the level of pressure support is adjusted according to their gas exchange, respiratory rate, and tidal volume. Numerous factors can contribute to the inability to wean from mechanical ventilation following congenital heart surgery (Table 36.4). As a general rule, however, suspected residual defects causing either a volume or pressure load must be excluded first by echocardiography or cardiac catheterization. Pulmonary edema, pleural effusions, and persistent atelectasis may delay weaning from mechanical ventilation. Residual chest and abdominal wall edema, ascites, and hepatomegaly can each limit chest wall compliance and diaphragmatic excursion. Chest tubes and peritoneal catheters may be necessary to drain pleural effusions and ascites, respectively. Table 36.4 Factors contributing to the inability to wean from mechanical ventilation after congenital heart surgery If atelectasis persists, bronchoscopy is often useful to remove obstructive secretions and to diagnose any extrinsic compression from enlarged PAs, a dilated left atrium, or conduits. Patients rarely fail from mild mechanical or dynamic airway compression alone and often have contributory residual lesions [35]. Phrenic nerve injury can occur during cardiac surgery secondary to traction, thymic resection, thermal injury from electrocautery, or direct transection as a complication of extensive aortic arch and pulmonary hilar dissection. Diaphragmatic paresis (no motion) or paralysis (paradoxical motion) should be investigated in any patient who fails to wean and extubate successfully [36]. Increased work of breathing, abnormal respiratory mechanics, increased PaCO2, and/or an elevated hemidiaphragm on chest radiograph following extubation (i.e., off positive pressure support) are all consistent with diaphragmatic dysfunction. Ultrasonography or fluoroscopy is used to confirm abnormal diaphragmatic movement. For an accurate diagnosis, these imaging investigations must be performed off positive pressure support. Additional discussion of airway management, ventilation, and extubation strategies is presented in Chapters 23 and 24. Fluid restriction and aggressive diuretic therapy are commonly used early after cardiac surgery with CPB and can result in metabolic disturbances and insufficient nutritional intake. A hypochloremic, hypokalemic metabolic alkalosis with secondary respiratory acidosis is a common complication from high‐dose loop diuretic use and can delay the ventilator weaning process. The need for and dosing of diuretic agents should be continually reevaluated. Furthermore, many patients will have limited reserve secondary to preoperative failure to thrive; maintaining adequate nutrition/caloric intake is challenging, given the catabolic state of patients early after surgery with CPB. Adequate analgesia and sedation are necessary to optimize synchronization with the ventilator and maintain hemodynamic stability as well as provide comfort. Depending on the age of the patient, the magnitude of the operation, any residual defects, and the preoperative condition, there is potential for both escalation and prolongation of these requirements. Appropriate analgesia can often be provided with a low‐dose opioid infusion. Although escalation may seem necessary based on clinical concerns, in particular hypertension or tachycardia, these are often secondary to inadequate sedation rather than from pain. A benzodiazepine or nonopioid drug such as dexmedetomidine is useful in these circumstances. The appropriate drug must take into account the anticipated duration of mechanical ventilation and the need to titrate rapidly to drug effect. When early extubation is planned, short‐acting adjuncts such as dexmedetomidine, ketorolac, and acetaminophen are useful [37]. A propofol infusion can be a helpful strategy in selected patient groups for whom agitation during rapid weaning is a sign of intolerance to airway stimulation as opposed to failure to wean from other causes. However, a propofol infusion must be used with caution because of potential hemodynamic complications and the risk of propofol infusion syndrome [38]. Oversedation can impair the weaning process because of somnolence, leading to hypoventilation, failure to trigger the ventilator, and inability to protect the airway. For patients who have required mechanical ventilation for several days to weeks, and, therefore, received higher cumulative doses and a range of analgesic and sedative medicines, rapid weans from analgesics and sedatives can result in withdrawal symptoms. These symptoms can often be confused with pulmonary or cardiac reasons for failure to wean, as respiratory and hemodynamic symptoms may predominate. It is important to have a systematic analgesia/sedation weaning protocol that incorporates the timing of weaning with objective withdrawal assessment and knowledge of pharmacokinetics [39]. Sepsis is a particular risk following cardiac surgery for a number of reasons, including the immunomodulating effects of CPB, risk factors such as an open sternum, the use of vascular catheters for invasive monitoring and IV medications, and liberal use of broad‐spectrum antibiotics. Sepsis can be a cause of failure to wean from mechanical ventilation because of its systemic effects, the associated increased work of breathing, and the imbalance between oxygen demand and delivery. Bronchospasm can complicate mechanical ventilation and the weaning process. While this may reflect intrinsic airway disease, bronchospasm can also result from increased airway secretions, airway infection, and extrinsic airway compression. Treatment with inhaled or systemic bronchodilators may be beneficial, although they should be used with caution because of their chronotropic and tachyarrhythmic potential. The sudden onset of bronchospasm with increased peak inspiratory pressure and difficulty with hand ventilation should raise the immediate concern for acute ETT obstruction or tension pneumothorax. Bronchospasm in patients with labile PVR may reflect acute pulmonary hypertension, and treatment is directed at maneuvers to lower PVR and improve systemic CO. Postextubation stridor may be due to mucosal swelling of the large airway, and treatment with dexamethasone before extubation can be beneficial to reduce edema in patients who have required prolonged use of an ETT. Stridor following extubation can initially be treated with nebulized epinephrine, which promotes vasoconstriction and decreases airway hyperemia and edema. If reintubation is necessary, a smaller ETT should be used. Vocal cord paresis or paralysis can result from surgical trauma to the recurrent laryngeal nerve and can be a cause of upper airway obstruction. Surgery on or near the aortic arch (e.g., repair of coarctation or interrupted aortic arch, and Norwood stage I palliation) is a risk factor for this injury, with symptoms ranging from weak or hoarse cry to significant upper airway obstruction requiring tracheal intubation. Diagnosis is typically made with either awake flexible nasolaryngoscopy or laryngeal ultrasound of the neck; both imaging procedures can be performed at the bedside [40]. The ability to clear secretions and the potential for nosocomial infection are additional concerns in patients who have been ventilated for an extended period of time. Inability to clear secretions because of sedation, bulbar and vocal cord dysfunction, ineffective cough following prolonged intubation, and poor nutritional state with muscle fatigue will result in atelectasis and respiratory failure. Frequent chest physiotherapy and nasopharyngeal suction are beneficial, provided that patients are hemodynamically stable with adequate gas exchange. Furthermore, the use of nasopharyngeal CPAP and/or HFNC can be beneficial by reducing the work of breathing; however, these patients have limited reserve, and frequent reassessment is essential. The accurate assessment of the postoperative patient’s CO should be a focus of management in the CICU. Recognition and effective management of LCOS is critical, as this clinical entity is associated with increased morbidity, including longer duration of mechanical ventilatory support and CICU/hospital LOS, and mortality [41, 42]. Data from physical examination, routine laboratory testing, bedside hemodynamic and electrophysiologic monitoring, echocardiography, and occasionally bedside CO determination are typically sufficient to manage patients optimally. If patients are not progressing as expected and low CO persists, a systematic evaluation for residual lesions should occur. The imaging assessment for residual lesions typically occurs noninvasively first by echocardiography, with the addition of computed tomographic (CT) angiography, if needed. When a more complete assessment with direct hemodynamic measurements is required, then cardiac catheterization should be undertaken. The systemic CO is defined as the product of ventricular stroke volume (in L/beat) multiplied by heart rate (in beats/min). The ventricular stroke volume is determined chiefly by three factors: afterload (the resistance to ventricular emptying), preload, and myocardial contractility. CO is usually indexed to body surface area (BSA, in m2) because it is a function of body mass. Thus, CO/BSA is designated as cardiac index (CI, in L/min/m2). The CI varies inversely with age: normal values in children at rest are 4.0–5.0 L/min/m2, whereas the normal resting CI at age 70 years is 2.5 L/min/m2 [43, 44]. Postoperative patients with low CO can present with a variety of abnormalities on physical examination, bedside monitoring, and laboratory assessment. These manifestations of low CO are listed in Table 36.5. Clinical signs on examination include cool extremities and diminished peripheral perfusion, tachycardia, hypotension, oliguria, and hepatomegaly. An increase in the arterial to mixed venous oxygen saturation (A‐VO2) difference of >30% and a lactic acidosis provide biochemical evidence for low CO. The atrial pressure is a useful measure to follow, and either an increase or decrease could be observed in LCOS. Factors that should be considered when evaluating the atrial pressure following surgery are shown in Table 36.6. The mechanism(s) underlying low CO in a specific patient can be related to either one or a combination of factors following surgery. Strategies for treating the patient with a low CO should focus on optimizing the balance between oxygen consumption (VO2) and delivery (DO2). In LCOS, oxygen and metabolic demand should be minimized by maintaining an adequate depth of analgesia and sedation, including chemical paralysis, in order to avoid movement, reduce muscle tone, and limit oxygen consumption. Strict avoidance of hyperthermia from any cause is essential, and in some circumstances, mild hypothermia may be preferable, although the effect of peripheral vasoconstriction and increase in SVR from hypothermia could have an adverse impact on myocardial wall stress and oxygen requirements. Table 36.5 Manifestations of low cardiac output A‐V, arteriovenous; BUN, blood urea nitrogen; Cr, creatinine; SvO2, systemic venous oxygen saturation. A thorough understanding of the underlying cardiac anatomy, surgical findings, and surgical procedures is essential because this will direct the initial postoperative evaluation and examination. Residual lesions may be evident by auscultation, intracardiac pressures and waveforms, and oxygen saturation data. For example, a large V‐wave on the left atrial waveform may indicate significant residual mitral valve regurgitation. An increase (step‐up) of the right atrial‐to‐PA oxygen saturation of more than 10% may indicate a significant intracardiac shunt across a residual VSD [45]. Table 36.6 Factors that should be considered when the measured atrial pressure is outside of the anticipated range However, if there are significant concerns for important residual lesions that are compromising CO and ventricular function, further evaluation with echocardiography and/or cardiac catheterization should be considered. Imaging of the heart may be difficult immediately after surgery because of limited transthoracic access and acoustic windows (e.g., due to chest tubes). During transthoracic echocardiography, it is important that hemodynamics are closely observed, because inadvertent pressure applied with the transducer may adversely affect filling pressures and mechanical ventilation. Similarly, vigorous antegrade flexion of a transesophageal echocardiography probe may induce pulmonary hypertension by altering left atrial filling due to partial obstruction of a mainstem bronchus. Although surgery may be routine for many uncomplicated defects such as ASD closure, the approach for more complex intracardiac repairs may cause specific postoperative problems. For example, if a right ventriculotomy is performed to close the VSD in a patient with TOF, RV dyskinesia and poor contraction may be apparent. On the other hand, if a transatrial approach had been used to close the VSD in the same patient, the risk for atrioventricular valve injury or dysrhythmias, such as junctional ectopic tachycardia and heart block, is increased. Often unexpected findings or technical difficulties at the time of surgery mean that modifications to the approach or procedure are necessary. A difficult procedure may lead to a longer time on CPB or additional traction on cardiovascular structures. Failure of adequate hemostasis may expose the patient to significant volumes of transfused blood products, and if there is inadequate drainage via chest tubes placed at the time of surgery, the risk for cardiac tamponade is significant. This may be an acute event, but more commonly, it is evident by progressive hypotension with a narrowing pulse width, tachycardia, an increase in filling pressures, and reduced peripheral perfusion with possible evolving metabolic acidosis. A sudden decrease in chest tube drainage (e.g., caused by an obstructive clot) should raise the suspicion for the potential of tamponade. This is primarily a clinical diagnosis, and treatment (i.e., opening of the sternum and potential need for chest washout and/or clot removal) should not be delayed while waiting for echocardiographic confirmation. Myocardial ischemia from inadequate coronary perfusion is often an under‐appreciated event in the postoperative pediatric patient. Nevertheless, there are a number of circumstances in which ischemia may develop, compromising ventricular function and CO. Myocardial ischemia may occur intraoperatively because of problems with cardioplegia delivery or insufficient hypothermic myocardial protection, and from intracoronary air embolism. In the CICU setting, mechanical obstruction of the coronary circulation is usually the cause of myocardial ischemia rather than coronary vasospasm. Examples include extrinsic compression of a coronary artery by an outflow tract conduit, annulus of a prosthetic valve or chest tube, and kinking or distortion of a transferred coronary artery button. While electrocardiogram (ECG) changes may indicate ischemia (e.g., ST‐segment abnormalities), a sudden increase in left atrial pressure or sudden onset of a dysrhythmia such as ventricular fibrillation or complete heart block may be an earlier warning sign. The effects of prolonged CPB relate in part to the interactions of blood components with the extracorporeal circuit. This is magnified in children due to the large bypass circuit surface area and priming volume relative to patient blood volume. Humoral responses include activation of complement, kallikrein, eicosinoid, and fibrinolytic cascades; cellular responses include platelet activation and an inflammatory response, with an adhesion molecule cascade stimulating neutrophil activation and release of proteolytic and vasoactive substances [46–49]. The clinical consequences can include increased interstitial fluid, generalized capillary leak, and potential multiorgan dysfunction. Total lung water is increased with an associated decrease in lung compliance and increase in A‐aO2 gradient; some of these effects can be attenuated by hemofiltration or modified hemofiltration in the operating room (OR) [50, 51]. Myocardial edema results in impaired ventricular systolic and diastolic function. A secondary fall in CO by 20–30% is common in neonates in the first 6–12 hours following the arterial switch operation, contributing to decreased renal function and oliguria [52]. Sternal closure may need to be delayed due to mediastinal edema and associated cardiorespiratory compromise when closure is attempted. Ascites, hepatic congestion, and bowel edema may affect mechanical ventilation as well as intestinal function (e.g., a prolonged ileus causing a delay in enteral feeding). A coagulopathy post‐CPB may contribute to delayed hemostasis. The clinical manifestation of some of these issues may be attenuated with the lower priming volumes and higher hematocrits used on modern bypass circuits (see Chapter 12 for a detailed discussion of the inflammatory response to CPB). The ECG is an essential component of the initial postoperative evaluation because the CICU team must identify whether the patient is in sinus rhythm early in the recovery period. If the rhythm cannot be determined with certainty from a surface 12‐ or 15‐lead ECG, temporary epicardial atrial pacing wires, if present, can be used with the limb leads to generate an atrial ECG [53]. Also, right and left atrial waveforms are useful in diagnosing atrioventicular synchrony (see Chapter 13). Temporary epicardial atrial and/or ventricular pacing wires are routinely placed in many patients to allow mechanical pacing if sinus node dysfunction or heart block should occur in the early postoperative period. Because atrial wires are applied directly to the atrial epicardium, the electrical signal generated by atrial depolarization is significantly larger and thus easy to distinguish compared with the P‐wave on a surface ECG. Sinus tachycardia, which is common and often secondary to medications (e.g., sympathomimetics), pain and anxiety, or diminished ventricular function, must be distinguished from a supraventricular, ventricular, or junctional tachycardia. Any of these tachyarrhythmias can lower CO by either compromising diastolic filling of the ventricles or depressing their systolic function [54, 55]. High‐grade second‐ and third‐degree (or complete) heart block can diminish CO by producing either bradycardia or loss of atrioventricular synchrony or both. Third‐degree block is transient in approximately one‐third of cases. If it persists beyond postoperative days 9–10, it is unlikely to resolve, and a permanent pacemaker is indicated (see Chapter 22) [56]. The diagnosis of insufficient preload is usually made by monitoring the mean atrial pressure or central venous pressure. The most common cause in the CICU is hypovolemia secondary to blood loss from postoperative bleeding. Initially after surgery and CPB, the filling pressures may be in the normal range or slightly elevated, but this often reflects a more centralized blood volume secondary to peripheral vasoconstriction and venoconstriction following hypothermic CPB. As the patient continues to rewarm and vasodilate in the CICU, additional intravascular volume may be necessary to maintain an adequate circulating blood volume and preload. There may also be significant third‐space fluid loss in neonates and small infants who manifest the greatest systemic inflammatory response following CPB. The “leaking” of fluid from vessels into serous cavities (e.g., ascites) and the extracellular space (peripheral edema that can progress to anasarca) requires that these patients receive close monitoring and volume replacement to maintain an adequate circulating blood volume. Patients with a hypertrophied or poorly compliant ventricle, and those with lesions dependent on complete mixing at the atrial level, also often require additional preload in the early postoperative period. Elevated afterload in both the pulmonary and systemic circulations frequently follows surgery with CPB [52, 57]. Excessive afterload in the systemic circulation (elevated SVR) results in diminished CO and is typically manifest by decreased peripheral perfusion and cool extremities. Treatment of elevated SVR includes recognizing and improving conditions that exacerbate vasoconstriction (e.g., pain and hypothermia) and administering a vasodilating agent. A vasodilator, which can be a phosphodiesterase inhibitor (e.g., milrinone), a nitric oxide donor (e.g., nitroprusside), a calcium channel blocker (e.g., nicardipine), or a peripheral α‐receptor antagonist (e.g., phenoxybenzamine or phentolamine), can be added to an inotropic agent such as epinephrine to augment CO. Neonates, who tolerate increased afterload less well than older infants and children, appear to derive particular benefit from afterload reduction therapy [58–60]. Because decreased myocardial contractility occurs frequently after reparative or palliative surgery with CPB, pharmacologic enhancement of contractility is used routinely in the CICU. Before initiating treatment with an inotrope, however, the patient’s intravascular volume status, serum‐ionized Ca2+ level, and cardiac rhythm should be considered. Inotropic agents enhance CO more effectively if preload is adequate, so IV colloid or crystalloid administration should be given if preload is low. If hypocalcemia (normal serum‐ionized Ca2+ levels are 1.14–1.20 mmol/L) is detected, supplementation with IV calcium gluconate or calcium chloride is appropriate, because Ca2+ is a potent positive inotrope itself, particularly in neonates and young infants. Supranormal supplementation of calcium should be avoided, however, as increased levels of diastolic myocardial calcium are potentially cytotoxic [61]. Dopamine is often the first‐line agent used to treat either mild hypotension (10–20% decrease in normal mean arterial blood pressure for age) or moderate hypotension (20–30% decrease in normal mean arterial blood pressure for age). This sympathomimetic agent promotes myocardial contractility by elevating intracellular Ca2+, both via direct binding to myocyte β1 adrenoceptors and by increasing norepinephrine levels. Dopamine is administered by a constant infusion because of its short half‐life, and a usual starting dose for inotropy is 5 μg/kg/min. At a dose >5 μg/kg/min, dopamine should ideally be infused through a central venous catheter to avoid superficial tissue damage if extravasation were to occur. The dose is titrated to achieve the desired systemic blood pressure, although some patients, especially older children and adults, may develop an undesirable dose‐dependent tachycardia. The challenge with dopamine is its simultaneous effects on β, α, and dopaminergic receptors, often resulting in undesired rhythm disturbances despite maintaining an adequate blood pressure [62]. For this reason, low‐dose epinephrine, up to 0.05 μg/kg/min, is also a useful first‐line inotropic agent, and it may have fewer arrhythmogenic side effects than dopamine in pediatric patients. If a patient does not respond adequately to dopamine up to 10 μg/kg/min or has severe hypotension (more than 30% decrease in mean arterial blood pressure for age), treatment with epinephrine should be considered. Epinephrine may also be used as a first‐line agent to treat mild‐to‐moderate hypotension. It should be given exclusively via a central venous catheter. A typical starting dose is 0.01–0.05 μg/kg/min with subsequent titration of the infusion to achieve the target systemic blood pressure. At high doses (i.e., ≥0.2 μg/kg/min), epinephrine can produce significant renal and peripheral vasoconstriction, tachycardia, and excessive myocardial oxygen demand (chronotropic and vasopressor effects) [58]. Patients with severe ventricular dysfunction who require persistent or escalating doses of epinephrine >0.15–0.2 μg/kg/min may benefit from opening of the sternum and/or should be evaluated for the possibility of mechanical circulatory support with a ventricular assist device (VAD) or extracorporeal membrane oxygenation (ECMO). Norepinephrine is a direct‐acting α agonist that primarily causes arteriolar vasoconstriction, but it also has positive inotropic actions. At doses of 0.01–0.2 μg/kg/min, it can be considered in patients with severe hypotension and low SVR (e.g., “warm” or “distributive” shock), inadequate coronary artery perfusion, or inadequate pulmonary blood flow with a systemic‐to‐PA shunt. It is also useful in patients with preserved systemic ventricular function but failing or inadequate RV contractility (PVOD or postoperative TOF with restrictive RV physiology). In patients with normal hearts, variation in afterload is accommodated by varying stroke volumes and contractility; the normal heart works at peak efficiency and contractility exceeds afterload. This increased contractility is also transferred to the RV through the shared intraventricular septum. The increased afterload also improves the filling characteristics of the LV (by shifting of the interventricular septum) and augments RV coronary perfusion (especially in systemic RV pressure where the RV is now dependent exclusively on diastolic aortic root pressure for coronary perfusion). In the failing heart and in patients with limited ventricular reserve, an increase in afterload may not be matched by an increase in contractility, with a resultant fall in CO. There can be a decrease in responsiveness to increasing doses of catecholamines over time; vasopressin at a dose of 0.1–0.5 mu/kg/min is a potent vasopressor that may help improve the hemodynamics in advanced shock without compromising cardiac function or other adverse sequelae [63]. A combination of epinephrine at lower doses (e.g., <0.1 μg/kg/min) or dopamine with an IV afterload reducing agent, such as milrinone, can help support patients with significant ventricular dysfunction accompanied by elevated afterload. Milrinone inhibits cyclic nucleotide phosphodiesterase III, thereby increasing cAMP, which results in an increased intracellular calcium concentration. In addition to acting as a positive inotrope, increased cAMP relaxes the systemic vasculature, leading to a decrease in afterload as well as a decrease in PVR [59]. The use of milrinone after neonatal and pediatric cardiac surgery has increased significantly over the past 15 years, in part due to the results of a double‐blind, placebo‐controlled multicenter trial that demonstrated a significant decrease in the incidence of LCOS (milrinone dose 0.75 mcg/kg/min) compared to placebo after infant and pediatric corrective heart surgery [42]. A more recent meta‐analysis evaluating the use of prophylactic milrinone in children undergoing surgery for congenital heart disease found that while there is some evidence it may lower the risk for LCOS, there is currently insufficient evidence to conclude that milrinone helps prevent LCOS or mortality [64]. Patients who have the clinical features of relative adrenal insufficiency may benefit from stress steroid therapy [65, 66]. This is primarily a clinical finding of poor vascular tone with persistent hypotension and volume requirement that is refractory to increasing inotrope and/or vasopressor support. The serum cortisol level may be low or show a limited response to adrenocorticotropic hormone stimulation testing [67]; however, the ranges of normal have not been established for pediatric patients after cardiac surgery, in particular for newborns and infants. There is no consistent correlation between serum cortisol level and the incidence of LCOS; nevertheless, stress doses of hydrocortisone (50 mg/m2/day) have been associated with increased systemic blood pressure and lower inotrope scores, although they have not been definitively demonstrated to improve eventual survival. A recent randomized controlled clinical trial also demonstrated that prophylactic, postoperative hydrocortisone infusion reduces the prevalence of LCOS after neonatal CPB [68]. The increased risk for infection and poor wound healing associated with stress dosing of steroids has led to a recommendation for shorter periods of administration (e.g., over 3–5 days) rather than continuing with a long taper [69]. Hypothyroidism is another cause for a persistent LCOS after cardiac surgery. Triiodothyronine (T3) levels have been demonstrated to be low after CPB and may remain low for up to 5 days after surgery, particularly if a sick euthyroid state develops and there is decreased conversion of thyroxine (T4) to the biologically active T3 in peripheral tissues [70, 71]. A pediatric randomized controlled trial evaluating protocolized treatment with T3 (two doses of 0.4 μg/kg during surgery and three doses of 0.2 μg/kg at 3, 6, and 9 hours after cross‐clamp release) demonstrated improvements in ejection fraction and time to extubation, specifically in patients younger than 5 months [72]. Sternal closure following cardiac surgery can restrict cardiac function and also interfere with efficient mechanical ventilation. This is particularly important for neonates and infants, in whom considerable capillary leak and edema can develop following CPB, and in whom cardiopulmonary interactions have a significant impact on immediate postoperative recovery. In the OR, mediastinal edema, unstable hemodynamic conditions from both systolic and diastolic dysfunction, and ongoing bleeding are indications for delayed sternal closure, although it may also be considered semi‐electively for patients in whom hemodynamic or respiratory instability is anticipated in the immediate postoperative period (e.g., following a modified Norwood procedure for HLHS). Urgent reopening of the sternum in the CICU following surgery is associated with higher mortality compared with leaving the sternum open in the OR [73], and successful sternal closure can be achieved for most patients by postoperative day 4 with a low risk of surgical site infection [74]. Patients in whom this cannot be achieved and who have additional risk factors (e.g., neonate, need for ECMO, modified Norwood procedure) have an 11% superficial sternal infection (SSI) rate and significantly greater mortality [75]. Additional discussion of hemodynamic management is presented in Chapter 21.
CHAPTER 36
Cardiac Intensive Care
Introduction
Pathophysiology of specific congenital cardiac defects and implications
Intercirculatory mixing, complete mixing, and streaming
Intercirculatory mixing lesions
Complete mixing lesions
Streaming
Shunts
Increased pulmonary blood flow
Simple shunt
Acyanotic
Cyanotic
Two ventricles
ASD
D‐TGA/VSD
VSD
PA/VSD
CAVC
DORV
Single ventricle
TA ± TGA
HLHS
DORV/MA
Norwood procedure
BT shunt
AP connection
PDA
PA/MAPCA
Truncus arteriosus
AP window
Complex shunt
Clinical consequences of increased pulmonary‐to‐systemic blood flow ratio
Decreased pulmonary blood flow
Target systemic oxygen saturation level
Outflow obstruction
Etiology
Considerations
Low FiO2
Inappropriately low‐set oxygen concentration for delivery
Failure of oxygen delivery device
Pulmonary vein desaturation
Impaired diffusion
Alveolar process: edema, infection
Restrictive process: effusion, atelectasis, pneumothorax
Intrapulmonary shunt
RDS
Pulmonary AVM
PA‐to‐PV collateral vessel(s)
Reduced pulmonary blood flow
Anatomic RV outflow obstruction
Anatomic pulmonary artery stenosis
Increased PVR
Atrial‐level right‐to‐left shunt
RV hypertension
Restrictive RV physiology (low compliance)
Severe tricuspid regurgitation
Large fenestration (modified Fontan operation)
Intra‐atrial baffle leak
Reduced aorta pulmonary shunt flowVentricular‐level right‐to‐left shunt
RV hypertension and residual VSD
Low dissolved O2 content (SV and TGA physiology)
Low mixed venous oxygen level
Increased O2 extraction: hypermetabolic state
Decreased O2 delivery: low cardiac output state
Anemia
Airway and ventilation management
Airway management
Mechanical ventilation
Cardiorespiratory interactions
Influence of lung volume
Afterload
Preload
Pulmonary ventricle
Elevated
Effect: ↑ RVEDp
↑RVp
↓Antegrade PBF
↑ PR and/or TR
Reduced
Effect: ↓ RVEDV
↑ RAp
Systemic ventricle
Reduced
Effect: ↓ LVEDp
↓ LAp
↓ Pulmonary edema
Reduced
Effect: ↓ LVEDV
↓ LAp
Hypotension
Influence of intrathoracic pressure
Right ventricle
Left ventricle
Positive end‐expiratory pressure
Alternative modes of ventilation and respiratory support
Early extubation
Weaning from mechanical ventilation
Restrictive defects
Residual cardiac defects
Restrictive pulmonary defects
Airway
Metabolic
Fluids and nutrition
Analgesia and sedation
Sepsis
Airway
Myocardial dysfunction and hemodynamic monitoring
Assessment of cardiac output
Physical examination
Bedside monitoring data
Laboratory and radiographic data
Surgical factors
Residual or unrecognized defects
Increased atrial pressure
Decreased atrial pressure
Complications related to surgery
Cardiopulmonary bypass and the systemic inflammatory response
Dysrhythmias
Low preload
High afterload
Decreased myocardial contractility
Delayed sternal closure
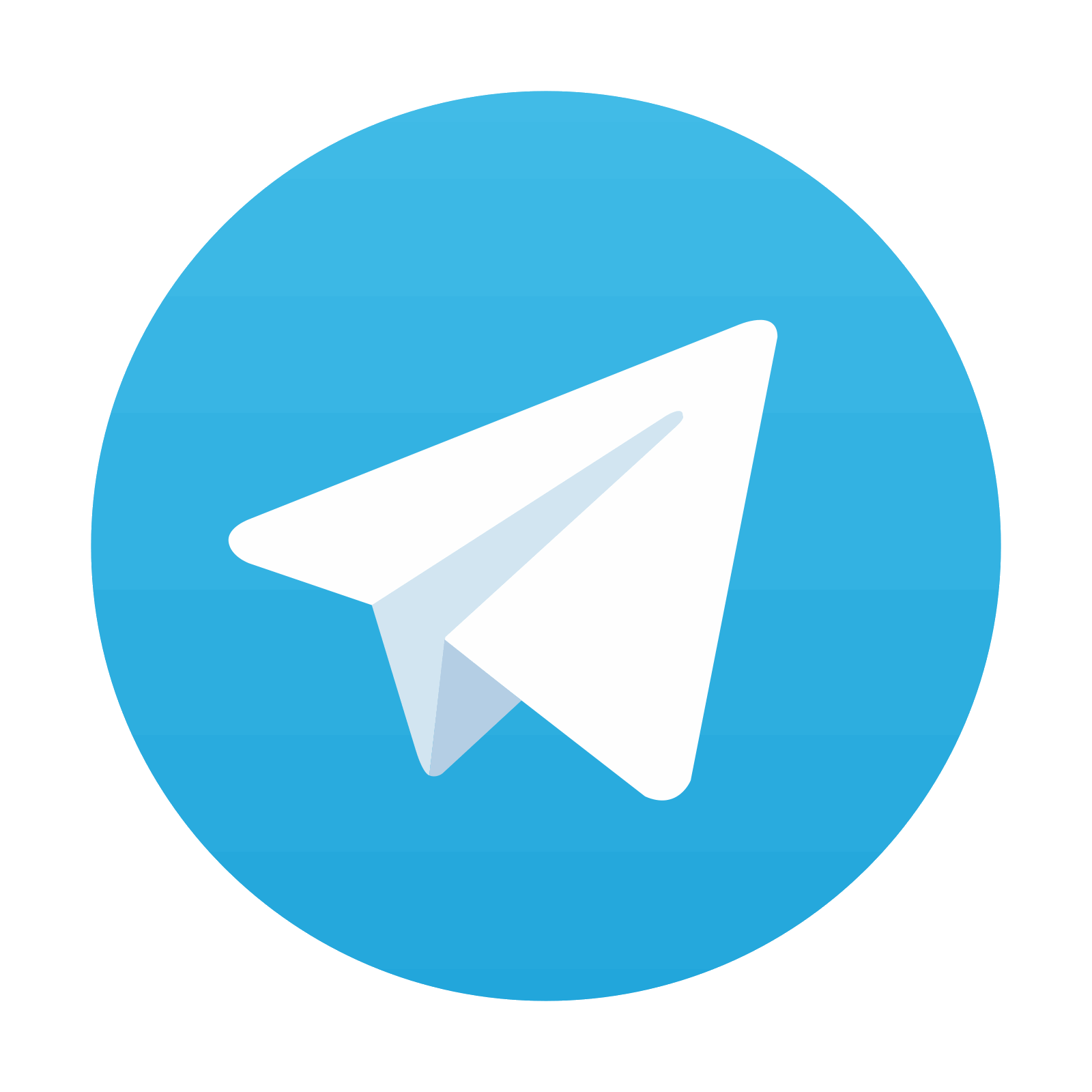
Stay updated, free articles. Join our Telegram channel
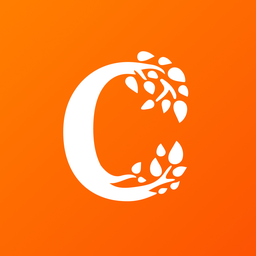
Full access? Get Clinical Tree
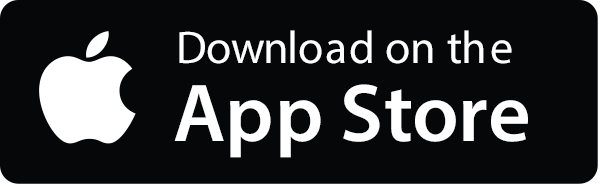
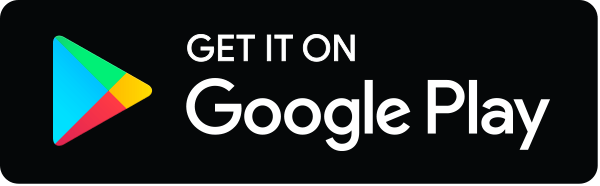