Javier J. Lasa1, Daniel Stromberg2, Sai S. Raju3, and Timothy P. Welch4 1 Cardiovascular Intensive Care Unit, Texas Children’s Hospital, Baylor College of Medicine, Houston, TX, USA 2 Dell Children’s Medical Center of Central Texas, The University of Texas at Austin Dell Medical School, Austin, TX, USA 3 Dell Children’s Medical Center of Central Texas, The University of Texas at Austin Dell Medical School, Austin, TX, USA 4 Arthur S. Keats MD Division of Pediatric Cardiovascular Anesthesiology, Texas Children’s Hospital, Baylor College of Medicine, Houston, TX, USA Although one of the most severe of adverse events related to anesthesia, cardiac arrest (CA) in the perioperative setting occurs infrequently, with rates of anesthesia‐related CA during pediatric anesthesia in the general population reported between 0.14 and 0.8% of anesthetics [2–4]. Significant variability in anesthesia‐related CA rates exists across the pediatric age and illness category spectrum; yet, there is clear evidence to support higher risks of periprocedural CA for patients with underlying CHD [5]. Children with CHD frequently undergo diagnostic and interventional procedures that require sedation or general anesthesia with over 45,000 noncardiac [6] and over 25,000 cardiac [7] surgical procedures performed every year across North America. In spite of these yearly procedural volumes for the CHD population, our understanding of the epidemiology and outcomes after anesthesia‐related CA remains limited to historical reports from CA registries and single‐center case series [6, 8, 9]. Clear limitations exist with volunteer registry reporting, including potential underreporting of events, which limits our understanding of true incidence as well as the risks associated with anesthesia during pediatric cardiac surgery, cardiac catheterizations, and noncardiac procedures in the CHD population. Over the last three decades, several single and multicenter retrospective cross‐sectional efforts have provided a basic foundation for our understanding of the incidence and potential risk factors associated with anesthesia‐related CA in the CHD population. The Pediatric Perioperative Cardiac Arrest (POCA) registry, formed in 1994 to study the etiology and outcomes after perioperative CA in anesthetized children, has published two reports that focused on anesthesia‐related CA which spanned the first 10 years of this registry [2, 8]. Of 373 cases of anesthesia‐related CA reported to the registry between 1994 and 2005, 34% (n = 127; acquired conditions included cardiomyopathy, pulmonary hypertension, arrhythmias, status‐post heart transplantation) were in children with congenital and/or acquired heart disease, of which 92% were in children with the American Society of Anesthesiologists (ASA) physical status III–V compared to 62% in children without heart disease. Of note, 47% of all CAs in children with CHD and acquired lesions occurred at the age of 6 months or younger, and 70% occurred in children aged ≤2 years. A slight majority of CAs occurred in the general operating room (OR) setting (54%) as compared to the cardiac OR (33%), cardiac catheterization laboratory (17%), and other imaging suites (3%). Again, younger age (<6 months old) was more commonly seen in the cardiac OR CA population (76%) as compared to general OR (38%) or catheterization laboratory (35%) [8]. In children with heart disease, those with single ventricle composed 19% of CAs, closely followed by patients with left‐to‐right shunts (e.g. VSD, PDA, AV canal, combined lesions; 18%), left‐sided obstructive lesions (e.g. aortic stenosis, coarctation of the aorta; 16%), acquired conditions (13%), Tetralogy of Fallot (12%), Truncus arteriosus (5%), and miscellaneous conditions (e.g. pulmonary hypertension, status‐post heart transplant, arrhythmias; 18%). Overall mortality in the heart disease CA population was 33%, with the highest mortality in left‐sided obstructive lesions and cardiomyopathy. Resuscitation details available for 68 patients with congenital and/or acquired heart disease revealed few instances of the use of extracorporeal cardiopulmonary resuscitation (E‐CPR; n = 3), which would suggest lack of availability of this resource in many centers in the 1990s and early 2000s. Current evidence suggests that patients with underlying cardiac disease are the highest utilizers of E‐CPR [10], with an epidemiologic report of CA in the cardiac intensive care unit (CICU) from the Pediatric Cardiac Critical Care Consortium (PC4) reporting 27.2% of all CAs occurring in participating CICUs undergoing E‐CPR [11]. Amongst single‐center reports, Boston Children’s Hospital has maintained an extensive registry of CHD patients anesthetized in cardiac operating room and catheterization laboratories with reported frequencies of 0.79% (during cardiac surgery) and 0.96% (during cardiac catheterizations) [4, 12]. Within the cardiac catheterization laboratory, recent efforts to evaluate outcomes after risk adjustment have led to the inclusion of CA as a part of a larger group of severe adverse events (AEs) [13, 14]. These efforts have confirmed the findings by Odegard et al. that CA occurs infrequently during pediatric diagnostic and interventional catheterizations (0.8‐1.6%). Furthermore, in an analysis of the American Heart Association (AHA)’s Get With the Guidelines‐Resuscitation registry of in‐hospital cardiac arrest (IHCA) across 33 pediatric hospitals between 2005 and 2016, Lasa et al. described results of CA occurring in the cardiac catheterization laboratory by evaluating survival to hospital discharge and favorable neurologic outcomes [15]. Of 19,957 pediatric IHCA events, only 203 index CAs were reported to the registry as occurring within the cardiac catheterization laboratory. The survival to discharge rate for the overall cohort was 69% (141/203), of which the majority (69%) of deaths were observed in children <1 year of age. Since serving as a co‐sponsor for the inaugural gathering of resuscitation researchers and healthcare providers at the Conference on Standards and Guidelines for Cardiopulmonary Resuscitation and Emergency Care in 1966, the AHA has continued to publish guidelines on the performance of CPR for both adult and pediatric patient populations. From 2015, the process of 5‐year updates was transitioned to an online format to allow significant changes in the science to be reviewed in an expedited manner and then incorporated directly into the guidelines if deemed appropriate. AHA has released the latest 2020 guidelines with 491 updated recommendations for adult, pediatric and neonatal life support. For the purpose of the pediatric advanced life support guidelines, pediatric patients are infants, children, and adolescents up to 18 years of age, excluding newborns. Infant guidelines apply to infants younger than 1 year of age. Child guidelines apply to children from approximately 1 year of age until puberty. For those with signs of puberty and beyond, adult basic life support guidelines should be followed. Although pediatric IHCA outcomes have improved steadily since 2004, similar gains are not being seen in out‐of‐hospital cardiac arrest (OHCA). Much of the variation in survival rates is thought to be due to the strength of the chains of survival, the critical actions that must occur in rapid succession to maximize the chance of survival from CA (see Figure 25.1) [16]. A separate OHCA chain of survival has been created to distinguish the differences between OHCA and IHCA. In both the chains, a sixth link has been added to stress the importance of recovery [16]. The major new and updated recommendations in 2020 can be seen in Table 25.1 [16]. Rapid recognition of CA, immediate initiation of high‐quality chest compressions, and delivery of effective ventilations are critical to improve outcomes from CA. The 2020 AHA recommendations for high‐quality CPR can be seen in Table 25.2 [16]. New changes to ventilation rates include increases in recommended respiratory rates to 20–30 breaths/min for infants and children with an advanced airway during CPR or for those requiring rescue breathing with a pulse. The Pediatric Cardiac Arrest Algorithm as updated in the AHA 2020 Guidelines is also available in Figure 25.2 [16]. Figure 25.1 Pediatric chains of survival for out‐of‐hospital (A) and in‐hospital (B) cardiac arrest. (Source: Topjian et al. [16]. Reproduced with permission from Wolters Kluwer Health, Inc.) Table 25.1 Key CPR guidelines recommendations and differences noted between 2010 and 2020 publications Source: Topjian et al. [16]. Reproduced with permission from Wolters Kluwer Health, Inc. Table 25.2 Current CPR guidelines recommendations for all age groups Source: Data from Topjian et al. [16]. For infants, single rescuers should compress the sternum with two fingers or two thumbs placed just below the intermammary line. For two rescuers, the two‐thumb encircling hands technique is recommended. If the rescuer cannot physically encircle the victim’s chest, the chest can be compressed with two fingers. If the rescuer is unable to achieve the guideline‐recommended depth, it may be reasonable to use the heel of one hand. For children, it may be reasonable to use either a one‐ or two‐hand technique to perform chest compressions. For OHCA, studies show similar rates of survival to discharge when comparing advanced airway interventions such as supraglottic airway or endotracheal tube with bag‐mask ventilation. Similarly, early placement of an invasive airway after IHCA was also not associated with improvement in survival to hospital discharge [17]. For IHCA there is insufficient evidence as of now to make any recommendation about advanced airway management [18]. The new guidelines emphasize administration of epinephrine within 5 minutes from the start of chest compressions with repetition every 3–5 minutes until the return of spontaneous circulation (ROSC) is achieved. For shock refractory ventricular fibrillation (VF)/pulseless ventricular tachycardia (pVT), either amiodarone or lidocaine can be used. Routine use of sodium bicarbonate and calcium is not supported by the current data unless their administration is indicated in specific circumstances such as electrolyte imbalances and certain drug toxicities. Table 25.3 lists pharmacologic therapy, indications, and precautions for resuscitation. Defibrillation is the definitive treatment of VF/pVT. An initial dose of 2 Joules per kilogram (J/kg) is recommended followed by an increase in the subsequent dose to 4 J/kg for the second shock. For subsequent shocks, higher energy levels may be considered but not to exceed 10 J/kg or the maximum dose for adults. Resuscitation in this population carries an added risk to healthcare workers as the chest compressions, positive pressure ventilation, and establishment of an advanced airway generate aerosols that can remain suspended in the air with a half‐life of ~1 hour. These aerosols can be inhaled by those nearby. To mitigate the risk of exposure to healthcare workers, emphasis should be placed on donning personal protective equipment (PPE) before entering the operation room/scene and limiting personnel in the room to only those essential for patient care. If an advanced airway is considered, pause chest compressions for intubation and use the intubator with the highest likelihood of first‐pass success. Intubate patients with a cuffed tube and connect the endotracheal (ET) tube to a ventilator with a high‐efficiency particulate air (HEPA) filter when available [20]. Protection against inhalation of the aerosolized virus with N95 mask or equivalent, eye protection, gown, and double gloving is important for suspected or confirmed COVID‐19 infection. Figure 25.2 Pediatric cardiac arrest algorithm as updated in the American Heart Association 2020 guidelines. (Source: Topjian et al. [16]. Reproduced with permission from Wolters Kluwer Health, Inc.) Table 25.3 Pharmacology: typical doses and indications Source: Marino et al. [19]. Reproduced with permission from Wolters Kluwer Health, Inc. ECLS indicates extracorporeal life support; FiO2, fractional inspired oxygen; IO, intraosseous; IV, intravenous; JET, junctional ectopic tachycardia; LCOS, low cardiac output syndrome; NAPA, N‐acetylprocainamide; SVR, systemic vascular resistance; SVT, supraventricular tachycardia; VF, ventricular fibrillation; VT, ventricular tachycardia; and WPW, Wolff‐Parkinson‐White syndrome. a The time range for the administration of the loading dose of amiodarone for the child with a perfusing rhythm is slightly longer (i.e. 30–60 minutes) than the pediatric advanced life support (PALS) 2015 recommended time for administration (i.e. 20–60 minutes). The reason for this slight difference is that the child with cardiac disease is likely to be or is at risk for hemodynamic compromise. b For treatment of hypotension or persistent bradycardia with a pulse in the patient with an at‐risk myocardium, give low dose via central administration 1 μg/kg (0.001 mg/kg), which is one‐tenth the standard recommended resuscitation dose for symptomatic bradycardia in PALS 2015. Regardless of the location in which a CA occurs, the provision of high‐quality CPR is highly dependent on rapid, coordinated, team‐based actions that extend throughout the spectrum of care both before, during, and after hypoxic‐ischemic arrest states (no flow/low flow). The likelihood of ROSC, survival to hospital discharge, and neurologic survival are all improved when patients receive high‐quality CPR [16]. Measures of high‐quality CPR include the provision of chest compressions with appropriate depth, rate, and recoil; minimal interruptions in chest compressions (high chest compression fraction); appropriate ventilation and monitoring of adequacy of ventilation; and reversal of treatable causes. Resuscitation science has historically focused on these elements of CPR including logistics and management of chest compressions, assisted ventilation, defibrillation for shockable rhythms, and pharmaceutical reanimation measures. However, recent efforts by the international resuscitation community have extended this focus to include prearrest and postarrest care in light of the reports demonstrating a plateau in survival outcomes for IHCA and OHCA in the pediatric population [21]. Subgroup analyses for outcomes data surrounding anesthesia‐related CA for patients with CHD are limited although care processes across the CA spectrum should not be limited by patient or hospital‐level factors [19]. In order to emphasize the importance of the elements of pre‐/intra‐/post‐arrest care, the 2020 AHA Guidelines for Cardiopulmonary Resuscitation and Emergency Cardiovascular Care report highlighted the importance of each element of care along the CA spectrum by utilizing the Chains of Survival model (see Figure 25.1). Prevention of CA in the in‐patient and/or peri‐anesthetic setting has traditionally been limited to anticipatory management of known high‐risk populations such as those with single‐ventricle CHD before/after Stage 1 repair, suprasystemic pulmonary hypertension, left‐ventricular outflow tract obstruction, and Williams syndrome [5, 22]. Recent quality improvement efforts by the PC4 have involved national implementation of a CA prevention bundle which identified high‐risk populations; standardized anticipatory care plan discussions twice a day; and provided ready access to resuscitation medications, sedation for noxious stimulation, and post‐arrest debriefing tools. Applying this methodology to the periprocedural environment presents challenges surrounding the predominantly nonmodifiable patient and procedure‐based factors that are presenting constant threats during operations and cardiac catheterizations. Ultimately, highly successful specialized teams with specific equipment, knowledge, and resources can rapidly respond to clinical decompensation events in the CHD population with precision and quality. The 2020 updated algorithm and recommendations for the provision of high‐quality CPR are summarized in Figure 25.2. Pediatric CPR is uncommon and thus very challenging to maintain excellent team skills, even in larger tertiary care children’s hospitals. Increasingly, high‐fidelity chest compression and ventilation data have become available to provide real‐time guidance to care teams providing CPR. These CPR feedback devices, when paired with invasive arterial blood pressure and end‐tidal CO2 monitoring, are shifting the paradigm away from provider‐focused CPR training, to one that is patient physiology and hemodynamically focused [23]. Measuring these physiological parameters, which are often available to the anesthesiologist during surgical and/or catheterization procedures, can be used to trend CPR quality, guide therapies during CPR, and gauge likely outcomes like ROC. In retrospective fashion, these data also are available in aggregate to guide quality improvement and resuscitation science efforts. Cardiopulmonary resuscitation that is prolonged and/or resistant to conventional CPR techniques may warrant rescue with mechanical circulatory support should the resources and expertise be available. E‐CPR is defined as the deployment and cannulation onto veno‐arterial extracorporeal membrane oxygenation (VA ECMO) during active conventional CPR. In the pediatric population, this resource‐intense therapy is highly dependent on the complex choreography of resuscitation teams and surgical staff (general/cardiothoracic surgeons) and often utilized in patients with underlying CHD [10]. Historically, E‐CPR began as a specific rescue modality after post‐cardiotomy cardiac arrest/cardiogenic shock in the pediatric CHD population [24, 25]. It has since evolved beyond the postoperative CHD population to include multiple populations of critically ill children including those with acute fulminant myocarditis, acute decompensated heart failure, and sepsis[10]. Activation of E‐CPR teams in the peri‐procedural arena may be less cumbersome than CAs occurring in other inpatient or outpatient settings; yet, excellent communication, multidisciplinary training, and resource allocation for perfusion/priming teams are crucial for effective E‐CPR in the current era [26]. Achieving ROSC with the resolution of no‐flow or low‐flow hemodynamic states has historically been the goal of providers during the high‐stress environment of a CA. However, since 2008, the international resuscitation community has recognized the role of ischemia‐reperfusion injury in the evolution of the disease state known as postcardiac arrest syndrome (PCAS) [27]. Also recognized in the pediatric population, PCAS is composed of several key pathophysiologic components: post‐CA brain injury, post‐CA myocardial dysfunction, systemic ischemia‐reperfusion response, and persistent precipitating pathophysiology [28]. Although a review of the unique pathophysiology of each phase is beyond the scope of this chapter, it is crucial for the cardiac anesthesiologist to consider the post‐return of circulation (ROC) management of the patient with CHD who has suffered a CA as a crucial and equally important time period as active CPR. Figure 25.3 (reproduced with permission from Topjian et al. [19]) provides a standardized approach to help clinicians monitor for ongoing secondary organ injury while also providing them with therapeutic options to address the known pathophysiologic derangements, ultimately providing the best opportunity to mitigate brain and other organ injury post‐arrest. Excellent overviews of postarrest stabilization can be found in the AHA guidelines [29], and a more recent AHA Scientific Statement [19]. The central nervous system is especially sensitive to hypoxic insult and reperfusion injury, and even with expeditious initiation of CPR, ischemic neuronal cell death rapidly occurs. Furthermore, there is a clear time‐weighted burden; with prolonged low‐flow states, irreversible injury is inevitable, and beyond 10–20 minutes, the likelihood of neurologically intact survival is low [30]. Neurologic management after CA, therefore, is focused on identifying actionable lesions, reducing cerebral metabolic demand, and optimizing both global and cerebral oxygen delivery. Postarrest neurologic prognostication is challenged by conflicting evidence and lack of specific biomarkers with high predictive value. Diagnostic neuronal injury patterns may take time to develop, highlighting the general recommendation to avoid neuroimaging for the first 72 hours post‐arrest. However, it is sometimes reasonable to pursue early neuroimaging to characterize the extent of injury, and screen for pathology amenable to medical or surgical intervention, such as space‐occupying hemorrhage or cerebral edema indicating possible intracranial hypertension. In neonates with open fontanelle, this can be initially performed with cranial ultrasound, but in most children, the initial modality of choice will be head computed tomography (CT). It should be noted that while certain findings (e.g., loss of grey‐white differentiation, sulcal effacement, and reversal sign) are unambiguously associated with poor outcome [31], the negative predictive value of a normal scan for survival is only 68% [32]. Ultimately, the most informative prognostic data is obtained from brain magnetic resonance imaging (MRI), which can detect the extent and location of injury patterns, specifically the watershed ischemia/infarct that correlates with poor outcomes [33]. The anesthesiologist will often be called upon to transport and provide supportive care to these patients for imaging studies as well as for potentially urgent/emergent neurosurgical intervention; hence, it is essential to understand the principles of neuroprotective management. Figure 25.3 Post‐cardiac arrest care checklist. Source: Topjian et al. [16]. Reproduced with permission from Wolters Kluwer Health, Inc. Neurologic monitoring is often imprecise and nonspecific, but it can afford the clinician useful data upon which to direct therapy. Electroencephalography (EEG), typically a continuous protocol with video monitoring, has become standard of care in the postarrest phase of intensive care unit (ICU) management. Since as many as half of therapeutically cooled post‐arrest patients will have seizures, most of which are subclinical/nonconvulsive [34], EEG serves an essential ongoing surveillance function. As seizures impose a substantial metabolic burden on an already vulnerable brain and are independently associated with mortality, it is critical to identify and treat them to mitigate secondary injury. Near‐infrared spectroscopy (NIRS) may be used to assess the adequacy of regional blood flow by providing trend data on brain tissue oxygen saturation, a surrogate for oxygen extraction. Monitoring indicators of flow, as opposed to pressure, is crucial after brain injury. Animal data from an experimental swine model has shown that the autoregulatory capacity of the cerebral vasculature, as assessed by NIRS‐derived indices, can be significantly altered following CA [35]. While various cerebral oxygenation saturation threshold targets have been proposed, unique caveats exist in the interpretation of NIRS values in children with CHD [36], as they must be considered in the context of the expected appropriate systemic arterial saturation unique to their specific underlying or palliated physiology (i.e. single ventricle anatomy with systemic to pulmonary artery shunts, cavopulmonary anastomoses, or mixing lesions, among others). Similar to the above is the selection of targets for oxygenation and ventilation, which must often be individualized to cardiac physiology. While hypoxemia is clearly detrimental, hyperoxia may also be deleterious in patients at risk for pulmonary overcirculation with an attendant decline in systemic and brain perfusion. Additionally, despite the cerebral vasodilatory effects of hypercapnia, normocapnia should be the default ventilation target for most patients [28]. In addition to diagnosing and treating nonconvulsive status epilepticus, and optimizing cerebral oxygen delivery, postarrest care is also focused on reducing cerebral metabolic demand through targeted temperature management. Fever/hyperthermia is commonly observed after CA, and aggressive control of fever and maintenance of normothermia with pharmacologic and/or mechanical measures is indicated. While improved seizure control has been shown with therapeutic hypothermia [37], there is equipoise on the superiority of directed therapeutic hypothermia on outcomes, with conflicting evidence from a recent large trial [38]. At present, the best evidence for comatose patients suggests either 5 days of strict normothermia, or alternatively, a 48‐hour period of 32–34 °C hypothermia, followed by 72 hours of normothermia [39]. Like the brain, the myocardium is vulnerable to ischemia‐reperfusion injury, and both the precipitating CA as well as the therapies used during resuscitation can lead to significant ongoing ventricular systolic and diastolic dysfunction [40]. While catecholamines serve a critical role in augmenting coronary perfusion pressure, thereby directly improving the success of resuscitation, their vasopressor effects impose an increased afterload and can lead to myocardial oxygen supply:demand mismatch. Accordingly, excessive attention to inappropriately chosen blood pressure targets may not only lead to excessive or inappropriate resuscitation but also perpetuate ongoing myocardial dysfunction. Advanced hemodynamic monitoring technologies allow the clinician to measure and trend in real‐time more relevant physiologic data on global and regional blood flow, essential to optimal post‐arrest care. While the choice of inotropic, vasopressor, or vasodilator agent is often institution‐ and provider‐dependent, the most appropriate selection can and should be made based on objective and serial hemodynamic assessments. Using such modalities as NIRS, various invasive continuous cardiac output devices [41] and emerging integrative oxygen delivery platforms with predictive analytics [42] can facilitate a more individualized approach and provide early identification of response to therapy and of patients at risk for deterioration (See Chapter 21 for additional discussion of hemodynamic support and monitoring). Despite appropriate and timely goal‐directed and physiologically driven medical hemodynamic support, some patients postarrest will evidence severe and persistent ventricular dysfunction. In this population, veno‐arterial extracorporeal membrane oxygenation (ECMO), either as a bridge to recovery or to more durable long‐term mechanical circulatory support (MCS) such as ventricular assist device (VAD) can be considered. The selection criteria, indications, technical/surgical aspects, and management of the various devices are beyond the scope of this chapter though they are addressed elsewhere in this textbook (See Chapter 37 for a detailed discussion of mechanical support). For a complete discussion of pediatric MCS, the reader is referred to two excellent recent reviews [43, 44]. Some fundamentals, however, warrant mention. First, and germane to the care of patients with CHD, is an understanding of the unique anatomic or physiologic features imposed by the underlying lesion that have implications on cannulation approach, type of device, and degree of support required (i.e. single or biventricular anatomy, among others) [45]. Second, the provision of such resource‐intensive rescue modalities must be reserved for patients with both a reversible cause of arrest and a reasonable probability of favorable neurologic recovery. As mentioned above, this is often a difficult or impossible assessment to make acutely, but there are instances where such support would clearly be futile. Finally, these therapies require significant multidisciplinary, institutional expertise to achieve excellent outcomes [46]. The kidneys are also vulnerable to ischemia during different arrest states. Acute kidney injury (AKI), defined and staged by the Kidney Disease: Improving Global Outcomes (KDIGO) criteria based on creatinine and urine output parameters [47], can manifest following successful resuscitation and is independently associated with mortality [48]. Since early diagnosis may improve outcomes, the identification of predictive biomarkers is an area of active investigation. Urinary neutrophil gelatinase‐associated lipocalin (NGAL) has been shown in post‐cardiopulmonary bypass patients to predict the development of AKI in children and precede creatinine elevation [49], which may be unreliable during dynamic changes in renal function. This and other biomarkers may serve a similar diagnostic or prognostic role after pediatric cardiac arrest, but no conclusive evidence exists currently. There is no specific treatment for AKI; therapies are largely supportive and are aimed at maintaining overall cardiac output and adequate renal perfusion, avoiding fluid overload, and minimizing further secondary renal insult from nephrotoxic drugs. Continuous renal replacement therapy (CRRT) can be initiated whenever the solute and water excretion or acid‐base balance functions of the kidneys become insufficient. The presence of an extracorporeal circuit such as ECMO often allows the hemofilter to be placed as an in‐line shunt, facilitating immediate therapy when clinically indicated [50]; however, most other patients will require placement of a short‐term hemodialysis catheter. Once initiated, the patient’s metabolic status can be tightly controlled, and optimal fluid balance targeted precisely. Several large cohort studies of pediatric CA have examined survival as well as midterm neurodevelopmental outcomes in survivors [11, 21, 51]. In general, cardiac surgical patients fare better than medical patients, and those with biventricular anatomy have better outcomes than those with single ventricle anatomy. Amongst pediatric survivors of IHCA following cardiac surgery, 5‐year survival has been reported as high as 66%, however, with demonstrably worse neurologic outcomes than age‐ and surgical risk‐matched controls. This study also found a linear correlation between both time‐to‐CPR initiation and total CPR duration and outcome [52]. Irreversible injury to the myocardium and kidneys can be managed with mechanical support or dialysis, respectively, and ultimately transplantation if not otherwise contraindicated. Children who suffer catastrophic brain injury following CA, conversely, cannot be salvaged in a similar manner for obvious reasons. Families of these children rightly wish for frank and evidence‐informed prognostication to assist them in making difficult decisions around the maintenance or cessation of life‐sustaining therapies. While neuroimaging has notable shortcomings in this regard, there is emerging interest in identifying serum brain‐specific biomarkers that may predict the outcome. Serum neuron‐specific enolase (NSE), S100b, and myelin basic protein (MBP) are specific to neurons, astrocytes, and myelin, respectively, and have been shown to correlate with mortality and functional neurologic outcome as assessed by the Pediatric Cerebral Performance Category scale [53, 54]. While these markers are not in widespread clinical practice, they may provide clinicians predictive data to guide discussions with families as well as to identify at‐risk patients whose clinical assessment may be obfuscated by neuroactive medication, critical illness, or equivocal imaging findings. Survivors of pediatric cardiac arrest clearly are at risk of severe, permanent, and debilitating adverse neurodevelopmental outcomes. As such, many will need ongoing intensive neurorehabilitation care, and ultimately be rendered technology‐dependent. There are both immediate peri‐arrest and long‐term ethical considerations that this raises, and the defensible choice is not always congruent with the wishes of the family or even the entirety of the medical team. The complex medical and legal factors implicit in this will not be discussed here, but all providers of care to these children must be cognizant of this and strive to treat them and their families with compassion.
CHAPTER 25
Cardiopulmonary Resuscitation in the Patient with Congenital Heart Disease
Epidemiology
Overview of current CPR guidelines
Guidelines/recommendations
2010
2020
Assisted ventilation rate: rescue breathing – in conditions where there is a palpable pulse but inadequate breathing.
Rate of 12–20
(One breath every 3–5 seconds)
Rate of 20–30 breaths/min
(One breath every 3–5 seconds)
Ventilation rate during CPR with an advanced airway
10 breaths/min
(one breath every 6 seconds)
20–30 breaths/min accounting for age and clinical conditions.
Endo‐tracheal tubes (ETT)
Both cuffed and uncuffed ETTs are acceptable for intubating infants and children.
It is reasonable to choose cuffed ETTs over uncuffed ETTs for intubating infants and children.
Cricoid pressure during intubation
Insufficient evidence to recommend
Routine use is not recommended.
Epinephrine administration
Reasonable to administer epinephrine in pediatric cardiac arrest
Reasonable to administer the initial dose within 5 minutes from the start of chest compressions.
Invasive blood pressure monitoring to assess CPR quality
Reasonable for providers to use diastolic blood pressure to assess CPR quality (>25 mmHg in infants, >30 mmHg in children)
Reasonable for rescuers to use diastolic blood pressure to guide CPR quality.
Detecting and treating seizures after ROSC
It is recommended to treat clinical seizures following cardiac arrest
When resources are available, continuous EEG monitoring is recommended for the detection of seizures following cardiac arrest in patients with persistent encephalopathy.
Evaluation and support for cardiac arrest survivors
Rehabilitation services are recommended for survivors. Ongoing neurological evaluation for at least the first year after cardiac arrest.
CPR guidelines
Infant
Children
Adult
Chest compression rate (per minute)
100–120
100–120
100–120
Depth of compression
One‐third of AP diameter of chest
(4 cm/1.5 in.)
One‐third of AP diameter of chest
(5 cm/2 in.)
At least 5 cm but not more than 6 cm (Once children have reached puberty)
Chest recoil
Allow the chest to recoil completely, after each compression
Rhythm check
Lasting no more than 10 seconds, approximately every 2 minutes
Respiratory rate with no advanced airway
Compression to ventilation ratio – single rescuer 30 : 2, two rescuers 15 : 2.
Compression to ventilation ratio – 30 : 2.
Respiratory rate with advanced airway
Reasonable to target respiratory rate of 1 breath every 2–3 seconds (20–30 breaths/min)
Provide breath every 2–3 seconds
CPR techniques
Advanced airway interventions during CPR
Drug administration during CPR
Management of ventricular fibrillation and/or pulseless ventricular fibrillation
Pediatric resuscitation in patients with suspected or confirmed COVID‐19 infection
Medication
Dose
Indications
Cautions/precautions
Adenosine
Initial dose: 100 μg/kg (0.1 mg/kg; maximum single dose: 6 mg) rapid IV push; second dose can be double the first dose (i.e. give 200 μg/kg [0.2 mg/kg] for second dose, maximum 12 mg)
Atrioventricular node–dependent SVT
Adenosine will not effectively terminate atrioventricular node–independent tachycardias, such as atrial flutter, ectopic atrial tachycardia, or atrial fibrillation. Should not be administered for wide QRS complex tachycardia unless it is clear that the underlying rhythm is not atrial fibrillation or atrial flutter with associated antegrade accessory pathway conduction Expert consultation should be obtained before the administration of adenosine as a diagnostic and potentially therapeutic intervention for stable patients who have wide QRS complex tachycardia
Amiodarone
For VF/pulseless VT, in absence of known or suspected long‐QT syndrome: Initial dose: 5.0 mg/kg IV/IO bolus.
Maximum single dose: 300 mg; can repeat to a total of three doses;
total: 15 mg/kg/24 hours; in adolescents, maximum 2.2 g/24 hours
For perfusing atrial or ventricular arrhythmias: Give loading dose of 5 mg/kg over 30–60 minutesa
Maximum single dose: 300 mg; can repeat to a maximum of three doses
Total: 15 mg/kg/24 hours; in adolescents, maximum 2.2 g/24 hours
If the patient is hemodynamically unstable or receiving other medications that lower heart rate, consider lower dose and slower infusion
Shock‐refractory cardiac arrest (VF/pulseless VT); atrial and ventricular arrhythmias; JET
Use lower dose and/or slower infusion if the patient is hemodynamically unstable or receiving other medications that lower heart rate; can cause hypotension; can prolong QT interval:
Atropine
For symptomatic bradycardia: 20 μg/kg (0.02 mg/kg); minimum single dose: 0.5 mg; maximum single dose: 500 μg (0.5 mg)
For emergent preintubation bradycardia prophylaxis: 20 μg/kg (0.02 mg/kg) Intubation: No minimum dose; maximum single dose 500 μg (0.5 mg)
Vagal‐mediated bradycardia; primary atrioventricular block; emergent intubation bradycardia prophylaxis
Loss of constrictive pupillary reflex to light
Calcium chloride (10% = 100 mg/mL = 27.2 mg/mL elemental calcium)
10–20 mg/kg; maximum single dose: 2 g
Hypocalcemia
Precipitates with sodium bicarbonate; rapid IV administration can cause hypotension, bradycardia, or asystole (particularly if the patient is receiving digoxin)
Calcium gluconate (10% = 100 mg/mL = 9 mg/mL elemental calcium)
50–100 mg/kg; maximum single dose: 3 g
Hypocalcemia
Precipitates with sodium bicarbonate; rapid IV administration can cause hypotension, bradycardia, or asystole (particularly if the patient is receiving digoxin)
Dexmedetomidine
0.5–1 μg/kg bolus; infusion 0.25–1 μg/kg/hour; titrate to effect
For sedation and mild analgesia
If at risk for heart block or symptomatic bradycardia, pacing capability should be available; can cause hypotension or bradycardia
Dobutamine
2–20 μg/kg/min
Myocardial systolic dysfunction (i.e. as inotrope and/or vasodilator)
Titrate to effect; can produce hypotension or tachyarrhythmias
Dopamine
2–20 μg/kg/min; if ≥20 μg/kg/min is required, consider using an alternative adrenergic agent
Systolic dysfunction; postoperative LCOS
Titrate to effect; can produce vasoconstriction and hypertension or tachyarrhythmias; increases myocardial oxygen consumption
Epinephrine
For pulseless cardiac arrest: Bolus: 10 μg/kg (0.01 mg/kg, or 0.1 mL/kg of 0.1 mg/mL) concentration during cardiac arrest; maximum dose: 1 mg
For symptomatic bradycardia: Bolus: 10 μg/kg (0.01 mg/kg, or 0.1 mL/kg of 0.1 mg/mL) concentration; maximum dose: 1 mg
For treatment of hypotension or persistent bradycardia with a pulse in the patient with an at‐risk myocardiurnt: Give low dose via central administration 1 μg/kg (0.001 mg/kg); continuous infusion: 0.01–0.2 μg/kg/minb
Cardiac arrest; symptomatic bradycardia; systolic dysfunction; postoperative LCOS
Significant vasoconstriction at higher doses; increases myocardial oxygen consumption
Esmolol
Bolus: 100–500 μg/kg (0.1–0.5 mg/kg) over 1–2 minutes
Infusion: 50–500 μg/kg/min
SVT; hypertension
Can cause bradycardia, hypotension, and/or hypoglycemia
Etomidate
Bolus: 0.2–0.4 mg/kg, over 30–60 seconds; maximum dose: 20 mg
Procedural sedation
Can cause apnea and adrenal suppression
Fentanyl
Bolus: 1–5 μg/kg continuous infusion for neonates and young infants: 0.5–5 μg/kg/hour
Continuous infusion for older infants and children: 1–3 μg/kg/min
Analgesia
Can cause apnea and chest wall rigidity in the naive patient
Furosemide
1 mg/kg; starting dose of up to 10 mg to naive patient
Diuresis
Can cause hypokalemia, hypochloremic metabolic acidosis, or hypotension if preload dependent
Heparin
Presumed shunt occlusion: 50–100 U/kg; anticoagulation before ECLS cannulation: 100 U/kg
Presumed shunt occlusion; ECLS cannulation
Can cause bleeding
Hydrocortisone
1–2 mg/kg; maximum dose: 100 mg
LCOS not responsive to inotropic agents
Can cause hyperglycemia and/or hypokalemia
Isoproterenol
0.05–2 μg/kg/min
Post–cardiac transplantation; long QT with torsade des pointes; primary atrioventricular block
Can cause hypotension, tachycardia, and increased myocardial oxygen consumption
Ketamine
0.5–2 mg/kg
Procedural sedation
Can cause apnea and increased respiratory secretions; myocardial depressant at high doses
Levosimendan
Bolus: 12 μg/kg; infusion: 0.1 μg/kg/min
LCOS
Lidocaine
Bolus: 1 mg/kg. may repeat Infusion: 20–50 μg/kg/min (repeat bolus dose if infusion initiated >15 minutes after initial bolus dose)
VF/pulseless VT cardiac arrest; ventricular arrhythmias
Monitor QTc and lidocaine levels; can cause seizures at high levels
Magnesium sulfate
Pulseless VT with torsade des pointes; bolus: 25–50 mg/kg; maximum dose: 2 g VT with pulses: 25–50 mg/kg over 10–20 minutes; maximum dose: 2 g
VF/pulseless VT arrest with torsade des pointes; hypomagnesemia
Can cause hypotension with rapid administration
Midazolam
0.05–0.2 mg/kg
Sedation
Can cause apnea or hypotension
Milrinone
Bolus: 50 μg/kg, administered over 10–60 minutes
Maintenance infusion: 0.25–1.0 μg/kg/min
LCOS
Hypotension
Nitric oxide
Up to 40 ppm
Increased pulmonary vascular reactivity; pulmonary hypertension ± crisis
Methemoglobinemia
Norepinephrine
Infusion: 0.025–0.3 μg/kg/min
LCOS with low SVR; clinically significant vasodilation
Vasoconstriction; increases myocardial oxygen consumption
Oxygen
FiO2 = 21–100%
Alveolar desaturation
Pulmonary overcirculation with an unrestrictive aortopulmonary shunt
Phenylephrine
Bolus: 5–20 μg/kg; (0.005–0.020 mg/kg) Infusion: 0.1–0.5 μg/kg/min
Cyanotic spell in unrepaired tetralogy of Fallot; symptomatic hypotension because of low SVR; shunt obstruction; coronary hypoperfusion
Can cause vasoconstriction or hypertension
Propofol
Bolus: 1–3 mg/kg
Infusion: 50–100 μg/kg/min
Sedation, amnesia
Hypotension; contraindicated in children with mitochondrial disease and not approved in children for prolonged sedation
Procainamide
Bolus: 15 mg/kg over 30–60 minutes; maximum dose: 100 mg
Infusion: 20–60 μg/kg/min
JET; SVT; atrial fibrillation
Monitor ECG and procainamide and NAPA levels; can prolong QT interval:
Prostaglandin E1
To establish ductal patency: 0.05–0.1 μg/kg/min, IV/IO infusion To maintain ductal patency: 0.01–0.05 to 0.01–0.02 μg/kg/min IV/IO infusion
Maintain patency of ductus arteriosus
Apnea; fever and hypotension
Sodium bicarbonate
Bolus: 1–2 mEq/kg slow IV push; dose adjusted to severity of base deficit; use 4.2% (0.5 mEq/L) concentration for neonates
Metabolic acidosis
Precipitates with calcium
Vasopressin
Infusion: 0.0005–0.01 U/kg/min
LCOS with low SVR; vasodilatory shock; diabetes insipidus
Hypertension and increased afterload; fluid retention
Verapamil
Bolus: 0.1–0.2 mg/kg
Do not administer to infants <12 months of age without expert consultation
SVT
Hypotension; have calcium available; not to be administered to infants (can cause apnea, bradycardia, and hypotension); avoid in patients with WPW
Phases of cardiac arrest
Post‐cardiac arrest care
Neurologic monitoring and targeted neuroprotective management
Management of postarrest myocardial dysfunction
Management of acute kidney injury
Outcomes, prognosis, and follow‐up after cardiac arrest
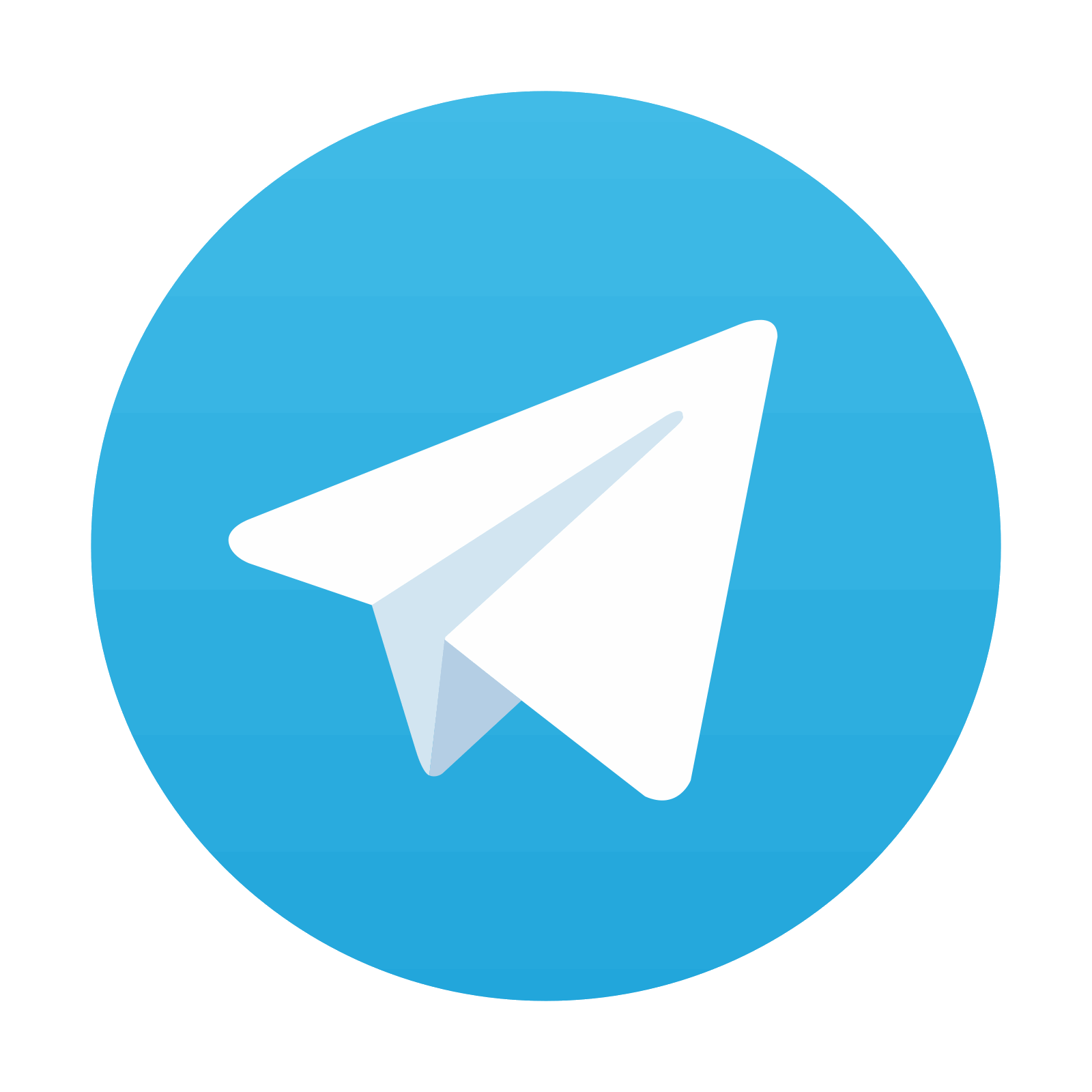
Stay updated, free articles. Join our Telegram channel
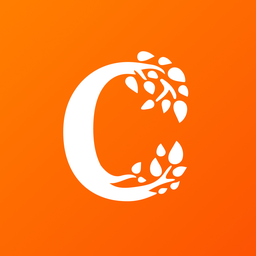
Full access? Get Clinical Tree
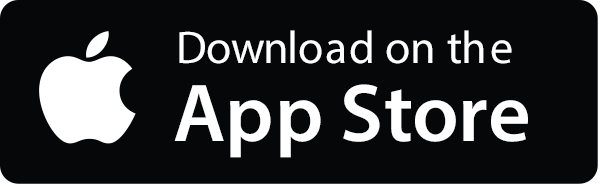
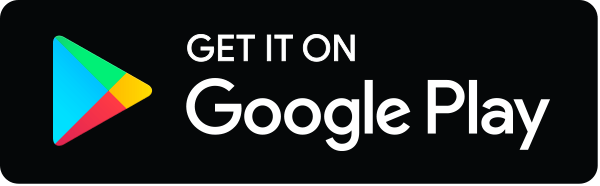