Abstract
Even though practices do differ geographically, general anaesthesia often involves some mode of mechanical ventilation different from normal spontaneous ventilation. This chapter will focus on the differences between these conditions, and describe the problems associated with artificial ventilation.
Introduction
Even though practices do differ geographically, general anaesthesia often involves some mode of mechanical ventilation different from normal spontaneous ventilation. This chapter will focus on the differences between these conditions, and describe the problems associated with artificial ventilation.
Lung Physiology
The lungs deliver O2 and remove CO2, goals that are achieved optimally if ventilation and perfusion are adequately matched. While alveoli are passive ‘soft bags’ that cannot inflate or deflate themselves, their volumes do range from totally collapsed to fully inflated to over-distended. Alveolar volume is the result of the balance between forces that cause alveolar expansion (thoracic cage, muscle activity, nitrogen) and forces that cause alveolar collapse (elastic recoil of the lung, alveolar surface tension, weight of viscera, hyperoxia).
Lung inflation is achieved by an increase in transpulmonary pressure generated by expansion of the thoracic cage by active contraction of the intercostal muscles (thoracic breathing) and the diaphragm (abdominal breathing). Expiration is most often passive, and ceases when the forces that cause the lung to collapse match the forces that cause it to expand. The lung volume at this equilibrium is the functional residual capacity (FRC).
The relative contribution of thoracic or abdominal breathing during inspiration varies according to body position: thoracic breathing predominates in the upright position, and abdominal breathing in the supine position. These differences reflect the fact that the respiratory system tries to minimize the work of breathing [1]. In the upright position, the abdominal muscles are contracted, and a shortened diaphragm has to make a substantial effort against the abdomen, therefore thoracic breathing prevails. In contrast, in the supine position, the abdominal muscles are relaxed and offer limited resistance to diaphragmatic displacement. In addition, the diaphragm is elongated with actin–myosine alignment optimal to generate maximal force.
During expiration in the supine position in the awake patient, diaphragmatic muscle tone impedes cephalic (non-dependent) displacement of abdominal viscera. This muscle tone is lost during anaesthesia, impairing ventilation/perfusion matching (see below).
Anaesthesia, Mechanical Ventilation and the Respiratory System
During anaesthesia, FRC decreases from an average of 3.5 L in the upright position to 2 L in the supine, anaesthetized subject [2]. This decrease is due to a loss of respiratory muscle tone, mainly in the diaphragm, accompanied by a minor decrease of the thoracic perimeter [3]. The increase in pleural pressure and resulting decrease in aerated lung volume renders the lung parenchyma prone to collapse and leads to airway closure. Airway closure during expiration (dynamic airway collapse) may occur in the healthy awake patient, but worsens with factors such as age, obesity or anaesthesia. These closed airways can reopen during inspiration, but some remain closed and cause alveolar resorption atelectasis, a problem worsened by high inspired O2 concentrations (FiO2). Actually, atelectasis is the main problem related to general anaesthesia and mechanical ventilation. It appears in 90% of patients and affects up to 10–20% of the lung during general anaesthesia, regardless of whether spontaneous or mechanical ventilation is used [2]. Ventilation of these atelectatic areas can result in cyclic opening and closing of alveoli, leading to local shear injury called ‘atelectrauma’ [4, 5]. A second problem is barotrauma or volutrauma, which can be occult in patients apparently ventilated without damage. Atelectrauma and volutrauma lead to biotrauma, i.e. biochemical injury or release of cytokines [6]. We will expand on these elements.
Atelectrauma
Atelectasis during anaesthesia is caused by three mechanisms: absorption of alveolar gas, impairment of surfactant function and compression of lung parenchyma [7].
Absorption of alveolar gas can facilitate alveolar collapse. In the awake subject, alveoli are filled with N2, O2, CO2 and H2O vapour, and the partial pressure of every gas helps keep the alveoli open. Nitrogen is an inert gas, and thus there is no net uptake from the alveoli across the alveolocapillary membrane. Therefore, N2 is always present in the alveoli and scaffolds them (‘helps keep them open’). Oxygen, however, is consumed: there is a net flow of O2 into the capillaries that matches O2 consumption of the patient. Therefore, if the alveolus contains high O2 partial pressures without other gases to scaffold the alveoli, the net O2 transfer will cause alveoli to deflate and collapse. This effect is not relevant in the non-dependent (anterior and cephalic) alveolar units with a high ventilation/perfusion (V/Q) ratio, but it is in the dependent (lower and posterior) units with a low V/Q ratio where O2 uptake from the alveolus exceeds delivery to it. If there is complete airway occlusion after the alveoli have been washed with 100% O2, lung units behind this obstruction will have all their O2 removed into the capillary blood and will fully collapse, generating a V/Q unit with absolute shunt.
Surfactant serves to decrease wall tension in the smaller alveoli, preventing them from collapsing. Surfactant function is impaired by inhaled anaesthetics, at least in laboratory studies [7]. In isolated animal lungs, surfactant is released by manoeuvres that increase alveolar size, such as larger tidal volumes or by inflating the lung to total lung capacity [7]. If these effects can be extrapolated to clinical practice, surfactant release could be improved by sighs or recruitment manoeuvres (RM).
Compression of lung tissue is the most important cause of atelectasis. It is caused by an increase of forces compressing the alveoli and a decrease of forces expanding lung units. The main external force is gravity, the effect of which becomes more relevant upon resuming the supine position. Gravity includes the weight of the heart, overweight due to obesity and the weight of abdominal viscera. The weight of abdominal viscera becomes more relevant during anaesthesia, due to the loss of diaphragmatic activity. During spontaneous inspiration, both the anterior and posterior parts of the diaphragm contract, and ventilation will thus be distributed to both the anterior and posterior parts of the lung. During mechanical ventilation, the diaphragm will act as a passive membrane and inspired gases will choose the path of least resistance, which is towards the non-dependent parts of the lungs – the dependent areas receive less ventilation because the dependent parts of the diaphragm bear the full weight of the abdominal contents. Once the volume and compliance of the dependent alveolar units are reduced, a vicious circle is entered of a lower alveolar radius, requiring higher pressures to open these alveoli with poor ventilation (Laplace’s law), causing a further decrease in alveolar volume and compliance, which in turn further worsens ventilation, leading to progressive alveolar collapse.
Atelectrauma is not caused by the atelectasis per se but mainly by the shear stress at the interface of open and closed lung regions [8]. Cyclic opening and closing of alveolar units harms lung parenchyma, mainly because forces acting on lung parenchyma are much higher than the airway pressures. A mathematical model postulated that an airway pressure of 30 cm H2O results in a pressure of 140 cm H2O in the interface between open and closed lung units [9].
Volutrauma
Mechanical ventilation with high airway pressures causes lung damage, called barotrauma [10]. Dreyfuss et al proposed using the term ‘volutrauma’ rather than barotrauma because injury is mainly related to lung deformation by the generated tidal volume (VT). Both barotrauma and volutrauma act simultaneously. Damaging forces related to pressure are defined as stress, and lung deformation related to volume is called strain [11].
High airway pressures (Paw) can only be harmful if they lead to a high transpulmonary pressure, which is the difference between pressure inside the alveolus (PA) and the pressure outside the alveolus, which is pleural pressure (Ppl). Fig. 19.1 displays two different situations. On the left (A), PA is high (40 cm H2O) and Ppl low (5 cm H2O), and the resulting high transpulmonary pressure (35 cm H2O) will cause tissue deformation and injury. In the example on the right (B), PA is also high (40 cm H2O) but so is the ‘external’ pressure Ppl (25 cm H2O), due to gravity, pleural effusion, compression by a CO2 pneumoperitoneum, Trendelenburg position or other causes. Transpulmonary pressure thus will be low (15 cm H2O), so that in this case the high PA will not be harmful. Thus, whenever transpulmonary pressure is high, lung deformation can occur and the lung can be damaged. This happens when high positive pressures are applied but also during spontaneous ventilation with high negative pressure generated during airway occlusion. In both situations, lungs are being damaged [6]. Note that transpulmonary pressure is calculated as PA – Ppl and not as Paw – Ppl. Paw (measured at the mouth) will only match PA (in the alveoli) when there is no airway flow, which, for example, is the case during an end-expiratory pause. This is explained in more detail later.
High VT (strain) can cause volutrauma when it is combined with high airway pressure (stress). Strain is the ratio of VT to the functional residual capacity (FRC) [11]. For the same VT, strain is increased when FRC is low. Because most research on strain has been done in ICU patients with ARDS, these concepts may not exactly apply to the intraoperative setting with low FRC such as obesity or laparoscopy.
Thus, ‘high Paw’ or ‘high VT’ are not synonyms for ‘lung trauma’. High Paw can be observed in a trumpet player, but in this setting expiratory muscles create high intrapleural pressures, therefore transpulmonary pressure is low and there is no barotrauma. Also, a high VT is needed to ventilate a tall basketball player, but this VT will be easily achieved with a low Paw because his FRC will be larger too.
The harmful threshold for stress is around 27 cm H2O and the threshold for strain is twice the FRC volume. Stress/strain control is crucial in patients with ARDS with a ‘baby lung’ [12]. In the operating room, injury related to excessive stress/strain is insignificant in most patients, but should be kept in mind in patients at risk for developing lung injury: those receiving long term ventilation and those likely to experience simultaneous hits on their lungs (see later).
Biotrauma
Biotrauma is the biological reaction to mechanical injury [4]. Damage of lung parenchyma causes the release of white cells and pro-inflammatory cytokines – interleukin (IL) IL-1β, IL-6, IL-8, tumour necrosis factor (TNFα) – resulting in lung inflammation.
Biotrauma is obvious when stress/strain is high enough to break lung structure, causing gross, visible anatomical damage. This is mainly caused by repeated recruitment/derecruitment and/or overdistension of the lung [13]. But biotrauma is also measurable with low-intensity damage caused by prolonged mechanical ventilation, fluid overload, hyperoxia, etc., and especially when their effects are combined.
Multiple-hit Theory
Patients submitted to aggressive surgical procedures are at risk of developing postoperative lung injury due to ‘multiple-hits’ on their lungs in the perioperative phase (Table 19.1) [14]. In the operating room, the lungs may sustain a first injury by sepsis, trauma, infection, or any other harm often related to a surgical problem. These problems are more or less unavoidable. But when we treat the patient in the operating room, we want to avoid second-hits that are under our control yet are also known to cause lung injury: atelectrauma, volutrauma, fluid overload, etc.
First hit | Second hit |
---|---|
|
|
Goals of Ventilation during General Anaesthesia
Intraoperatively, mechanical ventilation serves the same goals as physiological ventilation (O2 delivery and CO2 removal). Even though mechanical ventilation is innocuous in most surgical patients, we must be cautious in those patients at risk of postoperative pulmonary complications (PPC). We have to identify these patients. Table 19.2 shows a list of risk factors for PPC [14]. Predictive scores such as ARISCAT [15] can also be useful to stratify risk. A common example of patient at risk of PPC would be an 80-year-old patient presenting for a prolonged emergency laparotomy for colon perforation.
Table 19.2. Risk factors for postoperative pulmonary complicationsa.
Level of certainty | Patient-related factors | Procedure-related factors | Preoperative testing | |
---|---|---|---|---|
ACP guidelines [19] | Good evidence |
|
| Albumin < 30 g/L |
Fair evidence |
| Transfusion |
| |
Recently identified risk factors or conflicting results | Insufficient evidence |
|
|
a Adapted from Gallart L, Canet J. Post-operative pulmonary complications: Understanding definitions and risk assessment. Best Pract. Res. Clin. Anaesthesiol. 2015; 29 (3): 315–30.
Abbreviations: ACP, American College of Physicians; ASA, American Society of Anesthesiologists; BMI, body mass index; BUN, blood urea nitrogen; COPD, chronic obstructive pulmonary disease; GERD, gastroesophageal reflux disease; Hb, haemoglobin; SpO2, oxygen saturation measured by pulse oximetry.
Intraoperative Oxygenation
The use of a high FiO2 during anaesthesia can induce oxygen toxicity and worsen atelectasis. Oxygen toxicity has been demonstrated in animals but the toxic threshold for human adults is unknown. Pulmonary toxicity due to hyperoxia is related to prolonged exposure and high-stretch mechanical ventilation. Hyperoxia can enhance the lung toxicity of drugs such as bleomycin, adriamicin and amiodarone and possibly also other chemotherapy drugs such as cytotoxic antibiotics, alkylating agents, anti-metabolites, alkaloids or biological response modifiers [16]. On the other hand, some have suggested it may be prudent to avoid high O2 concentrations at the extremes of age, and when vasoconstriction or ischaemia–reperfusion injury is likely to occur (patients at risk of coronary disease, stroke or postoperative cognitive dysfunction) [17].
To consider what the optimal FiO2 in the perioperative period might be, we have to consider three different periods: induction, maintenance and emergence.
During induction, a high FiO2 prior to intubation (i.e. preoxygenation) will increase the time to desaturation during apnoea (e.g. during a dreaded cannot intubate–cannot ventilate scenario). In healthy patients, apnoea time to 90% SpO2 (oxygen saturation measured by pulse oximetry) was 411 sec after preoxygenation with 100% O2, but only 200 sec when preoxygenated with 60% O2 [18]. Thus, routine preoxygenation with a high FiO2 during induction is likely to improve safety. But the downside is an increase in atelectasis. It is widely accepted that at least 80% O2 is needed to cause atelectasis, but this is based on research in healthy subjects [18]. In obese, COPD or elderly patients alveolar collapse probably occurs with lower FiO2. In any case, the use of high FiO2 is recommended during preoxygenation prior to induction, and atelectasis can be treated after intubation with RM and PEEP.
During maintenance of anaesthesia, an FiO2 of at least 30% is used routinely to counteract the effect of V/Q mismatching on oxygenation. Hyperoxia (FiO2 > 80%) to decrease surgical site infection after colorectal surgery has been recommended by Centers for Disease Control (CDC) and World Health Organization (WHO), but this recommendation has generated controversy [19]. A meta-analysis concluded that intraoperative hyperoxia does not increase the risk of atelectasis if PEEP is used [20]. The level of PEEP required is unknown and probably has to be tailored for every patient [21].
During emergence, 100% O2 is commonly used to avoid diffusion hypoxia (Fink effect) for the first five minutes after discontinuing N2O or after liberal use of opioids. However, hyperoxia during emergence and after emergence could induce postoperative atelectasis. Although we use RM and PEEP before extubation, these recruitment tools can no longer be used after extubation, a crucial moment because the lung tends to collapse due to pain induced splinting, hypoventilation and decreased muscle activity. This collapse would be impaired by hyperoxia, thus some authorities recommend not to use hyperoxygenation before extubation [22].
Avoid Atelectrauma: Recruitment and Positive End-expiratory Pressure
The pressure needed to recruit alveoli (=critical opening pressure) is much higher than the pressure needed to keep these alveoli open after they have been recruited [23]. This is dictated by Laplace’s law: the lower the radius of the alveolus, the higher the pressure needed to open it. Once the alveolus is inflated, the radius increases and the pressure needed to keep the alveolus open decreases. Also, the critical opening pressure is not the same for all alveoli: it is higher in the dependent lung zones (posterior and caudal), it is intermediate in patients with obesity, COPD and/or after prolonged mechanical ventilation, and it is higher still in the presence of pulmonary disorders such as oedema, infection or ARDS.
Lachmann [24] stated: ‘open the lung and keep the lung open’, which means RM used to open alveoli have to be followed by PEEP to keep them open: both are needed. If RM are not followed by PEEP, alveoli will collapse again. If PEEP is administered without RM, the pressure will probably be ineffective to open collapsed alveoli.
RM during anaesthesia can be performed by bag squeezing, continuous positive airway pressure (CPAP) or stepwise VT changes, but probably the best systematized method is the cycling manoeuvre: both inspiratory pressure and PEEP are gradually increasing to 20 cmH2O (i.e. a total pressure of 20 + 20 cm H2O) [25]. Once 40 cm H2O is reached, PEEP is progressively decreased and the optimal PEEP value is determined by looking for the lowest PEEP value that still yields the highest increase in compliance (further explained below).
When might RM be used? Intraoperatively, the use of RM + PEEP could be particularly advantageous (1) after intubation, when atelectasis is common; (2) after any disconnection (especially if combined with airway suctioning), because alveoli have been derecruited; (3) before extubation, to generate the best recruitment conditions for the postoperative period; and (4) when adverse conditions for the lung are present, such as prolonged ventilation, obesity, pneumoperitoneum or relative hypoxaemia (i.e. low SpO2 despite high FiO2).
With regard to PEEP, there is no agreement as to the PEEP level that should be used if it is used and as to whether the routine use of intraoperative PEEP affects outcome [21]. While some experts and meta-analysis [26] recommend the routine use of PEEP to reduce PPCs, other studies do not concur this. In the PROVHILO study [27], 12 cm H2O PEEP with RM did not lower the incidence of PPC after open abdominal surgery compared to ≤ 2 cm H2O PEEP without RM. One working hypothesis is that patients might benefit from ‘individualized PEEP’ or ‘optimal PEEP’ as defined in the classic paper of Suter et al [28]. Gradually increasing PEEP will improve oxygenation by decreasing pulmonary shunt and improving lung compliance. As PEEP is being further increased, oxygenation may still be improving, but at some point, both O2 delivery and compliance will deteriorate, the former because heart–lung interactions will decrease cardiac output, the latter because high inflation pressure causes alveolar over-distension. ‘Best PEEP’ therefore is the lowest PEEP that generates optimal O2 delivery and compliance.
The pressure–volume (P–V) curve explains the above concepts as well as some concepts to be discussed later (Fig. 19.2). The P–V curve is S-shaped, and its slope or steepness (ΔP/ΔV) defines the compliance. The curve has three segments: an initial only slightly inclined part (collapse zone), a central steep incline (safe zone), and a final part that again has flattened out (overdistension zone). These segments are connected by a lower inflection point (between the collapse and the safe zones), and an upper inflection point (between the safe and the overdistension zones). The S-shape is pronounced in patients with ARDS but may be almost imperceptible in healthy subjects.
Fig. 19.2 Pressure–volume curve model in patients with mechanical ventilation. The first part of the curve starts from atmospheric pressure. A high pressure is needed to open collapsed alveoli. A lower inflection point indicates the pressure at which alveoli are opened. A second part of the curve shows the ideal zone with best compliance, where high volumes are achieved with low pressures. A third part of the curve, starting at an upper inflexion point, shows the overdistension zone: high pressures scarcely provide changes in volume and risk of barotrauma is increased.
If the lung is positioned in the collapse zone, compliance (the steepness) is low and a high pressure will be needed to open the collapsed alveoli. Atelectrauma is bound to result from opening and closing alveoli if the lung is being ventilated in this area of the P–V curve. The lower inflection point indicates the airway pressure at which alveoli start to open. In the central safe zone with the steep slope, compliance has increased (improved) because alveoli are open and they are easy to inflate. Thus, low airway pressure increments yield high volume changes. PEEP titrated to this part of the curve is optimal PEEP. The upper inflection point defines the point of (near) maximal lung volume. The absolute volume is high in healthy subjects but low in e.g. ARDS patients (‘baby lung’ concept) [12]. In this overdistension zone, high pressures will be needed to inflate the already overinflated lungs and volutrauma will ensue.
The alveolar opening pressure is not the same for all alveoli [29, 30]. Some are easily opened at low pressures, others need high pressures, and others still will not open despite recruitment manoeuvres. The S-shape is most consistently observed in patients with ARDS but it is not prominent in healthy subjects, who are less prone to atelectasis and in whom overdistension is observed only with very high VT. But even though the typical S-shape of the curve is not always present, the concepts of recruitment, safe and overdistension zones apply to all patients.
Optimal PEEP is the level of PEEP that positions the lung above the lower inflection point. Fig. 19.3a shows a theoretical model where the P–V curve would be straight. During pressure controlled ventilation (PCV), 20 cm H2O Paw + 10 cm H2O PEEP would generate the same VT as 20 cm H2O Paw + 0 cm H2O PEEP (ZEEP) because these changes occur along a straight line. With an S-shaped P–V curve (Fig. 19.3B), the same pressure difference (i.e. 20 cm H2O ) will generate a different VT with different PEEP levels. Ventilation with PEEP above the lower inflection point would position the lung on the straight ‘safe’ line. Ventilation without any PEEP (ZEEP) and thus below the lower inflection point would position the lung on the more horizontal part where compliance is worst, and as a consequence a lower VT for the same pressure difference will be generated when PEEP is reduced. Thus, the lower inflection point and optimal PEEP can be identified by monitoring the expired VT while decreasing PEEP during PCV. This procedure is described in Table 19.3.
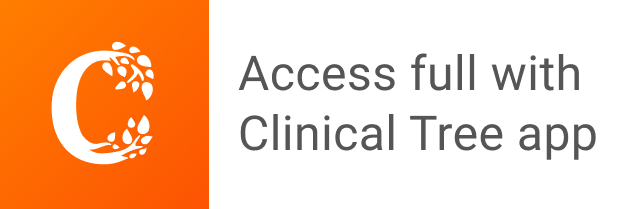