Laura A. Downey1 and David Faraoni2 1 Departments of Anesthesiology and Pediatrics, Emory University School of Medicine, and Children’s Healthcare of Atlanta, Atlanta, GA, USA 2 Department of Anesthesiology, Perioperative and Pain Medicine, Texas Children’s Hospital, and Baylor College of Medicine, Houston, TX, USA Pediatric cardiac surgery is associated with a substantial risk of bleeding, frequently requiring the administration of allogeneic blood products. The anemia and coagulopathy observed in neonates and children perioperatively are complex and multifactorial. Efforts to optimize preoperative hemoglobin, understand and correct coagulopathy, and incorporate blood conservation strategies to reduce bleeding are integral parts of pediatric cardiac anesthesiology and surgery. In this chapter, we will begin by reviewing the coagulation pathway, its maturation, and alterations associated with the pathophysiology of congenital heart defects (CHD). We will address preoperative optimization and blood conservation techniques, issues involved with the management of the coagulation system during and after cardiopulmonary bypass (CPB), and modalities for treating coagulopathy and bleeding in the perioperative period. Hemostasis is a complex physiologic process that regulates the formation and dissolution of a fibrin network through (i) platelet activation and aggregation, (ii) thrombin generation and fibrin clot formation, and (iii) fibrinolysis. The normal hemostatic mechanism that is triggered after vascular injury initiates a complex cascade of biochemical signaling and interactions between platelets, coagulation proteins, proteolytic enzymes, and fibrinolytic mechanisms. All of these actions are tightly regulated so that clotting occurs only at the site of vascular injury while maintaining blood flow in other parts of the circulation. The three phases of hemostasis are (i) primary hemostasis (formation of the platelet plug), (ii) secondary hemostasis (coagulation), (iii) tertiary hemostasis (fibrinolysis) (Figure 16.1). Figure 16.1 Simplified mechanisms of hemostasis including primary hemostasis and secondary hemostasis. Primary hemostasis reduces bleeding after vessel damage by inducing vasoconstriction at the site of injury and initiating platelet adhesion, activation, and aggregation to form a platelet plug. First, damage to the blood vessel walls exposes subendothelial collagen, which then binds circulating von Willebrand factor (vWF). Platelets adhere to the exposed vWF via glycoprotein 1b (GPIb) receptor on platelet surfaces. The binding of platelets to the subendothelial collagen‐vWF complex triggers platelet activation. Upon activation, platelets release thromboxane A2 (TXA2) and adenosine diphosphate (ADP) from their granules. These hemostatic mediators strengthen interactions between adherent platelets and promote the recruitment of additional circulating platelets to the growing platelet plug. Subsequently, negatively charged phospholipids are expressed on the surface membrane of activated platelets, which create an active surface on which coagulation proteins interact. Finally, the platelet glycoproteins GpIIb/IIa are converted to the active form and bind both fibrinogen and vWF to promote aggregation. The process of platelet aggregation is further modulated by the microenvironment. At low shear rates, platelet aggregation occurs predominately via (alpha)IIbß3 integrin and fibrinogen interactions, while at higher shear rates, platelets aggregate increasingly in a vWF‐dependent manner. Further aggregation and platelet plug development occur as surrounding platelets are activated by serotonin, TXA2, and ADP released by other platelets [1]. This local process is tightly controlled to prevent the propagation of the platelet plug beyond the site of injury by power platelet aggregation inhibitors and vasodilators produced by non‐injured endothelial cells. Secondary hemostasis leads to the formation of a fibrin clot by sequentially activating circulating coagulation factors to finally form insoluble fibrin. Our understanding of this “coagulation cascade” has evolved from the traditional description of independent “intrinsic” and “extrinsic” pathways being activated in plasma to a “cell‐based” concept of coagulation factors interacting on the surface of tissue factor (TF)‐bearing cells and activated platelets at the site of vascular injury [2]. The cell‐based model describes three overlapping phases of coagulation: initiation of coagulation factors on TF‐bearing cells, amplification of coagulation by thrombin generated on these TF‐bearing cells, and propagation of thrombin generation on platelet surfaces. TF, exposed in the vascular sub‐endothelium after injury, is the primary physiologic initiator of coagulation [2]. Initiation of coagulation occurs when plasma factor VII (FVII) binds tightly to TF and is rapidly activated to FVIIa. This FVIIa‐TF complex catalyzes the activation of coagulation factors, FX and FIX. The FXa on the surface of TF‐bearing cells binds with FVa to produce small amounts of thrombin (II). This initial amount of thrombin, though insufficient to initiate fibrin formation, plays an important role in subsequently activating platelets and FVIII during the amplification phase [2]. During the amplification phase, the small amount of thrombin generated on TF‐bearing cells amplifies the initial procoagulant signal by enhancing platelet adhesion, activating platelets, and activating factors V, VIII, and XI. Thus, thrombin acts on the platelet surface to “set the stage” for procoagulant complex assembly [2]. On the activated platelet surface, FIXa joins with FVIIIa to form the “tenase” complex (FVIIa/FIXa). The complex activates large amounts of FXa which joins with its cofactor, FVa, to form the “prothrombinase” complex (FXa/FVa). During the propagation phase, the “tenase” and “prothrombinase” complexes are assembled on the platelet surface to generate a large‐scale conversion of prothrombin into thrombin. Unlike the small amount of thrombin produced in the initiation phase, this quantity of thrombin is sufficient to cleave fibrinogen to fibrin strands that are necessary for clot formation [2]. The final phase of hemostasis, termed fibrinolysis, involves fibrin clot maturation and fibrinolysis. Activated factor XIII cross‐links the fibrin monomers and acts as “mortar” that cements the platelet plug to form a stable clot. After clotting has occurred and bleeding has stopped, activation of the fibrinolytic system breaks down the thrombus in order to re‐establish normal blood flow. Circulating plasminogen binds to fibrin within the clot. Tissue plasminogen activator (tPA) released from endothelial cells also complexes with fibrin and converts the bound plasminogen to its active form, plasmin, leading to the enzymatic dissolution of the thrombus. This stage is critical to maintaining and restoring the patency of the involved blood vessel so that blood flow can be restored, and wound healing can proceed. The hematological system constantly balances the ability to keep blood flowing through undamaged vessels with the ability to stop bleeding from damaged blood vessels. Under normal circumstances, blood vessels are non‐thrombogenic because of intact endothelial surfaces and circulating activated protein C, with its cofactor protein S inactivating any circulating FVa and FVIIIa to attenuate thrombin generation. Antithrombin (AT) neutralizes circulating FXIa, FXa, FXIa, and thrombin to prevent fibrin formation. Circulating nitric oxide and prostacyclin inhibit platelet activity and release tPA to promote fibrinolysis. Thus, clot formation is prevented in undamaged blood vessels while vascular damage disrupts these homeostatic mechanisms to create an environment conducive to clot formation. Furthermore, when vascular damage does occur, the coagulation process is restricted only to the injured vessels because the coagulation process‐inducing TF is expressed on damaged vessels and also because a host of circulating anticoagulants keeps the actions circulating activated coagulation factors in check. Under normal conditions, coagulation and fibrinolysis occur only where they are needed and are prevented from becoming pathological generalized problems by procoagulant and anticoagulant factors that delicately balance the system. Like most organ systems in the newborn, the hematologic system is not fully developed at birth but matures over the first year of life. In the late 1980s, Dr. Maureen Andrew termed the age‐related changes of the hemostatic system developmental hemostasis [3]. This parallel maturation of the coagulation and fibrinolytic systems maintains the delicate hemostatic balance between thrombosis and bleeding in healthy neonates and infants. An understanding of these changes in the coagulation system is critical for practitioners involved in caring for pediatric cardiac patients. Although maternal coagulation factors do not cross the placenta, the synthesis of coagulation proteins begins at approximately 11 weeks gestation. Levels of coagulation factors are dependent on the gestational age of the neonate, with major increases in both procoagulant and anti‐coagulant factors occurring between 30 and 40 weeks of gestation and reaching adult levels by 1 year of age [3–5]. In contrast, levels of fibrinogens, FV, VIII, FXIII, vWF, and platelet counts are comparable to adult values on the first day of life. In addition to these quantitative differences, recent studies have demonstrated qualitative differences in several hemostatic components, which manifest throughout the three stages of hemostasis (Table 16.1). Platelet counts in neonates are similar to those found in older children and adults, but neonatal platelets show a qualitative decrease in function for the first 2–4 weeks of life. However, the literature regarding neonatal platelet function is conflicting, with some studies demonstrating platelet hyporeactivity and others demonstrating accelerated coagulation [6–13]. The in vitro activation of neonatal platelets is decreased to a variety of standard agonists including epinephrine, ADP, collagen, and thrombin [10, 14]. This hyporeactivity manifests in several ways: (i) a decrease in platelet granule secretion; (ii) decreased expression of fibrinogen‐binding sites on platelet surfaces; (iii) deficiencies in neonatal phospholipid and calcium metabolism that impair platelet activation; and (iv) immature signal transduction pathways and reduced platelets surface receptors that impair platelet aggregation [6, 15]. As such, Caparros‐Peres and colleagues report reduced platelet adhesion and aggregation in a plasma‐free medium [11]. Despite the impaired function in in vitro, most in vivo assays of platelet function show adequate neonatal platelet function. For example, bleeding time (BT) and platelet closure time (PFA‐100) have been shown to be shorter than adults, suggesting increased adhesion and aggregation [12]. Similarly, viscoelastic testing often demonstrates normal or accelerated coagulation in this population [7]. Interestingly, when adult platelets were placed into neonatal blood, bleeding times were dramatically shorter when compared with adult platelets added to adult blood [13]. These in vivo assays may be explained by the prominent role that plasma proteins play in neonatal hemostasis. In fact, neonates have a higher concentration of plasma vWF and a greater percentage of the ultra‐large vWF necessary for improved platelet adhesion. Despite these conflicting studies, primary hemostasis appears to remain intact in healthy neonates and children. Table 16.1 Differences in coagulation components between neonates and adults Source: Adapted from Anderson et al. [3–5]. vWF = von Willebrand factor; F = factor; TFPI = Tissue factor pathway inhibitor; AT = antithrombin; PC = Protein C; PS = Protein S; alpha2M = alpha2‐macroglobulin; PAI = Plasminogen activator inhibitor. At birth, many of the coagulation factors involved in secondary hemostasis are quantitatively lower than adults. In newborns, mean levels of the vitamin K‐dependent procoagulant factors (II, VII, IX, X) and contact factors (XI, XII, PK, and HMWK) are about 70% of adult levels (Figure 16.2) [3]. This can be attributed to the immature fetal liver, which has reduced ability to provide the gamma‐carboxylation needed to produce vitamin K‐dependent factors [16]. This is partially counterbalanced by higher levels of vWF and ultra‐large vWF, which improve adherence between platelets and injured vessels [17] (Figure 16.3). Neonatal hemostasis is balanced by having concurrent lower levels of the anticoagulants antithrombin (AT), Protein C, and Protein S, and fibrinolytic factors (plasminogen and tPA) (Figure 16.4) [3–5]. The higher levels of thrombin inhibitor alpha2‐macroglobulin counteract lower levels of other anticoagulants. In the presence of these quantitative and qualitative factor deficiencies, neonates tend to generate significantly less thrombin than adults. In addition to lower levels of clotting factors, the existence of neonatal forms of clotting proteins has been described. Most notably, Brown and colleagues demonstrated an immature form of fibrinogen [18], which has differing phosphorus and sialic acid content than adult fibrinogen [19]. These differences lend unique properties to the differences in clot formation between neonatal and adult fibrinogen [18, 20]. Clots formed from adult fibrinogen display higher fiber density and significant three‐dimensional branching structure. In contrast, clots formed from neonatal fibrinogen display a more linear, two‐dimensional structure with increased porosity and less branching [18]. Clots formed from neonatal fibrinogen display a significantly faster degradation rate than their adult fibrinogen counterparts [18]. However, when adult and neonatal fibrinogen are mixed, simulating a transfusion event, neonatal and adult fibrinogen do not integrate completely during the process of fibrin formation (Figure 16.5). Even more concerning, these mixed clots have a slower degradation time than clots formed from neonatal fibrinogen alone. This data suggests neonatal clots may be more susceptible to fibrinolysis, but differences in the neonatal fibrinolytic proteins may help maintain the hemostatic balance. Tertiary hemostasis mainly involves the breakdown of the fibrin clot by plasmin. Neonatal plasminogen levels are about 25–50% of adult levels until about 6 months of age [21, 22]. Since plasminogen’s in vivo activator, tPA, is comparably low in neonates, the rate of conversion from plasminogen to plasmin is also decreased in neonates relative to adults. In contrast, the activity of plasminogen activator inhibitor type 1 (PAI‐1), the major inhibitor of plasminogen activation, is similar in neonates and adults [22]. Due to these imbalances and a qualitatively dysfunctional form of plasminogen, plasmin is generated at much slower rates in neonates [21]. Overall, the balance of factors favors a reduction in fibrinolytic activity. Figure 16.2 Vitamin K‐dependent coagulation factors and contact factors in full‐term infants through adulthood. F = factor. (Source: Adapted from Andrew et al. [5].) Figure 16.3 Coagulation factors in full‐term infants through adulthood. F = factor; vWF = von Willebrand Factor. (Source: Adapted from Andrew et al. [5].) Figure 16.4 NEW Vitamin K‐dependent coagulation factors and contact factors in full‐term infants through adulthood. AT‐III = Antithrombin III. (Source: Adapted from Andrew et al. [5].) In spite of these quantitative and qualitative differences in neonatal hemostasis, healthy neonates rarely suffer from spontaneous hemorrhage or thrombosis. This suggests that the maturation of procoagulants and anti‐coagulant factors maintain a relative balance in healthy neonates. However, thrombotic complications are more common in neonates, and thromboelastography (TEG) has shown that neonates and infants actually clot faster and have increased clot strength compared with adults [7]. For patients undergoing cardiac surgery, this balance can be easily disrupted and these patients can be placed at risk for both bleeding and thrombotic complications. In addition to the age‐related effects on coagulation factor levels and function, children with congenital heart disease (CHD) have complex alternations in their whole blood composition and coagulation profile, even more so in the presence of long‐standing hypoxemia. Since the current practice of pediatric cardiac surgery is to perform corrective procedures in infancy, most of the studies on the effects of long‐standing hypoxemia are several decades old. However, children with delayed surgical repair or single ventricle physiology (SVP) who live with chronic hypoxemia will develop cardiac pathophysiology‐induced coagulation abnormalities that affect the hematologic, endothelial, and hemostatic systems. These patients are at increased risk for perioperative bleeding and clotting due to the complex interplay of coagulation abnormalities including secondary erythrocytosis, thrombocytopenia, impaired platelet function, deficiency of vWF multimers, and a range of clotting deficiencies. Figure 16.5 Confocal microscopy analysis of clots constructed from both purified neonatal and adult fibrinogen. Clots were formed from purified adult (A, B) or neonatal (C, D) fibrinogen at a concentration of 2.5 (A, C) or 3.5 mg/mL (B, D) fibrinogen in the presence of 10 μg/mL thrombin. Clots were also formed by combining neonatal and adult fibrinogen in a 2.5 : 1 (E, G, H) or 1 : 2.5 (F, I, J) ratio to create clots simulating conditions observed after transfusion with a total fibrinogen concentration of 3.5 mg/mL in the presence of 10 μg/mL thrombin. Neonatal fibrinogen was labeled with Alexa‐Flour 488 (green) to allow for separate visualization of adult and neonatal fibrinogen labeled with Alexa‐Flour 555 (red). Representative images are presented. For combined fibrinogen samples, overlaid neonatal and adult fibrinogen channels (E, F) and corresponding individual neonatal (G, I) and adult fibrinogen (H, J) channels are presented. Scale bar = 20 μm. (Source: Brown et al. [18]. Reproduced with permission of Wolters Kluwer Health, Inc.) Irrespective of the primary cardiac lesions, patients with chronic hypoxemia often develop secondary erythrocytosis, which refers to an isolated increase in red blood cells (RBCs). This is in contrast to polycythemias that result in a marked increase in all three blood cell lines (RBCs, white blood cells, and platelets). In cyanotic heart disease, long‐standing hypoxemia and reduced tissue oxygenation stimulate the kidneys to produce erythropoietin (EPO). This secondary erythrocytosis is an adaptive physiologic mechanism designed to compensate for inadequate oxygenation. However, in the presence of a persistent right‐to‐left shunt causing chronic hypoxemia, tissue oxygenation cannot improve beyond a certain point. Nevertheless, the kidneys continue to secrete EPO, leading to a further increase in red cell mass and blood viscosity. As blood viscosity increases with the higher number of hematocrits, blood flow may become sluggish and increase the risk of thrombosis and infarction. In fact, the increased mass has been linked with an increased risk of cerebrovascular events in neonates and infants with cyanotic heart disease [23]. In addition to secondary erythrocytosis, many patients with CHD demonstrate abnormal hemostatic profiles. While this is multi‐factorial, qualitative and quantitative deficiencies in both platelets and fibrinogen are implicated. Patients with hematocrits above 60–65% often have decreased platelet counts. The thrombocytopenia seen in CHD patients is due to both decreased platelet production and increased peripheral destruction due to platelet activation from the high shear stress generated by increased blood viscosity [24]. Recent studies using TEG suggest that despite normal to elevated fibrinogen concentration, the fibrinogen function may be impaired. Cui and colleagues found that baseline tracings in patients with preoperative hematocrits >54% have decreased peak amplitude on TEG and TEG‐functional fibrinogen when compared to normal patients [25]. Similarly, Jensen and colleagues demonstrated that patients with a preoperative hematocrit >57% have a smaller angle (alpha), primarily representative of the fibrinogen contribution to clot formation [26]. In addition, many patients with CHD have reduced levels of vitamin K‐dependent factors (II, V, VII, X) likely due to chronic hypoxia and chronic passive congestion of the liver [27]. Finally, these patients appear to have accelerated fibrinolysis based on increased levels of D‐dimers and elevated plasminogen activator inhibitor type 1 (PAI‐1). However, it is unclear if the accelerated fibrinolysis is due to low‐grade intravascular thrombosis, increased fibrinolysis, or increased turnover of fibrinogen [28]. Table 16.2 Coagulation factor levels (mean ± SD) over time for the subgroup of 17 patients who had complete samples drawn at each stage Adapted from Odegard and colleagues [29] Data are mean ± SD. BDG, Bidirectional Glenn. The following symbols denote significant changes (P < 0.005) between selected stages: a Stage I to pre‐BDG; b Pre‐BDG to pre‐Fontan; c Pre‐Fontan to post‐Fontan. While chronic hypoxemia affects multiple aspects of the coagulation system, a prospective longitudinal study of coagulation factors in 20 neonates with hypoplastic left heart syndrome (HLHS) at each stage of their palliation highlighted the complexity of hemostatic changes in congenital cardiac patients. In these children, significantly decreased levels of fibrinogen, vitamin K‐dependent factors (II, VII, XI, and X), factors V and VII, as well as anti‐coagulation factors (anti‐thrombin, Protein C, Protein S, and plasminogen) were noted after initial palliation. These abnormalities persist through the bidirectional cavopulmonary anastomosis and just prior to the Fontan completion. Even after Fontan completion, many of these abnormalities persist, with the exception of an increase in FVIII levels and activity (Table 16.2). It is likely that the imperfect balance of procoagulant and anti‐coagulant factors contributes to the significant incidence of thrombotic events that occur in these patients [29]. In summary, the hemostatic abnormalities in patients with CHD are complex and include secondary erythrocytosis, thrombocytopenia, impaired fibrinogen function, and multiple coagulation factor deficiencies. Despite our best efforts at minimizing the effects of cardiac surgery on hemostasis, the underlying qualitative and quantitative hemostatic changes related to CHD itself predispose these patients to both bleeding and thrombosis. Bleeding in the perioperative period of cardiac surgery is associated with increased incidence of postoperative complications and mortality, both from the direct effects of bleeding and from the therapies deployed to restore hemostasis. As an example, blood transfusion has been shown to be associated with a dose‐dependent increased risk of postoperative infection and mortality in pediatric surgical patients [30]. Several studies have suggested that the increased bleeding risk observed in patients with CHD may be attributable to multiple contributing factors including both quantitative and qualitative differences in coagulation factors, relative immaturity of the coagulation system, endothelial dysfunction, intrinsic risk of surgical procedures, associated comorbidities (e.g., liver dysfunction), and iatrogenic factors (e.g., anticoagulation therapies, central venous access) [26, 31, 32]. Among children with CHD undergoing surgery, those with cyanotic heart disease (e.g., single ventricle physiology) are considered at higher risk for both intraoperative bleeding and postoperative thrombotic complications [29, 33]. Although the exact mechanisms for bleeding and thrombotic risks in children with CHD remain poorly understood, a complex multifactorial etiology including modifiable and non‐modifiable factors is hypothesized. Figure 16.6 summarizes the risk factors reported in the literature and that are discussed throughout this chapter. Patient‐risk factors are mainly related to the age of the patient at the time of surgery. Several authors have reported a strong association between age and the incidence of bleeding, with the highest risk of hemorrhage being reported in neonates [35, 36]. Age at the time of surgery is also associated with surgery‐specific risk factors as neonates often require complex surgical procedures involving prolonged CPB duration sometimes associated with deep hypothermic cardiac arrest (DHCA) [37]. Another surgery‐related risk factor is the timing for surgery (i.e., need for urgent or emergent surgery) which often mean hemodynamic instability (i.e., heart failure sometimes requiring extracorporeal membrane oxygenation [ECMO]), high requirement of ventilatory or inotropic support, and presence of multiple organ failure (i.e., acute kidney injury, liver failure, pulmonary edema). As discussed above, different bleeding risks have been reported according to the type of CHD. The presence of cyanosis will have an impact on coagulopathy, fibrinogen polymerization, and platelet function [26, 38]. Patients with cyanotic heart disease often require multiple surgical procedures, also increasing the bleeding risk. Although several authors have studied the predictive values of preoperative coagulation testing (i.e., standard laboratory tests, viscoelastic tests, thrombin generation, or platelet function), none of these tests have been shown to accurately predict perioperative bleeding, a testimony to the complexity and the multifactorial nature of perioperative bleeding [39, 40]. Although most risk factors are non‐modifiable, it is important to estimate the risk before surgery in order to choose the appropriate tools to monitor and treat coagulopathy using a standardized and targeted transfusion algorithm that we will discuss later in this chapter. Figure 16.6 Factors affecting perioperative hemostasis in pediatric cardiac surgical patients. (Source: Cohen et al. [34]. Reproduced with permission of Cambridge University Press.) During the preoperative evaluation of patients coming for cardiac surgery, practitioners should perform a complete history and physical examination to determine what laboratory testing is necessary. Patients with cyanotic heart disease may have secondary erythrocytosis, thrombocytopenia, abnormal levels of fibrinogen, and other coagulation factors. In addition, these children often have abnormal liver function tests secondary to systemic hypoperfusion, heart failure, hypoxemia, and hyperviscosity. Because the detection of these abnormalities and correction may help minimize the risk of bleeding during the intraoperative period, it is recommended that a basic electrolyte panel, complete blood cell count with differential, coagulation profile, a type and crossmatch, and liver function tests be obtained [41]. Practitioners should also question the patient and family members about bleeding or thrombotic events to determine if further hematology work up is necessary. For outpatients with acyanotic heart disease, several institutions have adopted strategies aimed at minimizing preoperative laboratory testing to reduce patient stress related to blood draws, minimize the risk of iatrogenic anemia, and reduce additional costs. In a survey of 12 congenital cardiac surgical programs, 11 institutions still send most baseline laboratory testing. However, many practitioners reported that in the year following the original survey, no cases were canceled or their management changed because of these laboratory results. While most practitioners agreed that a type and screen and complete blood count would be sufficient to manage outpatients, only one institution has implemented these changes thus far [42]. One of the cornerstones of modern Patient Blood Management protocols is the identification and the treatment of preoperative iron deficiency with or without anemia. Pediatric cardiac surgical patients can range from newborns to adolescents, suffering from cyanotic or non‐cyanotic cardiac defects, which result in varying baseline hemoglobin (Hb) concentrations and ferritin levels. The optimal preoperative Hb values for pediatric cardiac surgery remain to be determined as the World Health Organization (WHO) definition of anemia for pediatric patients does not apply to neonates and children with variable degrees of cyanosis and secondary erythrocytosis. However, one could argue that any pediatric cardiac surgical patient with a preoperative Hb below 11 g/dL should be considered anemic and might be suitable for preoperative anemia study and therapy. Higher thresholds might be worth consideration, especially in children with cyanotic heart defects. If a Hb of 11 g/dL is chosen as a threshold for intervention, up to 50% of patients with CHD would be diagnosed with preoperative anemia and should therefore be started on iron therapy before their procedure. In neonates and children undergoing noncardiac surgery, preoperative anemia was associated with an increased risk of mortality and postoperative complications [43, 44]. In cardiac patients, preoperative anemia was associated with an increased risk of perioperative acute kidney injury (AKI) in a retrospective cohort study of 220 patients undergoing cardiac surgery [45]. In addition, preoperative anemia has been shown to increase the risk of allogeneic transfusion in children undergoing noncomplex cardiac surgery (i.e., repair of ventricular or atrioventricular septal defects) [46]. Therefore, similar to adult patients, assessment of preoperative anemia and appropriate treatment should be recommended to avoid complications associated with perioperative and reduce the need for avoidable allogeneic blood transfusions. Iron deficiency is present in an estimated 70–80% of pediatric patients with inflammatory bowel disease and has been shown to impair health‐related quality of life [47]. Iron deficiency is also common in patients with chronic kidney disease and renal failure and is associated with increased all‐cause and cardiovascular mortality [48]. Iron deficiency was recently studied in children with heart failure [49]. In this cohort, the authors found that 56% of children with heart failure presented with iron deficiency. They also found that iron deficiency was associated with greater risks of ventricular assist device implantation, heart transplantation, or death. In adults, iron deficiency has been repeatedly shown to be associated with greater cardiovascular and all‐cause mortality, poorer functional capacity, lower health‐related quality of life, and greater readmission rates in patients with chronic heart failure. Multiple randomized controlled trials have shown that treating iron deficiency with intravenous iron improves each of these outcome measures [50, 51]. Keeping that in mind, preoperative treatment of iron deficiency anemia is recommended in some adult and pediatric guidelines for the management of heart failure [52] or blood conservation in adults and pediatric cardiac surgical populations [53, 54]. In children undergoing cardiac surgery, the optimal approach for preoperative treatment of iron deficiency anemia has never been appropriately studied. For the preoperative administration of iron, only a very limited number of studies exist, most of them looking at oral iron supplementation [55]. The largest study using intravenous iron has been published by Hassan and colleagues in 2017 in which the authors demonstrated that Ferumoxytol was effective in treating iron‐deficiency anemia in a small retrospective cohort of 54 patients [56]. The members of this group pointed out that a slow infusion rate and close monitoring allowed early detection of the infrequent adverse drug reactions. Although the concomitant administration of EPO has sometimes been suggested, data supporting the efficacy and safety of EPO in pediatric cardiac patients is sparse. In a small retrospective study, Shimpo and colleagues demonstrated that the administration of up to 300 IU/kg EPO was effective as an adjuvant therapy for open‐heart surgery [57]. However, the appropriate dosing regime has not been determined thoroughly. Appropriately designed studies are urgently needed to define the optimal treatment strategy (oral vs. intravenous iron), the dose, the duration, and to understand whether the addition of EPO would sometimes be beneficial. Figure 16.7 Integration of iron deficiency and anemia management into guidelines for enhanced recovery following pediatric cardiac surgery. (Source: made in ©BioRender ‐ biorender.com.) Although one of the challenges often reported for preoperative assessment and treatment of anemia in adult cardiac surgical patients is the short window between the initial patient assessment and the time of surgery, this is often not an issue in infants and children who usually have to wait weeks, sometimes months, before their surgical procedure. We should therefore take advantage of the wait time to correct iron deficiency and anemia. In addition, postoperative iron deficiency and hospital‐acquired anemia are also important issues that require our attention, and efforts should be undertaken to reduce unnecessary blood sampling and treatment of iron deficiency anemia should be initiated or resumed in the postoperative period before hospital discharge. The development of enhanced recovery after surgery (ERAS) protocols, as recently emphasized by the members of the American Association of Thoracic Surgeons’ Cardiac Clinical Practice Standards Committee [58], is an excellent opportunity to integrate perioperative management of iron deficiency and anemia integrated into the multimodal protocol to optimize enhanced recovery following pediatric cardiac surgery (Figure 16.7). Preoperative autologous donation (PAD) refers to the collection and anticoagulation of whole blood from a patient for anticipated perioperative transfusion. The amount of blood collected from a single donation is limited to about 10% of the patient’s total blood volume. While it may eliminate the risk of blood‐borne infections and may diminish immune modulation, PAD remains controversial due to issues with safety, cost, logistics around surgical scheduling, and the potential pathophysiologic effects on a child with complex CHD. Therefore, this practice is generally reserved for relatively healthy adolescent patients with simple cardiac anomalies. In contrast, autologous intraoperative blood collection and retransfusion have become more popular in order to minimize the risks associated with PAD. Acute normovolemic hemodilution (ANH) is the removal of whole blood from the patient before CPB while maintaining normovolemia through the infusion of crystalloids or colloids and then transfusing the blood after CPB. Patient selection depends on baseline hematocrit and hemodynamic stability, the composition of the CPB prime, and the targeted hematocrit on CPB. A retrospective study of 209 pediatric patients undergoing cardiac surgery (with ANH when appropriate), showed that 59.6% of patients >6 kg did not receive any blood transfusions, while all patients under 6 kg received a blood transfusion. Another study of 36 patients <10 kg matched 1:1 for intraoperative autologous blood collection and retransfusion vs. standard practice, demonstrated that patients in the study group received fewer blood products overall (47 mL/kg vs. 68 mL/kg) without increased 24 hours chest tube output, mechanical ventilation, or intensive care units (ICU) length of stay. Of note, platelet count and fibrinogen levels were significantly lower in the study group compared to the control group. While these studies show that the practice is relatively safe, this practice does not necessarily reduce overall blood transfusions in patients under 18 kg [59–61]. Figure 16.8 Summary of hemostatic activation mechanisms on cardiopulmonary bypass. BK = bradykinin; FXIIa = activated factor XII; TF = tissue factor; TPA = tissue plasminogen activator; Plt = platelets; Fib = fibrin degradation products; Endo = endothelium. (Source: Sniecinski et al. [62]. Reproduced with permission of Wolters Kluwer Health, Inc.) Cardiopulmonary bypass (CPB) is a unique scenario when the patient’s blood is exposed to non‐endothelial/non‐physiologic surfaces leading to a widespread activation of the coagulation, fibrinolytic, and inflammatory systems (Figure 16.8). Several mechanisms are involved in the hemostatic activation induced by CPB [62, 63]. The contact system (bradykinin system) is activated immediately when blood is in contact with the CPB artificial surfaces. In addition, factor XII auto‐cleaves itself upon contact with a variety of anionic surfaces leading to activated FXII, which converts prekallikrein into active kallikrein. Plasma kallikrein creates a positive feedback loop by producing more activated FXII, as well as producing bradykinin from high‐molecular‐weight kininogen (HMWK) [64]. Bradykinin levels increase 10‐fold due to increased HMWK cleavage as well as reduced lung clearance secondary to minimal pulmonary blood flow [65]. This has important implications for fibrinolysis because elevated bradykinin levels induce secretion of tPA. The role of thrombin generation during CPB is well‐documented, even in the presence of adequate anticoagulation [66]. The activation of the contact system, as well as the exposure of TF on the membrane of traumatized cells by aspiration of the surgical field and CPB, expression of TF due to inflammation and due to the generated thrombin will promote a vicious circle of activation of the hemostatic and fibrinolytic system. Endothelial cells release tissue‐type plasminogen activator (tPA), a major activator of plasminogen leading into fibrin‐degrading plasmin. In the setting of CPB, soluble and circuit‐bound fibrin provides a huge surface for plasminogen activation to occur. There is a 10‐ to 100‐fold increase in plasmin generation shortly after the commencement of CPB, and plasmin generation, along with fibrin degradation, remains increased 10‐ to 20‐fold throughout the duration of CPB [67]. During CPB, fibrin formation and degradation rates are nearly equal, indicating that much of the fibrin degradation is not at the sites of vascular injury. This hyperfibrinolytic state consumes fibrinogen, leaving less available for coagulation postoperatively. After CPB, platelet adhesion is decreased to the destruction of GPIb receptors by plasmin and by the shear stress. In addition, platelet aggregation is also decreased by thrombin, plasmin, and fibrin degradation products. Altogether, these processes lead to a quantitative and qualitative consumption of coagulation factors and platelets during CPB. Because coagulation and immune systems are associated, inflammation has multiple mediators that can create profound amplification in coagulopathy. A sepsis‐like clinical picture, named systemic inflammatory response syndrome (SIRS), often results from CPB and can be linked to the close relationship between inflammation and coagulation. Leukocytes, including neutrophils and monocytes, bind to and are activated by the surface of the CPB, which leads to an increase in tissue expression, procoagulant activation, and thrombin generation. The generation of inflammatory cytokines leads to increased vascular permeability, neutrophil infiltration into the tissue, and production of acute‐phase proteins. In addition, CPB needs to be primed with blood, fluid, or a combination leading to a variable degree of hemodilution promoting even more than coagulopathy. Postoperative hemostasis is greatly influenced by the degree of hemodilution that occurs as a result of CPB. The effect of dilution depends on the patient’s blood volume, the baseline blood concentrations (hematocrit, coagulation factors, platelets), and the volume and components of the CPB circuit prime. In children, hemodilution is an independent predictor of increased transfusions. As a result, smaller circuits have become more popular in order to minimize hemodilution, reduce the inflammatory response induced by CPB components, and decrease overall blood transfusions. Miniaturizing the CPB circuit is achieved by the elimination of non‐essential components, using small‐volume circuit components (oxygenator, filters, cannulae), decreasing the length and diameter of circuit tubing, using a remote pump head, assisted venous drainage, retrograde autologous circuit priming, and microplegia techniques [68]. As a result, some institutions report prime volumes of 95–110 mL for infants <5 kg. However, efforts to reduce the prime volumes require careful planning and meticulous management of bypass to mitigate risks. Originally, hemodilution during hypothermic CPB was used to reduce the use of blood products, promote microcirculatory flow and improve tissue perfusion. However, concerns emerged about postoperative cognitive impairment from reduced perfusion pressure, decreased oxygen‐carrying capacity, and increased cerebral blood flow causing an increased risk for cerebral microemboli. Subsequent studies have demonstrated that for infants undergoing biventricular repair, a hematocrit ~ 24% or higher at the onset of low‐flow CPB is associated with higher Psychomotor Development index scores at 1 year, reduced lactate levels, and improved fluid balance [69]. Some studies suggest that lower hematocrit levels are safe if mixed venous oxygen saturation, near‐infrared spectroscopy cerebral oxygen saturation, and lactate levels are maintained within the normal range [70]. Unfortunately, most of the studies evaluating lower hematocrits on CPB contain a small heterogeneous population and the nadir hematocrit was ~22%. In summary, due to differences in diagnosis, age at operation, and CPB management, it is unlikely that hematocrit alone can indicate when the benefits of RBC transfusion outweigh the risks of hemodilution. In addition to dilutional anemia, many coagulation factors have been noted to decrease at the initiation of CPB. Brown and colleagues demonstrated a decrease in both fibrinogen and FXIII due to hemodilution, which were restored to baseline levels after the transfusion of cryoprecipitate [18]. Of particular importance, the hemodilutional effects of CPB in neonates and infants often result in low fibrinogen levels that lead to impaired fibrin formation, inadequate clot formation, and increased risk of post‐CPB bleeding [18, 71–73] (Figure 16.9). Figure 16.9 Effects of hemodilution on coagulation factors in neonates undergoing cardiopulmonary bypass. Post‐CPB = cardiopulmonary bypass, F = Factor; PTF = Post Transfusion. (Source: Brown et al. [18]. Reproduced with permission of Wolters Kluwer Health, Inc.) In addition to miniaturizing the circuit volumes for pediatric patients, the prime composition is often adapted to the particular requirements of the patient and the operation. That being said, the majority of institutions still use a blood prime for infants and neonates. The lower the blood volume and hematocrit of the patient, the more banked blood needs to be added into the prime. While several institutions have access to fresh whole blood (<48 hours after donation), the majority of institutions use reconstituted whole blood (fresh frozen plasma (FFP) plus RBCs). Advocates for fresh whole blood prime believe that it reduces coagulopathy and also CPB‐mediated inflammation. However, Mou and colleagues performed a randomized trial in children <1 year of age comparing fresh whole blood with reconstituted blood. The authors found that patients who received fresh whole blood had increased ICU length of stay and more fluid overload [74]. In contrast, several other studies have demonstrated that patients receiving whole blood received fewer transfusions, significantly less chest tube output, and shorter length of mechanical ventilation and ICU stays [75–77]. Furthermore, adequately powered studies performed in the context of the current multimodal strategy to manage coagulopathy are required before the safety and efficacy of fresh whole blood can be determined. While most institutions still use RBCs and FFP in the CPB prime for neonates, there is little high‐grade evidence to support its use in older patient populations. Some institutions use albumin to maintain oncotic pressure and reduce fluid accumulation, while proponents of FFP argue that in addition to maintaining oncotic pressure, FFP increases the level of coagulation factors and reduces intraoperative transfusion requirements in complex neonatal and cyanotic patients. In a study of 60 patients weighing 7–15 kg randomized to FFP or crystalloid prime, Dieu and colleagues did not report any significant differences in median postoperative blood loss or transfusion requirements [78]. Other studies have demonstrated that patients with FFP prime have higher fibrinogen concentrations and improved TEG values at the end of CPB, but this was not associated with a decrease in postoperative transfusions. Unfortunately, the composition of the prime is often a matter of opinion and institutional practice and differs widely across institutions. The only consensus regarding CPB prime is minimizing transfusion requirements and hemodilution. Additional pediatric‐specific trials are needed to determine the optimal CPB prime composition.
CHAPTER 16
Coagulation, Cardiopulmonary Bypass, and Bleeding
Introduction
Coagulation
Mechanisms of hemostasis
Primary hemostasis
Secondary hemostasis
Tertiary hemostasis
Hemostatic homeostasis
Developmental hemostasis
Component
Neonatal versus adult levels
Primary hemostasis
←→ Platelet Count
↑ vWF
Coagulation factors
↓ FII, FVII, FIX, FX
↓ FXI, FXII
↓ to ←→ FVI, FXIII
←→ Fibrinogen
↑ FVIII, vWF
Anticoagulation factors
↓ PC, PS, AT, TFPI
↑ alpha2M
Fibrinolysis
↓ Plasminogen
↓ to ←→ PAI
Influence of congenital heart disease on coagulation
Erythrocytosis and hemostasis
Variable
Stage I
Pre‐BDG
Pre‐Fontan
Post‐Fontan
Fibrinogen (mg/L)
200.5 ± 70
203.5 ± 86
292.8 ± 76
350.5 ± 93
Antithrombin (%)
36.8 ± 12
75.4 ± 18a
93.1 ± 16b
56.9 ± 15
Protein C (%)
17.7 ± 9
43.2 ± 12a
60.2 ± 16b
56.9 ± 15
Protein S (%)
34.1 ± 17
81.3 ± 16a
71.1 ± 18
76.3 ± 19
Factor II (%)
37.5 ± 14
64.7 ± 15a
77.8 ± 13
81.9 ± 11
Factor V (%)
67.9 ± 19
79.1 ± 15
86.9 ± 21
71.8 ± 19
Factor VII (%)
32.7 ± 14
49.3 ± 13a
57.9 ± 15
49.2 ± 14
Factor VIII (%)
50.3 ± 21
63.5 ± 26
90.9 ± 36
175.5 ± 89c
Factor IX (%)
32.5 ± 14
47.2 ± 17
62.9 ± 13
72.5 ± 18
Factor X (%)
33.2 ± 9
63.6 ± 15a
71.5 ± 13
72.4 ± 16
Preoperative considerations
Predictors of bleeding post‐CPB
Preoperative laboratory testing
Preoperative anemia
Autologous donation
CPB‐associated coagulation changes
Exposure to CPB circuit
Hemodilution and CPB prime
Dilutional anemia
Dilution of coagulation factors
Composition of CPB prime
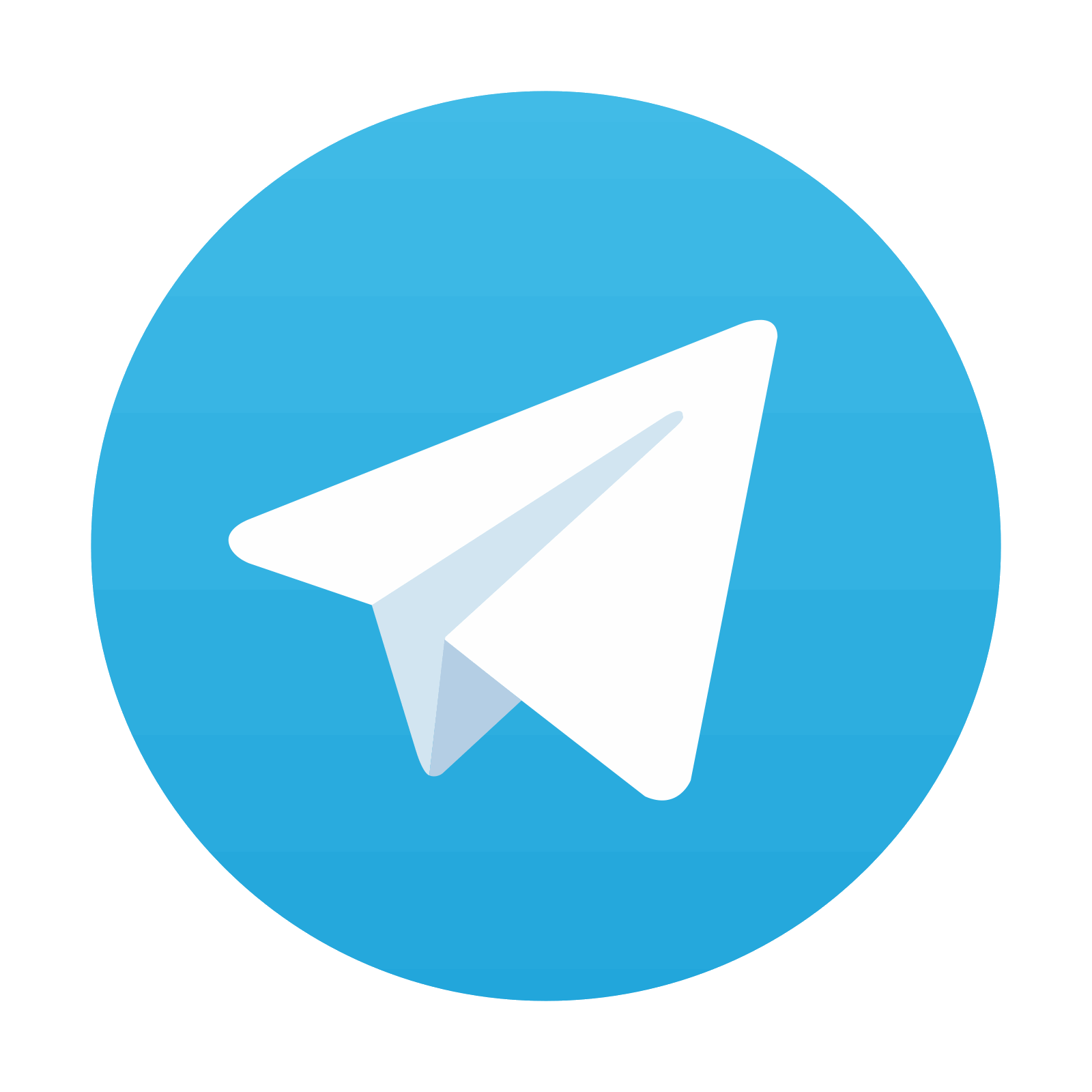
Stay updated, free articles. Join our Telegram channel
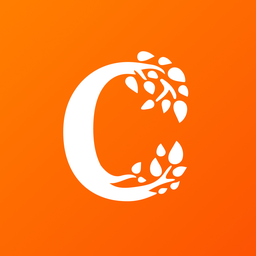
Full access? Get Clinical Tree
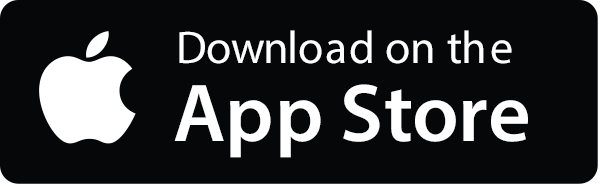
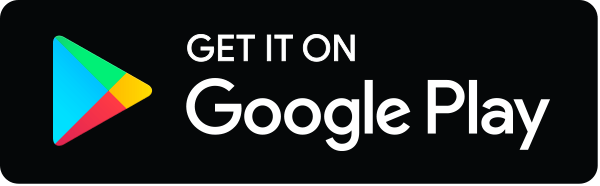