Abstract
In Europe, approximately 30% of surgeries are performed in patients with cardiovascular comorbidities and up to 42% of intraoperative complications are cardiac related [4]. Often, the anaesthesiologist needs to intervene to maintain haemodynamic function and prevent perioperative complications.
Introduction
In Europe, approximately 30% of surgeries are performed in patients with cardiovascular comorbidities and up to 42% of intraoperative complications are cardiac related [4]. Often, the anaesthesiologist needs to intervene to maintain haemodynamic function and prevent perioperative complications.
Cardiac and Haemodynamic Function
The haemodynamic system has a large reserve capacity, redundancy, closed-control loops and compensatory mechanisms. Critical failures that overwhelm these mechanisms lead to catastrophic physiological sequelae very quickly. Part of the quantitative assessment of the cardiac and haemodynamic system is to ascertain the degree to which these compensatory mechanisms are active, and how much reserve capacity the system still has available. An important end-point of cardiac and haemodynamic performance is the maintenance of adequate tissue perfusion and tissue oxygen delivery. Figure 14.1 illustrates the physiological mechanisms and relationships affecting tissue oxygen delivery.
Anaesthetic Medications
Volatile anaesthetic agents depress myocardial contractility and reduce SVR leading to a decrease in MAP. Nitrous oxide alone, for example, in sedative concentrations, has only slight systemic haemodynamic effects [5]. However, when combined with volatile anaesthetic agents, vascular resistance and blood pressure are increased, when compared to using the volatile anaesthetic without nitrous oxide but adjusted to achieve the same level of anaesthesia [6, 7]. Many anaesthetic agents also alter autonomic reflexes, e.g. avoiding or counteracting the increases in HR and blood pressure from surgical stimulation or from the irritating airway effects of some volatile anaesthetics. Among the intravenous anaesthetic agents, propofol has the most profound effects on the cardiovascular system (Table 14.1), including negative inotropy and vasodilation, resulting in marked hypotension. Ketamine, etomidate and opioids have considerably less impact (Table 14.1).
Table 14.1. Cardiovascular effects of intravenous anaesthetic drugs, including propofol [95], ketamine [95, 96], etomidate [95], and opioids [97].
BP | CO | SVR | HR | ||
---|---|---|---|---|---|
Propofol bolus induction | 25–40% decrease | 15% decrease | 15–25% decrease | unchanged | |
Propofol infusion maintenance | 20–30% decrease | unaltered | 30% decrease | variable | |
Ketamine | increase | increase | increase | ||
Etomidate | almost no effect on cardiovascular system | ||||
Opioids | (decrease) | (decrease) | (decrease, especially fentanyl) | milder than other IV anaesthetics |
Therapeutic Intervention Options for Haemodynamic Management
A recent survey revealed that only 5.4% of North American anaesthesiologists and 30.4% of European anaesthesiologists indicate that their institution or group have a written haemodynamic management protocol [3].
Table 14.2 lists pharmaceutical agents used in intraoperative haemodynamic management, their pharmacokinetic characteristics and their haemodynamic effect.
Drug | Onset of action | Duration of action | Receptor | Haemodynamic effect | ||||||
---|---|---|---|---|---|---|---|---|---|---|
(min) | (min) | α | β | CO | HR | SVR | MAP | PuVR | PerVR | |
Adrenaline | Immediate | 5–15 | + | + | ↑ | ↑ | ↑ | ↑ | 0 | |
Isoproterenol | 1–5 | 15–30 | 0 | + | ↑ | ↑ | ↓ | ↓ | 0 | |
Noradrenaline | Immediate | 5–15 | + | + | 0 | 0 | ↑ | ↑ | ↑ | |
Dopamine | 2–4 | 10–15 | + | + | ↑ | ↑ | ↑ | ↑ | 0 | |
Dobutamine | 1–2 (peak at 10) | 10–15 | 0 | + | ↑ | ↑ | ↓ | ↓ | ↓ | |
Milrinone | 1 | 45–60 | 0 | 0 | ↑ | 0 | ↓ | ↓ | ↓ | |
Phenylephrine | 1–3 | 10–20 | + | 0 | 0 | ↓ | ↑ | ↑ | ↑ | |
Vasopressin | 1–3 | 5–10 | 0 | 0 | 0 | 0 | ↑ | ↑ | 0 | |
Ephedrine | 1–3 | 15–20 | + | + | ↑ | ↑ | ↑ | ↑ | 0 | |
Levosimendan | 5 | 1–2 h | 0 | 0 | ↑ | 0 | ↓ | ↓ | ↓ | |
Esmolol | 1 | 10–20 | 0 | – | ↓ | ↓ | 0 | ↓ | 0 | |
Labetalol | 2–5 | 6h | – | – | 0 | (↓) | ↓ | ↓ | ||
Clevidipine | 2–4 | 5–15 | 0 | 0 | ↑ | 0 | ↓ | ↓ | ||
Nicardipine | 2 (5–15 for infusion) | 2–4 h (4–6 h for infusion) | 0 | 0 | ↑ | ↓* | ↓ | |||
Nitroprusside | Immediate | 1–2 | 0 | 0 | 0 | ↓ | ↓ | ↓ | ||
Nitroglycerin | 2–5 | 3–5 | 0 | 0 | ↓ | ↑ | ↓ | ↓ | ↓ | |
Clonidine | 30 | 4-6 h | +** | (↓) | ↓ | ↓ | ↓ | ↑ | ||
Urapidil | 2 | 4-5 h | – | 0 | 0 | ↓ | ↓ | ↓ | ||
Enalaprilat | 15 | 6h | 0 | 0 | (↑) | 0 | ↓ | ↓ | ↓ | |
Fenoldopam | 5 | 30–60 | 0 | 0 | ↑ | ↑ | ↓ | ↓ | ↓ | |
Hydralazine | 5–15 | 6–12 h | ↑ | ↑ | ↓ | ↓ | ↓ |
PuVR … Pulmonary Vascular Resistance, PerVR … Peripheral Vascular Resistance
* coronary, ** cerebral
Fluid shifts, haemorrhage, evaporation and changes in vascular capacity necessitate the administration of fluids. Outcome is affected by the volume, the type of fluid and timing of administration. Goal-directed therapy (GDT) aims to provide protocol-based guidance about fluid administration but there is no agreement on which if any of the published GDT approaches is best. Some have suggested that the variability in the preoperative and intraoperative conditions, patient comorbidities and anaesthetic management require marked individualizations of fluid management [8]. Moreover, determining the adequacy of fluid therapy is very difficult and frequently unreliable. Cannesson and Gan summarize the philosophy of GDT as providing fluids only, if the patient responds to the fluid [9]. Myburgh et al provide a thorough review of the different types of resuscitation fluids, but conclude that there is currently no ideal resuscitation fluid [10].
Haemodynamic Variables and Their Target Ranges
In a meta-analysis, a proactive haemodynamic monitoring strategy combined with targeted haemodynamic interventions has been shown to reduce surgical mortality and complications [11]. The ideal variables for haemodynamic management reflect end-organ perfusion and can be measured easily, accurately, reproducibly and continuously, or at least rapidly. Tissue hypoperfusion was one of the three strongest intraoperative predictors of postoperative complications in a study of 438 patients undergoing moderate-risk elective surgeries [12]. Tissue hypoxia has been found to play a central role in organ dysfunction [13], which in turn can compromise perioperative patient outcome [14]. However, tissue perfusion and tissue oxygenation are difficult to measure. Often, surrogate variables are used instead.
Even though most organs require blood flow rather than blood pressure [15], the most commonly used variable for managing haemodynamics is blood pressure. Blood pressure values vary with age [16]. USA data indicate that hypertension (>= 140–159/90–99 mmHg systolic/diastolic blood pressure) is prevalent in approximately 30% of the population, making it one of the most common chronic medical conditions [17, 18]. A meta-analysis of 30 observational studies associates preoperative hypertension with a 35% increase in risk of intraoperative cardiovascular complications [19].
However, evidence for optimal perioperative blood pressure targets is lacking [4, 20]. A systematic literature review found 140 different definitions of intraoperative hypotension in 130 articles, where the most frequent definition was a systolic blood pressure below 80 mmHg, a systolic blood pressure decrease of more than 20%, or a combination of absolute and relative thresholds (100 mmHg and 30% decrease) [21]. Patients presenting with chronic hypertension may have a shifted or impaired cerebral or renal autoregulation. Population-based blood pressure targets will likely not be adequate in these patients. Instead an individualized approach is needed, supported by monitoring of indicators that reflect end-organ perfusion [20].
A transient increase in blood pressure (by 20–30 mmHg) and heart rate (by 15–20 bpm) can be seen after laryngoscopy and intubation [22]. The blood pressure increase may be blunted by using opioids during induction. Anaesthetic agents can trigger a decrease in blood pressure and heart rate. Low blood pressure has also been observed in about a third of patients after spinal anaesthesia, one of the most common spinal anaesthesia complications [23]. Another frequent cause of perioperative hypotension is hypovolaemia due to dehydration or haemorrhage. A retrospective study in 33,330 non-cardiac surgeries found an increase in the risk of acute kidney injury or myocardial injury dependent on the duration of intraoperative MAP below 55 mmHg [24]. Surgical technique (e.g. laparoscopic insufflation), patient positioning and positive pressure ventilation can lower blood pressure by decreasing venous return and cardiac output.
Perioperative goal-directed therapy (PGDT) provides a rational, protocol-driven approach to haemodynamic optimization, often using IV fluids and vasoactive medications. A 2013 Cochrane review of 31 studies of PGDT in 5292 patients found that PGDT did not have an impact on mortality but that it did reduce postoperative renal failure, respiratory failure and wound infections [25]. Studies of GDT show a wide variety of protocols, endpoints and haemodynamic monitoring techniques [9]. The Surviving Sepsis campaign guideline recommends CVP 8–12 mmHg, MAP >= 65 mmHg, urine output >= 0.5 mL/kg/h, and central venous oxygen saturation (ScvO2) or mixed venous oxygen saturation (SvO2) 70% or 65%, respectively, and suggests using lactate levels as an indicator of tissue hypoperfusion [26]. Some studies have found HR’s association with outcome not to be consistent and to be confounded with other variables, such as patient presentation, beta-blocker medications and operative variables – raising doubts about the value of HR as a good variable for haemodynamic management [27, 28].
In their meta-analysis of proactive perioperative haemodynamic management trials, Hamilton et al [11] found in 29 studies target values similar to those recommended by the Surviving Sepsis campaign. These were CVP 6–15 mmHg, MAP of a minimum of 60–110 mmHg (average 74 mmHg) and maximum of 100–110 mmHg (average of 105 mmHg), urine output at least 0.5–1 mL/kg/h, and SvO2 minimally 65–70%. Only five of the reviewed studies used heart rate using a target of a maximum heart rate of between 100 to 120 beats per minute.
Of note, these surrogate variables, with the exception of SvO2 and ScvO2, may be normal, even when tissue hypoperfusion is present [29, 30]. In high-risk patients undergoing major surgery, 65% [31] and 73% [32] were found to be the optimal cut-off ScvO2 values to predictively discriminate complications. However, using ScvO2 to guide goal-directed therapy failed to improve mortality [30].
Indicators of the patient’s need for intravenous fluids include positive pressure variations (PPV) and stroke volume variations (SVV) with typical thresholds for indicating fluid responsiveness of 10–15% during closed chest positive pressure ventilation of at least 7 mL/kg [33–36].
Intraoperative events result in haemodynamic variable deviations from their normal range, as detailed in Table 14.3.
Monitoring
Invasive and non-invasive arterial blood pressure, central venous pressure, cardiac output and pulmonary capillary wedge pressure are the top five modalities used in North America and Europe during high risk surgery (Fig. 14.2) [3].
Fig. 14.2 Haemodynamic monitoring modalities used by North American and European anaesthesiologists for managing patients during high-risk surgery [3]. Legend: IBP (Invasive arterial pressure), CVP (Central venous pressure), NIBP (Non-invasive arterial pressure), CO (Cardiac output), PCWP (Pulmonary capillary wedge pressure), TEE (Transesophageal echocardiography), SPV (Systolic pressure variation), PWV (Plethysmographic waveform variation), PPV (Pulse pressure variation), ScvO2 (Central venous saturation), SvO2 (Mixed venous saturation), DO2 (Oxygen delivery), SVV (Stroke volume variation), NIRS (Near-infrared spectroscopy), GEDV (Global end diastolic volume).
Invasive arterial blood pressure monitors display the continuous blood pressure waveform and calculate systolic (SYS), diastolic (DIA) and mean arterial (MAP) blood pressure as well as heart rate. Similar setups with catheters connected to an external pressure sensor are used to measure pressures other than arterial, e.g. central venous pressure and pulmonary artery pressures.
Non-invasive arterial blood pressure monitors most commonly use an oscillometric cuff wrapped around an extremity, typically the upper arm. Measurements are intermittent, and should be limited in frequency to help avoid peripheral nerve injury from inflation of the cuff. Oscillometry is known to have limited accuracy, especially in patients with stiff arteries [37–39]. In a comparison of non-invasive and invasive arterial blood pressures in more than 24,000 cases, Wax et al found that non-invasive blood pressure was underestimated during hypertension and overestimated during hypotension [40].
Arterial tonometry and volume clamping allows continuous non-invasive blood pressure monitoring as well as display and derivation of the same or similar variables as invasive blood pressure monitoring. Cannesson et al systematically reviewed and analysed 29 studies of commercially available devices and found that their accuracy and precision did not meet the Association for the Advancement of Medical Instrumentation’s performance requirements (accuracy <= 5 mmHg and precision <= 8 mmHg) for non-invasive blood pressure monitors [41]. It might be that the requirements that target intermittent non-invasive blood pressure monitors are not appropriate for continuous non-invasive blood pressure monitors.
Most anaesthesiologists indicated in a recent survey [3] that they monitor central venous pressure (CVP) in high risk surgery cases. CVP is used as an indicator for volume expansion in these cases, together with blood pressure, urine output and clinical experience. This is in spite of the fact that more than 95% of anaesthesiologists acknowledge that CVP is not the best predictor for fluid responsiveness, and that there are ‘no data to support the widespread practice of using central venous pressure to guide fluid therapy’ [42].
The development of the Swann–Ganz pulmonary artery catheter (PAC) in the 1970s led to the widespread adoption of thermodilution cardiac output measurement – and eventually GDT. The PAC-based thermodilution method can be performed either intermittently by injecting room temperature or iced saline into the right atrium, or continuously, using a PAC with integrated heating filaments. The resulting change in temperature is measured downstream, in the pulmonary artery, and used to calculate cardiac output, assuming the indicator mixed completely with the blood and no indicator was lost. Accuracy is better the colder the indicator solution is and the lower the cardiac output is, because of a better signal to noise ratio. The PAC also allows measurement of pulmonary artery pressure, pulmonary artery wedge pressure and, with some PAC catheters, continuous monitoring of mixed venous oxygen saturation (SvO2). Evidence has amassed that data provided through use of the PAC, including thermodilution, is not accurate, that the PAC is associated with significant risk and poor outcome, that many clinicians are not able to correctly interpret information obtained through the PAC and that information may not help in the management of critically ill patients and in fact may result in overtreatment [43–46].
Less invasive and non-invasive cardiac output approaches have become available as alternatives to PAC-based thermodilution. Oesophageal Doppler cardiac output measures flow velocity in the descending aorta using a small-diameter dedicated ultrasonic probe inserted through the mouth or nose. Oesophageal Doppler can show beat-to-beat stroke volume, cardiac output, heart rate and a number of parameters derived from the flow velocity waveform. It assumes a homogeneous (or known) velocity profile across the aortic cross-section, a known cross-sectional area that stays constant over a heartbeat, a fixed and known ratio of cardiac output distribution between ascending and descending aorta and a correct positioning of the Doppler probe to make sure the ultrasonic beam is directed towards the descending aorta. Oesophageal Doppler cardiac output has been found in human and animal studies to be just slightly less accurate than thermodilution and associated with improved patient outcome [47].
Thoracic bioimpedance cardiac output measures the voltage drop across thoracic electrodes resulting from the injection of a small high-frequency electrical current across the thorax using two additional electrodes and measuring the resulting voltage drop. Assuming a certain bioimpedance model of the thorax and assuming that changes in impedance are related to changes in blood volume within the thorax, aortic flow rate and stroke volume are calculated. The approach is sensitive to the appropriateness of the thorax model, the placement of the electrodes, non-cardiac output related fluid shifts in the thorax and electrical noise. Variations of bioimpedance such as electrical velocimetry (relates impedance changes not to aortic flow but to aortic blood velocity) and bioreactance (considers the phase shift between electrical current and voltage drop) aim to ameliorate the sensitivity to electrode placement and thorax model. Studies of bioimpedance cardiac output agree that it lacks interchangeability with thermodilution but some indicate that bioimpedance might be successfully employed as a trend monitor and to judge fluid responsiveness [48].
In partial CO2 rebreathing cardiac output the volume of CO2 excreted through the lungs per breath is related to cardiac output. This method assumes that cardiac output, venous CO2 concentration and the slope of the CO2 dissociation curve stay constant during the measurement period and that end-tidal CO2 can be measured reliably and reflects alveolar CO2. Human and animal studies report mixed results. A meta-analysis by Peyton et al [49] found partial CO2 rebreathing to perform similarly to other non-invasive cardiac output approaches (oesophageal Doppler, bioimpedance and pulse contour). However, just like other non-invasive methods, it did not meet Critchley and Critchley’s postulated criteria of 30% or better agreement [50].
Pulse contour cardiac output analyses the continuous arterial blood pressure waveform and estimates cardiac output from vascular impedance and the area under the blood pressure curve during systole. Pulse contour cardiac output may become inaccurate if vascular impedance changes. Some of the commercially available pulse contour cardiac output monitors require occasional calibration, usually with less invasive methods, e.g. transpulmonary thermodilution or lithium dilution. A recent literature review of five commercially available pulse contour cardiac output monitors that use invasive blood pressure measurement found a total pooled underestimation bias of 40% and a standard deviation of the error of 1.25 L/min and only limited agreement with thermodilution during haemodynamically unstable periods. Frequent recalibration with thermodilution improved the accuracy of cardiac output estimations [51]. Another review showed that cardiac output estimated using non-invasive continuous blood pressure provides reasonable estimates of cardiac output, but does not reach the threshold for interchangeability with invasive thermodilution cardiac output [52].
Less invasive cardiac output indicator dilution approaches do not require a PAC. Instead, the indicator is injected through a central venous catheter and the thermodilution temperature curve is then measured in a large peripheral artery, e.g. the femoral or axillary artery. The shape of this transpulmonary indicator dilution waveform can then also be used to derive other variables of interest, such as extravascular lung water (EVLW), a measure of pulmonary oedema, and global end-diastolic volume (GEDV), an indicator of preload. EVLW estimation by transpulmonary thermodilution has been well validated and its ability to predict mortality in critically ill patients demonstrated in a number of studies [53]. In order to determine the need for fluid administration or fluid responsiveness, static preload indicators, such as central venous pressure, are inadequate. Static preload indicators do not consider where on the Frank Starling curve the heart is operating: preload is not the same as preload-responsiveness. In contrast, dynamic indicators involve changing the preload, and assessing the impact on stroke volume. Preload is changed as a matter of course by positive pressure ventilation and its impact on venous return. A mix of spontaneous and mechanically provided breaths will compromise the performance of this approach. This method assumes that the transmission of airway pressure to the thorax is not impeded, e.g. through low lung compliance or open chest or laparoscopic surgery. SVV might be assessed directly using continuous cardiac output approaches, through changes in pulse pressure measured from a continuous invasive or non-invasive blood pressure waveform (PPV), or through changes of the plethysmographic waveform, for example from a pulse oximeter. For these approaches, a trend monitor is sufficient; they do not require an absolute measurement. A systematic review encompassing 29 studies of mechanically ventilated patients demonstrated high sensitivity and specificity for predicting fluid responsiveness using respiratory variations of pulse pressure or stroke volume [54].
Oxygen saturation of haemoglobin helps determine the blood’s oxygen carrying capacity of arterial blood, tissue oxygenation. The utility of pulse oximeters that measure arterial oxygen saturation have been expanded by adding an indicator of peripheral perfusion, measurement of carbon monoxide and methaemoglobine saturation, absolute haemoglobin, and respiratory rate.
Venous oxygen saturation can be measured optically and continuously in mixed venous blood (SvO2) of the pulmonary artery by some of the modern PACs. It can be used to gauge, at constant SpO2, how much oxygen the body has extracted. As an alternative, if PAC use is not indicated, central venous oxygen saturation (ScvO2) can be measured, instead, at the tip of the central venous catheter. Because the relationship between ScvO2 and SvO2 is not constant they cannot always be interchanged with each other [55].
Near-infrared spectroscopy (NIRS) approaches have been developed that allow non-invasive monitoring of cerebral oxygenation. Cerebral oximetry measures non-pulsatile average tissue oxygenation, with a majority signal contribution from venous blood (approximately 70%) and the balance from arterial blood. Factors that might affect accuracy include changes in haemoglobin, haematoma, skull thickness and cerebrospinal fluid area, deviation from the assumed 70/30% ratio of venous to arterial signal contribution and jaundice.
Because of wide ranges of normal cerebral tissue oxygenation (60–75%), a coefficient of variation of the absolute baseline of 9.4% [56], and the large variability of algorithmic differences, cerebral oximetry is best used as a trend monitor. Thresholds between 12% and 20% decrease from baseline have been reported to be able to predict clinical symptoms of cerebral ischaemia during carotid surgeries. In an intervention algorithm based on cerebral oxygenation monitoring proposed by Denault et al [57] a decrease of cerebral oxygenation of 20% or more triggered an in-depth inspection of other haemodynamic parameters. The evidence for improved outcome from use of cerebral oximetry is still elusive, however [58].
Left Ventricular Echocardiography
Perioperative physicians use transthoracic (TTE) and transoesophageal echocardiography (TEE) in the operating room, emergency room and critical care settings. Evaluation of global LV systolic function is a universal requirement. No other bedside diagnostic technique offers the immediacy or comprehensiveness that echocardiography provides for this evaluation.
The most common and clinically useful LV echocardiographic modalities are fractional area change, fractional shortening and myocardial velocity assessment. In urgent clinical situations, experienced practitioners estimate LV systolic function qualitatively recognizing that only very marked abnormalities produce life-threatening consequences.
Fractional area change: the fractional area change (FAC) of the LV may be obtained with any of a number of different cross-sections, but the transgastric (TG) mid-ventricular short-axis (SAX) is usually the best choice because foreshortening of the left ventricle is a common problem with the TEE long-axis cross-sections. The simple formula for this calculation is:

where EDA is the end-diastolic area and ESA is the end-systolic area. Usually, the result is expressed as a percentage. Software packages provide an easy way to make these measurements by tracing the endocardial borders in both systole and diastole. By convention, the papillary muscles are included within the area measurements. Although this measurement is obtained easily, its interpretation has inherent limitations. For example, when FAC is measured using the TG mid-ventricular cross-section, segmental wall motion abnormalities (SWMA) in the ventricular base or apex will lead to overestimation of global LV function. Also, FAC is dependent on ventricular preload and afterload. Thus, changes in FAC may not reflect changes in LV global function unless loading conditions remain constant.
Fractional shortening (FS) is analogous to FAC in that it measures the change in dimension (instead of cross-sectional area as used in FAC) occurring during systole. The equation for this measurement is:

where LVED is the LV end-diastolic diameter and LVES is LV end-systolic diameter. FS was the principal measure of LV function in the early days of echocardiography when only M-mode technology was available. However, it is still a commonly cited measurement in reports and studies. The TG mid-ventricular cross-section is the most useful for this measurement with the M-mode cursor placed across the myocardium of interest. FS has the same limitations as FAC when used for the assessment of global LV function. In fact, given the one-dimensional nature of M-mode, FS is less likely than FAC to reflect global ventricular function in the presence of any SWMA. Unlike the textbook values, Table 14.4 provides normal values for anaesthetized patients [59]. Wall thickening is included for completeness.
Three-dimensional (3-D) systems capture real-time end-diastolic and end-systolic volumes and allow measurement of ejection fraction and stroke volume. However, these systems are expensive and complicated to operate. Like the parameters in Table 14.4, ejection fraction and stroke volume measurements are impacted substantially by changes in LV preload and afterload.
Doppler tissue imaging: Doppler tissue imaging (DTI) uses pulsed-wave Doppler technology adapted to measure myocardial velocities instead of blood flow velocities [60]. Typically, myocardial velocities range between +20 and –20 cm/s. During normal LV contraction, the mitral annulus descends toward the apex of the heart. DTI measures the velocity of this descent (Sʹ). Sʹ correlates with traditional measures of LV function including ejection fraction and the rate of rise of LV systolic pressure (dP/dt) [60, 61]. In addition, Sʹ decreases in the presence of myocardial ischaemia and responds to changes in inotropy [62, 63]. The four chamber cross-section is best for measuring Sʹ: the sampling volume is placed at the lateral insertion point of the mitral valve into the left ventricle and the cross section is aligned so that the sampling cursor is directly parallel with the motion of the annulus. The resulting Doppler tracing shows the downward Sʹ systolic wave caused by the descent of the base of the LV as well as two upward diastolic waves Eʹ and Aʹ. These waves are caused by the upward motion of the base of the LV associated with the early and late phases of left atrial filling. Sʹ is an easy and reproducible measurement. However, Ama et al tested its load dependence in 42 haemodynamically stable patients with normal segmental LV function after coronary surgery. Sʹ did not change significantly in response to a 20% increase or decrease in MAP induced by phenylephrine or nitroglycerine [64]. However, it increased significantly when preload was augmented by rapid infusion of colloid [63]. In summary, Sʹ is less dependent on afterload than FAC and FS, but like these other two measures, it remains critically dependent on preload. However, DTI provides a quick and easy way to estimate LV ejection fraction: an Sʹ greater that 7 cm/s usually correlates with an LV ejection fraction ≥ 50% [65]. This correlation was established using TTE. TTE estimates of S′ may be somewhat greater than TEE measurements because with TTE the Doppler beam and annular motion may be better aligned. However, Sevimli et al estimated that difference to be less than 2% [66]. Clearly, in an individual patient, if the TEE Doppler beam and annular motion are off parallel alignment by more than 30°, you should expect significant underestimation. Likewise, if a segmental wall motion abnormality affects annular motion, the Sʹ may not reflect global LV systolic function.
Right Ventricular Echocardiography
Traditionally, echocardiography has focused more on the evaluation of LV function and the RV has often been termed ‘the forgotten ventricle’. Barring significant intra-cardiac shunt, however, these two pumps in series must provide the same cardiac output and therefore the function of the right ventricle is equally important as the function of the left.
Classic teaching about RV failure in the setting of myocardial infarction (MI) has been to give the patient IV fluids, elevate their CVP and turn the RV into a ‘conduit’ for passive blood flow. Outcome studies, however, showed that patients with right-sided MI had significantly greater morbidity and mortality than those with left-sided MI [67] and, recently, the importance of RV dysfunction has been demonstrated in patients with systolic heart failure [68], in right-sided MI [69] and in those undergoing cardiac surgery [70–72]. Objective measures of RV function are therefore becoming a more essential component of the echocardiographic exam.
The biggest challenge to the echocardiographic assessment of RV function is its complex geometric shape. The LV has a simple bullet shape that is amenable to two-dimensional (2-D) modelling and allows relatively accurate approximations of ejection fraction (EF) from 2-D images. The RV, on the other hand, has a relatively complex crescentic shape and 2-D approximations of RV volumes and EF are not valid [73]. The objective evaluation of the RV, therefore, has relied on surrogate measures of function such as tricuspid annular plane motion and cross-sectional area change.
The most widely used measure of RV function in echocardiography is the tricuspid annular plane systolic excursion (TAPSE), which is a measure of the distance the tricuspid valve annulus is displaced during systole. A four-chamber view of the heart is obtained and the motion of the tricuspid annulus is tracked over time using M mode echocardiography. The vigorous contraction of a normal healthy RV results in a tricuspid annular excursion of around 2–3 cm. A TAPSE < 1.7 cm suggests RV dysfunction [74]. Another common measure of RV function is the peak velocity of the tricuspid annulus as it descends from the base of the heart during systole (S’). This velocity is measured using TDI and an S’ < 9.5 cm/s suggests RV dysfunction [74].
These two measures of tricuspid annular motion are the most commonly reported objective measures of RV function with transthoracic echocardiography (TTE). When compared to the 3-D assessment of the RV using MRI, however, these surrogate measures of RV function show poor correlation with RV EF [75], most likely because they assess the function of only a single segment of only one of the four walls of the RV. In addition, geometric changes that occur with the heart after pericardiotomy appear to result in a decrease in TAPSE that persists for greater than 12 months after cardiac surgery even though 3-D measures of RV ejection fraction are unchanged [76], suggesting that TAPSE may not be a valid measure of RV function after cardiac surgery. Finally, measurements of systolic excursion and tissue Doppler based velocity are highly angle dependent. Whereas the angle of the ultrasound beam aligns well with tricuspid annular motion in a four-chamber view using TTE, this is not true of the corresponding measurement in TEE. These measures of RV function, therefore, should probably not be used for the intraoperative assessment of RV function.
Two additional echocardiographic measures of RV function are fractional area change (FAC) and myocardial performance index (MPI). The FAC is percentage change in the cross-sectional area of the RV through the cardiac cycle. Analogous to EF, FAC is calculated by subtracting the end-systolic area of the RV in a four-chamber view from the end-diastolic area and then normalizing it by the end-diastolic area. RV dysfunction is suggested by an RV FAC < 35% [74]. Despite being a 2-D estimation of 3-D EF, RV FAC has been shown to correlate better with RV 3-D EF than either TAPSE or S’ [75], can be used intraoperatively with TEE and is a significant predictor of outcome after cardiac surgery [71].
Myocardial performance index (MPI) is a measure of the ratio of duration of the isovolumic phases of ventricular contraction and relaxation to the duration of the systolic ejection period. A healthy RV will have relatively short isovolumic times and a relatively long ejection period making this ratio a small number. In RV dysfunction, the isovolumic times are prolonged and the ejection period shortens. MPI can be measured using spectral Doppler of a tricuspid regurgitation jet and the right ventricular outflow tract ejection. Alternatively, simultaneous measurements of MPI can be made using TDI. RV dysfunction is suggested by a pulsed wave Doppler MPI > 0.43 or a tissue Doppler MPI > 0.54 [74]. MPI has also been shown to predict outcome after cardiac surgery [70] and is applicable to both TTE and TEE.
Two relatively new measures of RV function are speckle-tracking strain and 3-D RV EF measured with echocardiography. Both of these methods require specialized software that was initially only offered offline on a separate computer. Recently, however, major manufacturers have started making these software applications available on the echo machine itself such that these calculations can now be made during image acquisition or in the operating room.
Speckle-tracking strain echocardiography uses echogenic ‘speckles’ in the myocardium to track movement of the myocardium over time [77, 78]. RV strain is calculated as the percentage of myocardial shortening during systole. RV global longitudinal strain is the average strain of the three segments of the lateral wall in a four-chamber view and the three segments of the inter-ventricular septum. The septum is included because of the contribution it is thought to make to RV ejection and cardiac output. RV free wall strain (RV FWS) is the same measurement; only the inter-ventricular septum values are removed and thus it is the average strain of the three segments of the lateral wall of the RV. This measure is analogous to TAPSE except that speckle-tracking echocardiography is relatively angle independent and instead of only measuring the excursion of the tricuspid annulus, it measures myocardial shortening of the entire lateral wall of the RV. RV FWS is a promising new technology that is the best predictor of RV dysfunction when compared to more traditional echocardiographic measures of RV function [75]. RV strain has also been shown to predict outcome in patients with systolic heart failure [68] and right-sided MI [69], and those undergoing cardiac surgery [72].
Three-dimensional echocardiography is not a new technology. However, only in the last decade has computer processing on echo machines been fast enough to incorporate this technology into day-to-day practice. Most new echo machines have the capability of acquiring 3-D images of the heart and when applied to the RV, volumetric data can be obtained and then used to calculate 3-D EF. TTE measurements of 3-D RV EF have been shown to correlate well with the gold standard of cardiac MRI [79]. 3-D RV EF has also been shown to be feasible to measure with TEE in the operating room [80], although the software to make these calculations is expensive and not available on most echo machines.
To summarize, despite its reputation as ‘the forgotten ventricle’, multiple studies now demonstrate the importance of RV function in patients with cardiovascular disease. The assessment of RV function is difficult because the complex shape of the RV precludes accurate estimations of ejection fraction using 2-D echocardiography. Surrogate measures of RV function such as TAPSE and S’ are often used, but these have limitations, particularly when used with TEE in the operating room. Newer measures such as RV strain and 3-D RV EF show promise in terms of their diagnostic accuracy but require specialized software. Hopefully over time, this new software will become less expensive and more available to end-users in the operating room.
Closed Loop
As discussed, it is hard to set and justify specific absolute haemodynamic target values for the individual patient based on population statistics. When appropriate target values have been determined by the clinician, closed-loop systems might help reaching and maintaining these values automatically. Academic research going back decades [81] has shown systems that have demonstrated lower inter-patient variability for fluid management [82], more consistent and stable control of blood pressure [83] and good performance of a number of other haemodynamic closed-loop systems [84]. However, hardly any device has taken the requisite regulatory and safety engineering steps to make it to the market, with one of the notable and rare exceptions being the GenESA closed-loop system for the control of a synthetic beta agonist for cardiac stress test applications [85]. Closed-loop and autonomous systems are rapidly emerging even in areas in which safety is a top priority. It may be expected that such systems will find their way into anaesthesia and perioperative haemodynamic management eventually [86].
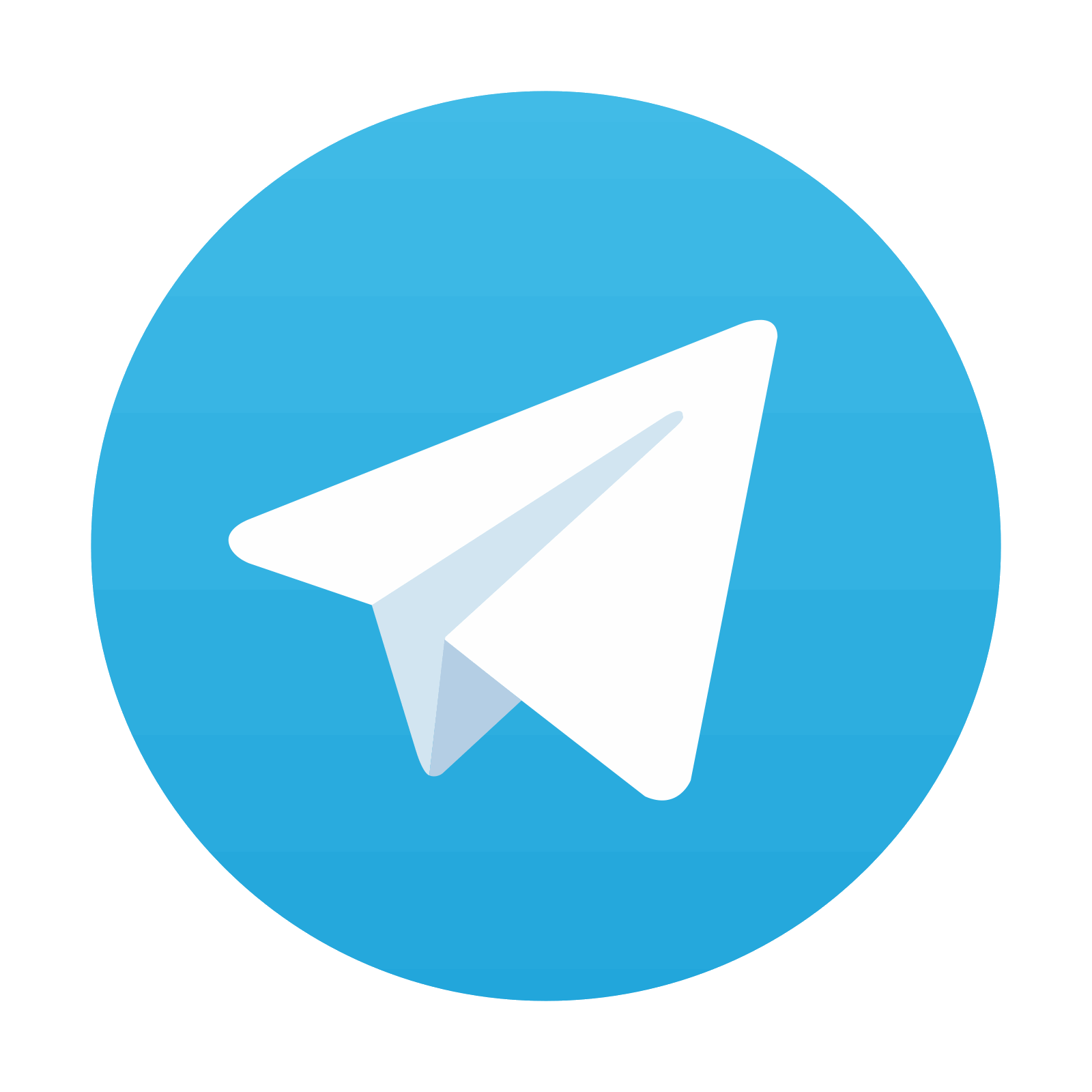
Stay updated, free articles. Join our Telegram channel
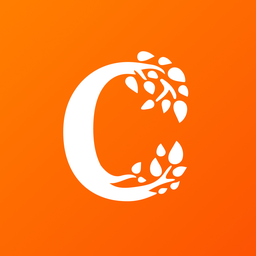
Full access? Get Clinical Tree
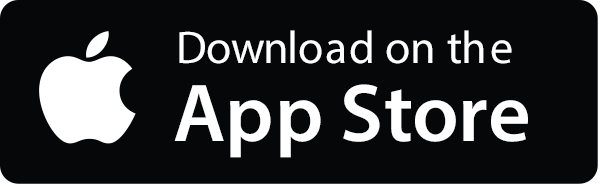
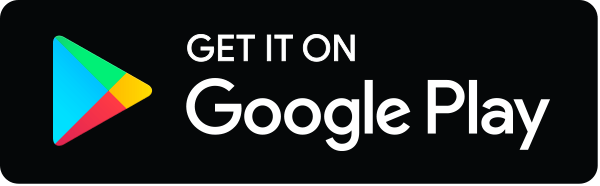