Abstract
Surgical anaesthesia usually requires hypnosis, antinociception (ensuring blood pressure and heart rate control) and immobility with varying degrees of muscle relaxation. However, the relative contribution of these three components to the state of anaesthesia may vary between different anaesthesias and surgical procedures. While volatile anaesthetics may be used to produce anaesthesia in and by themselves, most often anaesthesia is produced by a combination of drugs. Anaesthesia produced by the concomitant use of hypnotics, analgesics and neuromuscular blocking drugs is called ‘balanced anaesthesia’.
Muscle Relaxation and Neuromuscular Block
Surgical anaesthesia usually requires hypnosis, antinociception (ensuring blood pressure and heart rate control) and immobility with varying degrees of muscle relaxation. However, the relative contribution of these three components to the state of anaesthesia may vary between different anaesthesias and surgical procedures. While volatile anaesthetics may be used to produce anaesthesia in and by themselves, most often anaesthesia is produced by a combination of drugs. Anaesthesia produced by the concomitant use of hypnotics, analgesics and neuromuscular blocking drugs is called ‘balanced anaesthesia’.
The degree of neuromuscular block induced by neuromuscular blocking drugs and the depth of anaesthesia (induced by other drugs) both affect muscle relaxation [1]. While in the early days of our profession deep ether anaesthesia alone provided sufficient muscle relaxation for many procedures, the introduction of neuromuscular blocking drugs nowadays allows us to target specific degrees of muscle relaxation in an easy and safe manner. Provided the airway is secured and adequate ventilation and hypnosis are ensured, adverse effects of neuromuscular blocking drugs are few.
After briefly reviewing pertinent physiology, the different degrees of neuromuscular block depth are defined and the tools to measure them are described. The chapter then focuses on the properties of modern neuromuscular blocking drugs (only cisatracurium, mivacurium and rocuronium will be discussed) and suxamethonium. Finally, the clinically optimal neuromuscular block is defined, and the different routes to achieve this goal are explored.
Neuromuscular Junction
An action potential causes synaptic vesicles of the motor nerve endings containing the neurotransmitter acetylcholine to fuse with the plasma membrane and release their content into the neuromuscular junction. Acetylcholine then binds to postjunctional nicotinic acetylcholine receptors on the motor endplate. These receptors consist of two alpha and one beta, delta and epsilon subunits. They are directly linked to ion channels. If acetylcholine is bound to both alpha subunits, the permeability of the ion channel for Na+ and K+ ions increases, depolarizing the muscle cell [2]. Subsequent calcium release causes contraction of the cell, and if a sufficient number of muscle cells are depolarized simultaneously, muscle contraction ensues.
In addition to the postjunctional receptors, there are also prejunctional acetylcholine receptors that are involved in the modulation of acetylcholine release in the neuromuscular junction. They consist of three alpha and two beta subunits. While clinically used non-depolarizing neuromuscular blocking drugs have affinity for these presynaptic receptors, the only available depolarizing neuromuscular blocking drug, suxamethonium, does not [3]. Because a non-depolarizing neuromuscular block displays fade after train-of-four stimulation (see further) while a suxamethonium induced neuromuscular block does not, it is plausible to explain this by the aforementioned different effects on presynaptic nicotinic acetylcholine receptors. Fade is used to quantify the degree of neuromuscular block elicited by the non-depolarizing neuromuscular blocking drugs.
Quantification of Neuromuscular Block
Because the individual response to neuromuscular blocking drugs varies to such an extent that it becomes unpredictable in the individual patient, neuromuscular function has to be measured continually during anaesthesia. Fortunately, of all components making up anaesthesia, the degree of the neuromuscular block is the one that can be measured most easily by applying a supramaximal electrical stimulus to a peripheral motor nerve and quantifying the subsequent muscle contraction (or other muscle response). A complete or partial neuromuscular block is said to exist if no or some (but not a full) response is present, respectively.
After administration of a neuromuscular blocking drug, the degree of neuromuscular block is not uniform in all muscles throughout the body. For example, laryngeal muscles achieve peak effect faster than peripheral muscles but the effect there also dissipates faster. Both pharmacokinetic (PK) (e.g. blood flow) and pharmacodynamic (PD) (sensitivity) factors account for these differences. These differences have to be taken into account when monitoring neuromuscular block in one muscle (e.g. because of ease of access) to get an idea of the neuromuscular block in another muscle that may be the more relevant one to ensure optimal surgical conditions – it explains why the quantification of neuromuscular block at the hand is not perfectly informative for neuromuscular block at diaphragm or abdominal wall [4].
Stimulation Patterns
While quantifying the muscle response following electrical stimulation of a motor nerve seems straightforward, in reality it is a rather complex subject.
While a number of stimulation patterns are in widespread use, the type of electrical stimulation is often quite similar. Typically, a unipolar constant-current pulse is used, and this current has to be strong enough to evoke a supramaximal response from the muscle. The reason for the need of supramaximal stimulation is to ensure that the magnitude of muscle contraction is independent of the intensity of the electrical stimulation. If all of the motor units innervated by the stimulated nerve are recruited, then we have supramaximal stimulation. The magnitude of the contraction then (ideally) becomes independent of the magnitude of the electrical stimulus. Under these conditions, the only limiting factor in muscle contraction is the neuromuscular transmission, which is the characteristic of interest. This method also reduces the dependence of muscle contraction on stimulating electrode conditions which can vary during the surgical procedure. Moisture or gel under the electrodes changes the electrical properties of the skin under the electrodes. Typically, constant-current pulses of up to 50–60 milliamperes are used with duration of 200–300 microseconds.
Ideally, both the stimulation site, i.e. the site where electrodes are placed, and the monitoring site, i.e. the site where muscle response is registered, have to remain easily accessible throughout the surgical procedure. Because during most surgical procedures at least one hand is accessible, the ulnar nerve at the wrist and the adductor pollicis it innervates are the most commonly used monitoring sites. When this site is not accessible, the orbicularis oculi or corrugator supercilii at the eye, and the flexor hallucis brevis at the foot can be used. Accessibility of the area of the motor nerve is important for the initial electrode placement, but once the electrodes are placed, often the site does not need repeated access.
Single-twitch Stimulation
Single-twitch stimulation refers to the application of a single electrical pulse to a motor nerve, with quantification of the resulting muscle contraction called ‘single-twitch response’. Before the administration of neuromuscular blocking drugs, a ‘control twitch’ is determined that serves as the baseline muscle contraction to which subsequent twitches are referenced. A value of 0% indicates complete neuromuscular block, and 100% indicates no neuromuscular block. While single-twitch stimulation has been used extensively in older research, it has only been rarely applied in routine clinical practice. A proper understanding of this method helps the clinician to gain insight into much of the research on neuromuscular blocking drugs.
The interpretation of the single-twitch stimulation response is confounded by ‘twitch potentiation’, a physiological phenomenon whereby repetitive stimulation can cause a gradual increase in twitch response that ultimately plateaus. To make matters even more complex, the magnitude of the control twitch response may also depend on the stimulation pattern. Because intraoperative neuromuscular transmission monitoring requires repetitive stimulation of the nerve, post-relaxation twitch responses, defined as the responses during and after the recovery of neuromuscular block, will be potentiated and plateau at some higher value. If insufficient time is taken for twitch response stabilization prior to the administration of neuromuscular blocking drugs, twitch potentiation during and after recovery of a drug-induced neuromuscular block can result in a twitch response that exceeds the control twitch, obtained prior to the administration of neuromuscular blocking drugs, by 150–180%. In these conditions, a twitch response of 100% of the control twitch will falsely indicate full recovery and underestimate a residual block that may still cause the patient to experience clinical signs of residual neuromuscular block.
‘Pre-relaxation twitch stabilization’ will eliminate ‘twitch potentiation’ as a confounding factor when interpreting twitch responses after the administration of neuromuscular blocking drugs, but reaching a plateau can require up to 30 min of 0.1-Hz single-twitch simulation. Increasing the stimulation frequency can speed up the rate at which a plateau is reached but may also affect the ultimate plateau height as well. Careful balancing of these effects can be helpful in achieving a stable ‘control’ twitch response before the administration of neuromuscular blocking drugs. A two-second 50-Hz tetanic stimulus followed by two minutes of 0.1-Hz stimulus has been suggested for reasonably rapid twitch stabilization [5, 6]. Some researchers create a mathematical model of the postsynaptic process that causes a rise towards a plateau and incorporate it in their models of muscle relaxation [7].
Train-of-four Stimulation Pattern
The difficulties of obtaining and interpreting the ‘control-twitch’ for single-twitch stimulation have led to the development of the train-of-four (TOF) stimulation pattern. This stimulation pattern consists of four electrical pulses separated by 0.5 seconds, with each train usually separated by 12–20 seconds. As the depth of a non-depolarizing neuromuscular block increases, the fourth twitch will diminish first because the previous three stimuli have depleted the acetylcholine content of the presynaptic vesicles, causing a preponderance of the competitive inhibitor at the neuromuscular junction. As the concentration of the competitive inhibitor (the non-depolarizing neuromuscular blocking drug) further increases and the block deepens, the third, second and first twitch responses also decrease (in that sequence, because less and less acetylcholine is available during each subsequent pulse in the TOF sequence). The origin of the TOF ‘fade’ is thought to be a prejunctional effect of non-depolarizing neuromuscular blocking drugs.
The TOF stimulation pattern eliminates the need for a pre-relaxation control twitch because it references the magnitude of the fourth to the first twitch and uses this ratio to quantify the depth of a neuromuscular block. This ratio is known as the TOF ratio. A TOF ratio of 100% indicates the absence of neuromuscular block and 0% indicates that the fourth twitch has disappeared, suggesting a moderately deep level of neuromuscular block. At deeper levels, the third, second and first twitches become progressively smaller and ultimately disappear. As recovery from neuromuscular block occurs, the twitches reappear in order and when the fourth twitch reappears the TOF ratio can be again calculated. Even with a TOF ratio of one, a significant number of acetylcholine receptors may be blocked. It reflects the considerable margin of safety of neuromuscular transmission: up to 80% of receptors may be blocked without a noticeable effect on muscle strength. It also explains why the top-up dose of a neuromuscular blocking drug to maintain a certain block is only a fraction of the initial dose (see further).
When the fourth twitch disappears and the so-called TOF count becomes three (out of four) and the TOF ratio becomes 0%, a ‘moderately deep’ block is said to exist. Once the TOF ratio becomes zero, the ratio itself cannot be used to quantify deeper blocks, and the clinician reverts to counting the number of detectable twitch responses after TOF stimulation, with a TOF count of three, two, one and zero indicating a progressively deeper block. A TOF count of zero or one corresponds to moderately deep neuromuscular block and indicates that considerable time must elapse before the fourth twitch will reappear and a TOF ratio can be calculated.
Tetanic Stimulation Pattern
Even when the TOF count is zero, deeper levels of neuromuscular block can be monitored still with a post-tetanic count (PTC) stimulation pattern. To obtain a PTC, a supra-maximal tetanic stimulation pattern of 50 Hz for five seconds is followed by a three-second pause, after which a single-twitch 1 Hz stimulus is applied for 15 seconds. The number of muscle responses to the 1 Hz stimuli is related to the time of reappearance of the first twitch of a TOF stimulus [8].
Tetanic stimulation overcomes a deep non-depolarizing block by inducing by a process called ‘post-tetanic facilitation’: tetanic stimulus induced exhaustion of presynaptic vesicles accelerates recycling and synthesis of acetylcholine in the prejunctional synapse, a process that actually overshoots in the sense that a larger than normal amount of acetylcholine is released after a subsequent single twitch. This excessive amount of acetylcholine succeeds in overcoming a deep (competitive, non-depolarizing) neuromuscular block. Very deep levels of neuromuscular block can be quantified in this manner. A PTC of one to two suggests that considerable time is still needed before reappearance of the first twitch response to a TOF stimulus. The PTC pattern is typically preceded by a number of TOF stimulations and the tetanic stimulation of the PTC is only applied if no detectable responses are observed.
Quantification of the Response to Stimulation: The Muscle Response
Following stimulation of a motor nerve, muscle response can be detected and quantified in a number of ways. Each method has various advantages and disadvantages.
Manual or Visual Estimation
The clinician can simply feel or observe the muscle contraction. However, manual estimation is not particularly accurate – even experienced researchers cannot observe fade at TOF ratios higher than about 40% [9]. Because current standards mandate that TOF ratios exceed 90% [10, 11] at the end of a procedure, manual or visual estimation is insufficient to judge whether recovery is adequate. Manual estimation is acceptable to monitor deep levels of neuromuscular block with the PTC stimulation pattern. It is generally accepted though that state-of-the-art neuromuscular monitoring requires objective quantification of muscle contraction.
Mechanomyography
Mechanomyography (MMG) measures the peak isometric muscle force after supramaximal neuromuscular stimulation. MMG is considered the ‘gold standard’ of neuromuscular monitoring because it is the generation of muscle force that maintains the airway and effective contraction of the diaphragm [12]. However, despite its importance, there are no commercially available devices that use isometric MMG neuromuscular monitoring. MMG has been used for research purposes though.
Typically, the peak isometric muscle force of the thumb’s adductor pollicis is measured after stimulating the ulnar nerve at the elbow or at the wrist. MMG requires the associated limb to remain immobile. The thumb is held immobile by fixing the forearm to an arm-board, and force is measured using a strain gauge at the thumb [13]. The resting tension of the muscle or ‘preload’ influences the muscle force that is generated – it is generally accepted that a preload associated muscle length of 100–125% of resting length generates maximum muscle force. At the adductor pollicis, this corresponds to a preload of approximately 200 grams, and ideally this should vary less than 25% throughout the period of neuromuscular monitoring. Obviously, the force of the preload is not exclusively conveyed to the muscle – some force is transferred to connective tissues as well. The geometry of any joints of the system also plays a role in the force produced. A drawback of the arm-board system is that subtle changes in patient positioning can change the placement of the forearm within the arm-board, which changes the resting tension, which in turn affects interpretation of the observed muscle force.
Non-isometric Mechanomyography
Related to MMG monitoring is mechanical sensing of the muscle response. In this approach muscle contraction causes bending or compression of a piezoelectric sensor. It is used in the clinically widely applied NMT MechanoSensor® (GE Healthcare, Finland) (Fig. 11.1). The transducer is available in adult and paediatric sizes. Muscle preload cannot be evaluated because this depends on the mechanical properties of the transducer and how it matches with the individual anatomy. However, this approach does make attaching the device to the patient considerably simpler than for isometric systems, is much less sensitive to variation in patient positioning, and – even though it does not measure isometric muscle force – is sufficiently accurate for clinical applications.
Acceleromyographic Measures
If the limb associated with a muscle is free to move when the motor nerve is stimulated, then the movement of the limb can be also used to quantify muscle response. Acceleration is related to muscle force through Newton’s second law, force = mass × acceleration. Because mass remains constant, the peak acceleration must be proportional to the peak muscle force resulting from the activation of the motor nerve. Accelerometers are robust, wear and tear free, and inexpensive to manufacture, making them well suited for the harsh clinical environment. They are also small, allowing considerable flexibility in how they can be placed: even though most are commonly used to measure thumb adduction, they can also be applied to the obicularis occuli, corrugator supercilii and the flexor hallucis brevis muscles. Many patient monitors have integrated modules which quantify the degree of neuromuscular block with accelerometry.
Electromyographic Measures
Prior to generating force, action potentials propagate throughout the muscle, initiating calcium release from the sarcoplasmic reticulum. These action potentials can also be detected and used to quantify the muscle’s response to neuromuscular stimulation. The summation of the action potentials can be recorded using electrodes placed over the belly of the activated muscle. The peak-to-peak amplitude or the integration of the surface underneath the electromyographic (EMG) signal can be used to quantify muscle response. The ulnar nerve is stimulated at the elbow or wrist and the EMG response captured with electrodes placed over the adductor pollicis (at the thenar eminence), the abductor digiti minimi (at the hypothenar eminence), or at the first dorsal interosseus muscle. An active recording electrode should be placed over the muscle and a ground electrode between the stimulation and the recording electrodes. Sources of artefacts are multiple. External pressure on or moving of the electrodes and the arm can make the EMG quantification unreliable, but can be avoided by properly shielding and immobilizing the arm and electrodes. Changes in temperature, electrode conditions (due to, for example, absorption of electrode gel and sweating) and monopolar diathermy can all interfere with EMG monitoring.
Neuromuscular diseases can make EMG neuromuscular monitoring problematic. Volatile anaesthetics can impair muscle function and force generation without having an effect on the EMG signal [14, 15]. In general, MMG and AMG rather than EMG are often preferred as techniques to monitor the degree of a neuromuscular block because they measure the actual movement in muscles.
Pharmacology of Neuromuscular Blocking Drugs
Several drugs can prevent the contraction of skeletal muscles at the neuromuscular junction by inhibiting the synthesis or release of acetylcholine or by blocking its actions at the postjunctional membrane. Clinically used neuromuscular blocking drugs mainly act by the latter mechanism, inhibition of the postjunctional actions of acetylcholine at the nicotinic acetylcholine receptor. Two classes of neuromuscular blocking drugs exist, non-depolarizing and depolarizing. Only one depolarizing drug is clinically used, suxamethonium (also called succinylcholine). These two drug classes have different properties.
All neuromuscular blocking drugs are quaternary ammonium compounds that hold acetylcholine somewhere in their chemical structure. The quaternary ammonium (NH4+) prevents their absorption from the gastrointestinal tract, placenta and into the cerebrospinal fluid (CSF). Because of these chemical properties, neuromuscular blocking drugs are not widely distributed in the body and their volume of distribution (0.1–0.5 L/kg) is rather close to the volume of extracellular fluid [17].
Non-depolarizing Neuromuscular Blocking Drugs
Mechanism of Action
Chemically, non-depolarizing neuromuscular blocking drugs are either benzylisoquinolones (cisatracurium, mivacurium) or aminosteroid derivatives (rocuronium). They are competitive inhibitors of acetylcholine at the postjunctional nicotinic receptor. If they occupy one or both alpha subunits, ion channel opening is prevented and neuromuscular transmission becomes impaired. At higher concentrations they can also block the channel directly, in a non-competitive action. However, this so-called ‘channel block’ is of minor clinical significance [16].
Adverse Effects
The action of non-depolarizing neuromuscular blocking drugs on nicotinic receptors outside the neuromuscular endplate or on muscarinic receptors explains many of their adverse effects. Cisatracurium, mivacurium and rocuronium have essentially no effects on nicotinic receptors in the autonomic ganglia (Table 11.1). Via an effect on the vagal nerve, rocuronium can activate cardiac muscarinic receptors, but tachycardia will only ensue at a dose which is three- to five-fold higher than a usual dose for endotracheal intubation. Benzylisoquinolones can cause serosal mast cells to release histamine, but this effect is mild with mivacurium and absent with cisatracurium. Overall, the incidence of serious adverse effects is low. However, of all intraoperative life-threatening anaphylactic or anaphylactoid reactions (1 in 10,000 to 20,000 anaesthetics in a French study), 60% are caused by neuromuscular blocking drugs [17].
Table 11.1. Effect of commonly used neuromuscular blocking drugs on the autonomic nervous system and histamine release and their basic PK and PD properties.
Drug | Chemical structure | Autonomic Nervous System and Histamine Release | Basic PK and PD Properties | ||||||
---|---|---|---|---|---|---|---|---|---|
Autonomic ganglia | Cardiac muscarinic receptor | Histamine release | ED95 (mg/kg) | Rate of infusion required to maintain neuromuscular block at 95% (μg/kg/min) | Time to peak effect following 2 × ED95 (min) | Time to T1 = 25% of control following 2 × ED95 (min) | Renal clearance (% total clearance) | ||
Cisatracurium | Benzylisoquinoline | None | None | None | 0.05 | 1–2 | 5.2 | 20–50 | 16 |
Mivacurium | Benzylisoquinoline | None | None | Slight | 0.08 | 3–15 | 3 | 10–20 | < 5 |
Rocuronium | Aminosteroid | None | Blocks weakly | None | 0.3 | 9–12 | 1.5 | 20–50 | 10–25 |
Suxamethonium | Diacetylcholine | Stimulates | Stimulates | Slight | 0.3 | – | 1.4 | < 10 | < 2 |
ED95 is the dose which is required to induce a 95% neuromuscular block (the height of the first twitch in the TOF sequence reaches 5% of the height of the control twitch obtained before the administration of neuromuscular blocking drugs).
Pharmacokinetics and Pharmacodynamics of Non-depolarizing Neuromuscular Blocking Drugs
Essential basic PK/PD characteristics are summarized in Table 11.1. Renal clearance accounts for 10–25% of the elimination of rocuronium [18], with the remainder being excreted into bile unchanged or after metabolism by the liver. While its metabolism remains poorly understood, increased rocuronium requirements in patients ingesting enzyme inducing drugs indicate that rocuronium is at least partly metabolized by the inducible microsomal enzymes in the liver. Cisatracurium is degraded by Hoffman elimination, a chemical process independent of renal or hepatic function. Mivacurium is metabolized by plasma pseudocholinesterase, which explains why its plasma clearance (5 L/min in healthy adults) exceeds liver blood flow.
Simultaneous PK/PD modelling has been used to give a full description of the pharmacological properties of neuromuscular blocking drugs. Population modelling is a powerful tool to identify quantitatively which factors affect the PK/PD of various drugs. The major strength of the population approach is that useful information can be extracted even from sparse data using blood samples and pharmacological monitoring during routine efficacy studies conducted during the development of a drug. Table 11.2 shows the population PK/PD variables for non-depolarizing neuromuscular blocking drugs.
Table 11.2. Population pharmacokinetic and pharmacodynamic variables for non-depolarizing neuromuscular blocking drugs.
Author | Subgroup | Pharmacokinetics | Pharmacodynamics | ||
---|---|---|---|---|---|
Cisatracurium | Schmith [54] |
|
|
| |
Lui [55] | control group |
|
|
| |
Tran [56] |
|
|
| ||
Mivacurium | Schiere et al [19] |
|
|
| |
Rocuronium | Ploeger [51] | Caucasians |
|
|
|
Japanese |
|
| |||
Kleijn [52] |
|
|
| ||
Proost [53] |
|
|
|
WGT=bodyweight in kg; AGE=age is years; CR=creatinine clearance in mL/min; Asian=1 for asian, 0 otherwise; Sev=1 for Sevoflurane anaesthesia, 0 otherwise.
For explanation of the PK and PD variables, see the body of the text.
The model parameters shown in Table 11.2 allow computer simulations to be performed of the behaviour of neuromuscular blocking drugs following any proposed administration regime. Compartmental volumes V1 (central), V2 and V3 (peripheral) represent drug distribution volumes, where the drug is administered (central) and where it can diffuse or be transported while in the body. These may have vague associations with different tissues in the body due to various factors such as differing solubilites and other drug–tissue interactions. In any case they represent a rather extreme simplification of the complex biological processes underlying drug distribution.
Model clearance parameters such as CL (elimination), Q2 and Q3 (inter-compartmental) represent drug transport between the various compartments, with CL being a special case of drug transport from the central compartment out of the body or otherwise made inactive. Drugs with a higher CL tend to have shorter duration of action in a straightforward manner. Interestingly, they also tend to provide a more rapid onset of paralysis because these drugs allow greater initial doses, creating a stronger driving force for drug distribution to the effect compartment. The rate of drug distribution to the effect site, described as keo, also plays an essential role in determining a drug’s potential for rapid onset of effect. A slow keo limits the rate of onset of action. While a simple first-order rate constant is often sufficient to describe drug transport to the effect site, mivacurium is an exception to this, requiring modelling of an interstitial compartment for accurate prediction of PD [19]. Other PD parameters describe various characteristics of drug effect. Ce50 describes the effect-site concentration at which 50% of drug effect is achieved. Lower values for Ce50 indicate higher drug potency. Parameter λ describes the slope of the sigmoidal Emax PD model. High values of λ indicate that drug effect changes rapidly over a low range of drug concentrations, creating an ‘on–off switch’ like drug effect. Lower values of λ describe a more gradual relationship between effect-site concentration and drug effect.
To highlight the PK/PD differences between two neuromuscular blocking drugs with an intermediate duration of action, we have simulated the course of effect-site concentrations after the administration of one ED95 dose of either rocuronium or cisatracurium. We also simulated context-sensitive 50% effect decrement times as a function of the duration of infusion which maintained neuromuscular block (and effect-site concentration) constant (Fig. 11.2).
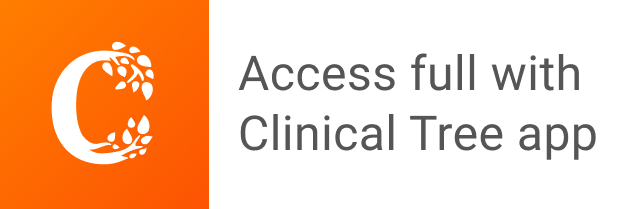