Abstract
Respiratory depression is a common and unwanted side-effect of anaesthetics, sedatives and analgesics. It has been known since the end of the 18th century that anaesthesia has pronounced effects on control of respiration, however the effects are highly specific depending on the drug and drug concentration.
Introduction
Respiratory depression is a common and unwanted side-effect of anaesthetics, sedatives and analgesics. It has been known since the end of the 18th century that anaesthesia has pronounced effects on control of respiration, however the effects are highly specific depending on the drug and drug concentration.
Respiratory depression in the perioperative context is a drug-induced depression of breathing, resulting in a reduction in minute ventilation and a subsequent rise in PaCO2 (arterial CO2 partial pressure). Often, this is detected as a low respiratory rate or as a desaturation. Notably, desaturation is a late sign of respiratory depression, especially if the patients are on supplemental oxygen (e.g. nasal cannula) so pulse oximetry, although extensively used, is not an optimal way of monitoring respiratory depression. Monitoring of respiratory frequency or carbon dioxide gives more precise measurements of respiratory depression.
A small percentage of patients undergoing surgery will need an intervention at the postoperative ward for treatment of respiratory depression [e.g. arousal, verbal or mechanical stimulation, continuous positive airway pressure (CPAP)]. It can be notoriously difficult to predict and identify which patients will be at risk for postoperative respiratory depression. Therefore, knowledge about the physiological mechanisms behind respiratory depression and the pharmacological effects of the drugs used in anaesthesia is crucial to understand the complexity of respiratory depression in the perioperative period. For each individual patient, the physiology, pharmacology but also comorbidities, effects of acute illness etc. have to be taken into account. There are groups of patients that are extra vulnerable to respiratory depression, such as patients with obstructive sleep apnoea, morbidly obese patients, patients with neuromuscular disorders, very young (neonates) and very old patients as well as very sick patients (ASA IV–V).
During anaesthesia the patient is closely monitored in the operating room by an anaesthesiologist or anaesthesia nurse in the room. Because respiratory depression and apnoea are to be expected, everyone is well prepared to manage this departure from normal physiology. The real challenge of respiratory depression starts during emergence from anaesthesia and in the early and late postoperative period, especially at the surgical ward, where the frequency and quality of monitoring for respiratory depression vary.
Overview of Control of Respiration
Control of breathing is of crucial importance for adequate oxygenation and removal of CO2. It is based on chemical and behavioural control. Regulation of breathing is tightly controlled by autonomic mechanisms. In addition, during wakefulness, we can use our neocortex to voluntarily adjust ventilation to override the autonomic drive if it would be insufficient or to enable speech, diving, singing and the response to pain. Deviation from chemical equilibrium at the cellular level will elicit a compensatory response (e.g. changes in respiratory rate and tidal volume) to restore homeostasis. Anaesthesia and sedation interact with key components of regulation of breathing, ultimately causing various degrees of respiratory depression. In order to understand this, basic knowledge about regulation of breathing is needed.
Regulation of breathing is coordinated in the brainstem. After afferent input from various sensors, the central breathing pattern may be adjusted, causing efferent signals to be transmitted to effectors such as the respiratory muscles (Fig. 10.1).
Fig. 10.1 Afferent input that interacts with control of breathing. (From Eckert DJ and Butler JE (2017) Respiratory Physiology: Understanding the Control of Ventilation. In M Kryger, T Roth and WC Dement, eds., Principles and Practice of Sleep Medicine (Sixth Edition), Elsevier, pp. 167–173.e4.)
Respiration is generated and controlled by the respiratory neurons of the medulla that receive afferent input from central chemoreceptors located in the central nervous system (CNS) and from peripheral chemoreceptors in the carotid bodies. There are also peripheral chemoreceptors in the aortic bodies, but these seem to be of less importance in humans. Afferent input also comes from peripheral stretch receptors in the lung, from receptors in muscles and tendons, and from afferent signals from pain, touch and temperature receptors.
The respiratory neurons in the medulla can be divided into the ventral and dorsal respiratory group, based on their anatomical localization. The dorsal respiratory group contains the nucleus tractus solitarius (nTS), the key cardiorespiratory integrator. The ventral respiratory group consists of four nuclei and includes the pre-Bötzinger complex that is the major respiratory pacemaker. Close to the ventral respiratory group lies the retrotrapezoid nucleus that has important connections to the dorsal respiratory group and the key respiratory control centre, the nTS. The retrotrapezoid nucleus is an important area for central chemoreception. Thus, the respiratory neurons of the respiratory centre and the central chemoreceptors are both located in the medulla, but are clearly separated entities.
Central chemoreceptors tightly control the arterial CO2 partial pressure by sensing the pH-changes in cerebrospinal fluid. Because the blood–brain barrier is impermeable to polar molecules such as hydrogen ions and bicarbonate, a decrease in blood pH cannot affect the central chemoreceptors. In contrast, CO2 readily passes the blood–brain barrier, and a rise in arterial pCO2 therefore causes a similar rise in the pCO2 of the cerebrospinal fluid (CSF) (however the pCO2 in CSF is approximately 1.3 kPa higher than the arterial). An elevation of pCO2 (CSF) increases the concentration of hydrogen ions in CSF and subsequently reduces the pH in the CSF:CO2 + H2O <-> H2CO3 <-> HCO3– + H+ (increased H+ -> decrease in pH)(1)The resulting decrease in pH stimulates the central chemoreceptor neurons by a mechanism that is still not fully understood. Approximately 70% of the ventilatory response to CO2 arises from the central chemoreceptors, and 30% from the peripheral chemoreceptors. Notably, the central chemoreceptors do not respond to hypoxia.
The response of the central chemoreceptors to changes in CSF PCO2/H+ can take up to one minute, in contrast to the rapidly responding peripheral chemoreceptors in the carotid bodies that responds to hypoxia but also to a lesser degree to, for example, hypercarbia and low pH.
During resting ventilation, the central chemoreceptors modulate breathing, and the peripheral chemoreceptors remain mostly silent (unless exposed to hypoxia). An increased activity in the peripheral chemoreceptors in the carotid bodies is transferred via the glossopharyngeal nerve to the nTS, where it modulates respiration. The efferent arc of breathing control is mediated via the phrenic nerve and respiratory muscles, and results in an increased work of breathing.
Ventilatory Responses to Hypercapnia
During normal circumstances, arterial PaCO2 controls ventilation. Therefore, the ventilatory response to hypercapnia has often been used as a measure to investigate the effect of drugs on respiration. An increase in inhaled CO2 causes a linear increase in ventilation which can be described in terms of a slope (S, l/min) and the X-axis intercept B at zero ventilation (kPa):
B is the apnoeic threshold, the PaCO2 value below which ventilation ceases. The bold line in Fig. 10.2 displays a normal ventilation curve with a slope of 15 L/min and an intercept (apnoeic threshold) at 4.8 kPa.
There is wide inter-individual variation in the PaCO2/ventilatory response. The response is linear up to approximately 10 kPa, but at higher CO2 partial pressures central respiratory neurons become depressed and the ventilation decreases. The linear PaCO2/ventilation slope is steeper during hypoxia and less steep during hyperoxia (Fig. 10.2). As shown in Fig. 10.2, respiratory depression may be caused by a shift of the PaCO2 /ventilatory response curve to the right, or a decrease in slope, or both.
Hypercapnia arouses and stimulates the respiratory centre. When patients are waking up from anaesthesia, we use this physiological process: we allow the end-tidal CO2 and thus PaCO2 to rise to stimulate the respiratory centre, thereby increasing the likelihood that the patient will start breathing spontaneously. Vice versa, hyperventilation may lower PaCO2 below the apnoeic threshold, causing the patient to cease any spontaneous breathing efforts.
Ventilatory Responses to Hypoxia
The ventilatory response to hypoxia is an immediate reaction to hypoxaemia in arterial blood that aims to restore normoxia. In a healthy adult breathing room air, desaturation and subsequent hypoxaemia occur within a few minutes if apnoea occurs and even faster in patients with comorbidities. The hypoxic ventilatory response is illustrated below (Fig. 10.3).
Fig. 10.3 The hypoxic ventilatory response. Hypoxia initially causes an acute increase in minute ventilation (the acute hypoxic ventilatory response or HVR), but this is followed after two to five minutes by a decline in ventilation (the hypoxic ventilatory decline or HVD).
Minute ventilation increases within seconds after hypoxia (the hypoxic ventilatory response). The degree of the response is dependent on whether there are isocapnic or poikilocapnic conditions. But after two to five minutes, the hypoxic ventilatory decline (HVD) sets in, caused by hypoxia-induced depression of the respiratory neurons in the brainstem. If hypoxia persists, a new balance will occur and (during isocapnia) ventilation will gradually increase again. Under normal conditions, the ventilatory response to hypoxia only contributes a small part to resting ventilation, but its contribution may become crucial in situations where the central chemoreceptors are affected, i.e. after opioid administration, in patients with chronically high pCO2 etc. – in fact, approximately 10% of the population lacks the ventilatory response to hypoxia. Patients that have undergone bilateral neck dissection and to some extent also those that underwent bilateral carotid surgery and thus had their carotid bodies removed, totally lack the ventilatory response to hypoxia. In animals this response restores with time, probably due to compensatory mechanisms in the aortic bodies, but in humans this is irreversible due to the lack of clinical effect by the aortic bodies [1].
If progressive hypoxia is applied during 15 minutes, ventilation progressively increases. This pO2/ventilation curve has the shape of a rectangular hyperbola (Fig. 10.4).
Hypoxic ventilation can also be tested with the Dejour’s test: in a healthy adult, administration of 100% oxygen for a short period will silence the chemoreceptive cells in the carotid body and transiently reduce basal ventilation. Patients that are exposed to chronic or intermittent hypoxia such as COPD or obstructive sleep apnoea (OSA) will have a disturbed response to hypoxia.
Effects of Anaesthetics, Sedatives and Analgesics on Control of Breathing
General anaesthesia impairs control of breathing. Different drug classes differently affect the various parts of control of breathing, i.e. resting ventilation and the hypercapnic and hypoxic ventilatory response.
Inhaled anaesthetics: Resting ventilation is affected by inhaled anaesthetics in a partial pressure dependent manner. At low partial pressures, there are only minimal effects on the minute volume: tidal volume will gradually decrease, but this is compensated by an increase in respiratory frequency. Importantly, anaesthesia per se can reduce metabolic demand and thereby cause a decrease in CO2 production. At higher partial pressures of the inhaled anaesthetic, the respiratory frequency slows down and the spontaneous minute ventilation can become very low. The subsequent rise in PaCO2 may partly counteract the reduction in ventilation. Since monotherapy with inhaled anaesthetics rarely causes apnoea during spontaneous ventilation up to 2MAC but does depress airway reflexes around 1.5MAC, inhaled anaesthetics have been used for ‘awake’ fibreoptic intubation in patients with compromised airways.
Inhaled anaesthetics dose-dependently attenuate the response to hypoxia; at subanaesthetic concentrations, such as those that can be seen in the postoperative period or in an awake sedated patient, the ventilatory response to hypoxia is reduced by approximately 30–50% and at anaesthetic concentrations the response is virtually abolished [2, 3, 4]. The ventilatory response to increased CO2 during subanaesthetic concentrations of inhaled anaesthetics is reduced, but to a lesser extent than the hypoxic response [3].
Propofol: Propofol is a well recognized respiratory depressant. Resting ventilation is reduced in a dose-dependent manner: with increasing concentrations, minute ventilation is reduced, mainly due to a reduced tidal volume, and a subsequent rise in PaCO2 occurs. Apnoea after induction with propofol is common. The ventilatory response to hypoxia is reduced by 50% during moderate sedation. There is a similar 50% reduction in hypercapnic ventilatory response with increased doses of propofol [5, 6, 7].
α2-agonists such as clonidine and dexmedetomidine have minimal effects on resting ventilation in both sedative and anaesthetic doses and have therefore been put forward as a sedative/anaesthetics with minimal effect on ventilation. However, when dexmedetomidine is given as a bolus dose of 1–2 μg/kg over ten minutes, a short apnoea might occur, and minute ventilation can be slightly decreased [8]. Oral clonidine causes a reduction in the ventilatory response [9] and, recently, it was demonstrated that dexmedetomidine in sedative doses reduces both hypoxic and hypercapnic ventilatory response in healthy volunteers [7].
Ketamine is an intravenous anaesthetic that causes dissociative anaesthesia, and in contrast to other intravenous anaesthetics is characterized by maintaining more stable respiration. Low doses of ketamine (< 0.5 mg/kg) stimulate the respiratory centre, causing an increase in frequency and tidal volume that results in an increase in minute ventilation [3]. Anaesthetic doses of ketamine (1–2 mg/kg) depress ventilation. The ventilatory response to increased CO2 is also reduced, with a right-shift in the CO2 response curve (i.e. a parallel shift and no change in slope). Ketamine inhibits NMDA-receptors but in addition works as a μ-opioid receptor agonist, and studies involving μ-opioid receptor knock-out mice indicate that the respiratory depressant effect of ketamine most likely is related to its effect on the μ-opioid receptor [3]. There have not been any human studies on the effect of ketamine on hypoxic ventilatory response.
Benzodiazepines and more specifically midazolam dose-dependently depress ventilation. Resting ventilation is reduced by a reduction in tidal volume. Induction of anaesthesia with midazolam can cause apnoea. Furthermore, midazolam attenuates the ventilatory response to both hypoxia and hypercarbia [3].
While non-opioid analgesics such as non-steroidal anti-inflammatory drugs (e.g. paracetamol) have minimal effect on control of breathing, opioids are the classical example of a respiratory depressant drug. The most commonly used opioids during anaesthesia are morphine, fentanyl, alfentanil, sufentanil and remifentanil. Opioids target μ-, κ- and δ-opioid receptors, but it is now clear that it is the interaction with the μ-opioid receptors at respiratory neurons in the respiratory centre of the brainstem that is responsible for opioid-induced respiratory depression. Importantly, the μ-opioid receptor antagonist naloxone can reverse opioid-induced ventilatory depression. It is however critical to consider the duration of action of the administered opioid in relation to the duration of action of naloxone. Currently, alternative approaches to avoid opioid-induced respiratory depression are being investigated, e.g. biased μ-opioid receptor ligands that selectively target a non-β-Arrestin signalling pathway associated with the G-protein of the μ-opioid receptor [10].
Synergy for Opioids, Hypnotics and Benzodiazepines
Modern anaesthesia and sedation strategies are based on so-called balanced anaesthesia, i.e. a combination of a hypnotic/sedative with an opioid and a muscle relaxant, in order to achieve desirable levels of hypnosis/sedation, analgesia and immobility without cardiovascular compromise. Importantly, drug synergy may cause profound respiratory depression. With all anaesthetics, the respiratory depressant effect is augmented (or synergistic) if an opioid is added.
If a low dose of ketamine is combined with a low dose of propofol, midazolam or opioid, the respiratory stimulant effect of ketamine will prevent the respiratory depressant action of these drugs; with higher doses of ketamine though, respiratory depression is augmented. Sedative doses of propofol in combination with low doses of opioids (e.g. remifentanil) cause synergistic depression of resting ventilation, increase resting PaCO2 and decrease the ventilatory response to CO2 [11]. It is speculated that this is due to an interaction with chemoreceptors (by propofol) and a simultaneous inhibition of behavioural control of breathing (by remifentanil) [11].
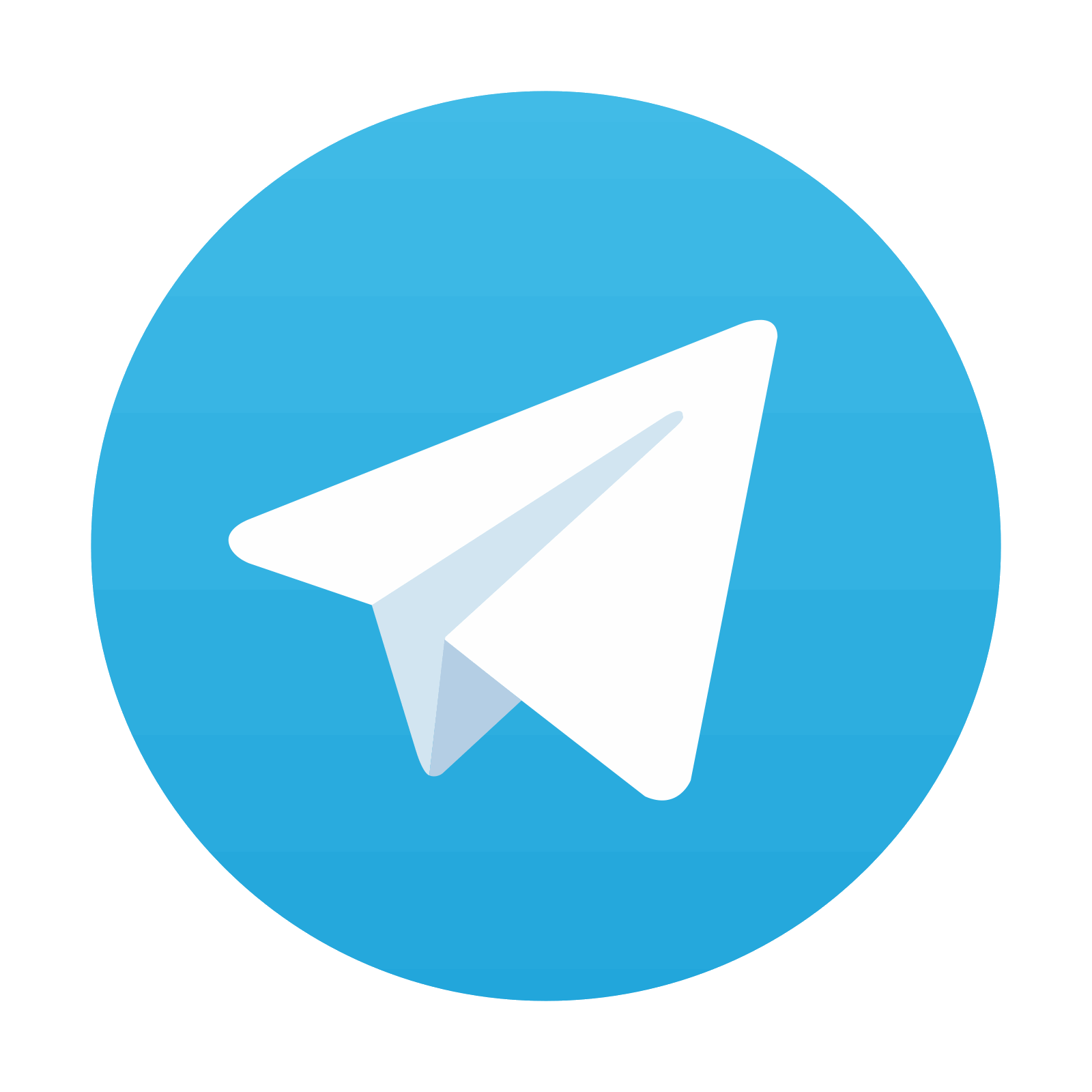
Stay updated, free articles. Join our Telegram channel
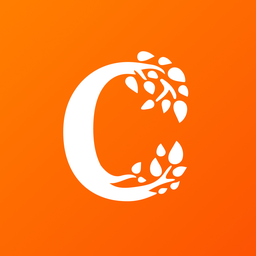
Full access? Get Clinical Tree
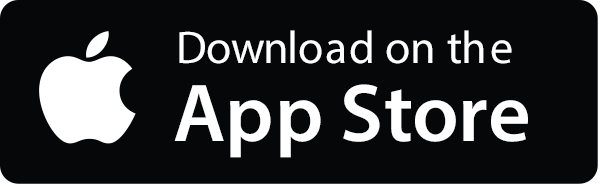
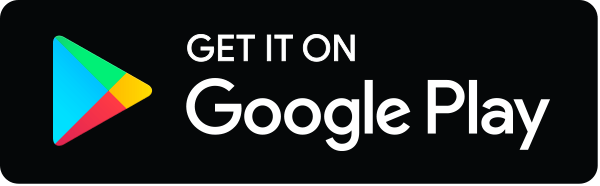
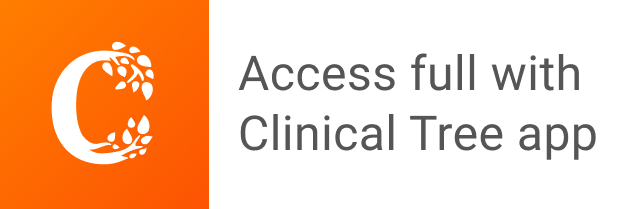