Uteroplacental Circulation and Respiratory Gas Exchange
Mark I. Zakowski
Sivam Ramanathan
Revised from the fourth edition, written by Julian T. Parer, Mark A. Rosen, and Gershon Levinson.
The placenta is a union of maternal and fetal tissues for purposes of physiologic exchange of nutrients, respiration and metabolic waste. Since many stillbirths and depressed fetuses are the result of intrauterine asphyxia, the factors governing the adequacy of placental function, particularly respiratory gas exchange, assume great importance.
Placental Anatomy and Circulation
The human placenta is a dynamic organ with vast changes from initial implantation in the uterine wall until birth. The human embryo initially implants into the uterus at the blastocyst stage. The human placenta grows initially in a low-oxygen environment, with PO2 <20 mm Hg, following the historical development of species from the pre-oxygen atmosphere epoch. Prior to 10 weeks gestation, the uterine spiral arteries are blocked by extravillous trophoblasts, with an absence of blood flow on Doppler and in vivo oxygen tension <20 mm Hg (1). The placenta and burgeoning fetus are supplied by secretions from the endometrial glands, and perhaps should be described as deciduochorial during this time period (2). The low-oxygen milieu stimulates placental angiogenesis via hypoxia inducible factor-1α and its effect on vascular endothelial growth factor and placental growth factor protects against damage and congenital abnormalities during organogenesis by reactive oxygen species (ROS). ROS are highly reactive oxygen-based molecules with an unpaired valence shell electron that damage DNA, lipids, proteins, and enzymes. The endometrial endovascular trophoblastic plugs are normally lost at 10 to 12 weeks gestation with maternal blood flow occurring peripherally, and then centrally on the placenta. The remodeling results in high volume, low-resistance maternal blood flow within the intervillous space. Improper remodeling at this secondary invasion stage is a precursor of preeclampsia. Intervillous oxygen tension rises to 40 to 80 mm Hg, with higher oxygen tension in the central part of the placental lobule and lower at the periphery of the placenta (Fig. 2-1). The placenta adapts to changes in maternal blood flow and increased oxygen tension. The placenta increases the antioxidant enzymes catalase, glutathione peroxidase, and superoxide dismutase within the placenta to deal with the increase of ROS and oxidative stress of the increased blood flow and increased PO2. Insufficient trophoblast invasion and lack of proper vascular remodeling in early pregnancy produces premature blood flow and increased oxygenation in the intervillous space, which actually reduces maternal blood flow later in pregnancy (3).
The human placenta is classically described as a villous haemomonochorial type with only one layer of syncytiotrophoblast intervening between maternal and fetal circulation. The villi are projections of fetal tissue surrounded by chorion that are exposed to circulating maternal blood. The chorion is the outermost fetal tissue layer. At term, the human placenta weighs about 500 g and is disc shaped, with a diameter of approximately 20 cm and a thickness of 3 cm. The normal fetal-to-placental-weight ratio is approximately 6:1 at term. Before this, the placenta is relatively heavier and the ratio is less (e.g., 3:1 at 30 weeks of gestation).
Circulation of blood through the placenta is illustrated in Figure 2-2. The maternal blood is carried by the uterine arteries, which divide into spiral arteries in the basal plate. Blood is spurted from these arteries into the intervillous space. It traverses upward toward the chorionic plate, passing fetal villi where exchange takes place, and finally drains back to veins in the basal plate.
The fetal circulation within the placenta is quite different. Blood is carried into the placenta by two umbilical arteries that successively divide into smaller vessels within the fetal villi. Ultimately, capillaries traverse the tips of the fetal villi where exchange occurs with maternal blood within the intervillous space. The maternal–fetal blood flow pattern and vascular geometry consist more like a cross-current exchanger than the more efficient counter-current exchange mechanism. The blood is finally collected into a single umbilical vein in the umbilical cord, and this carries the oxygen, nutrient-rich and waste-poor blood to the fetus.
Fetal and maternal blood streams are separated by three microscopic tissue layers in the human placenta. The first layer is the fetal trophoblast, which consists of cytotrophoblast and syncytiotrophoblast. The syncytiotrophoblast is the metabolically active part of the placenta, where much of the endocrine function of the placenta occurs. The other tissue layers are fetal connective tissue, which serves to support the villi, and the endothelium of fetal capillaries (Fig. 2-3). As the placenta and fetus grow, the diffusion distance decreases as the rate of fetal capillary growth in the villi exceeds the trophoblast growth; thus placental oxygen diffusive conductance is matched to fetal weight (demand), which is also true for other species (4). At term, the ratio of cytotrophoblast to syncytiotrophoblast is significantly lower than earlier in pregnancy.
The quantitative relationship of fetal and maternal blood flow and relative concentrations of substances at any one point in the human placenta are quite complex. The relative rates of blood flow in various areas of the placenta are also quite variable, and there is a continually changing concentration of nutrients and waste materials in various areas of the placenta as exchange occurs (5).
Mechanisms of Exchange
Substances are exchanged across the placental membrane by five mechanisms (Fig. 2-4): Diffusion (passive and facilitated), active transport, bulk flow, pinocytosis/phagocytosis, and barrier breaks (6). The placenta is a dynamic organ throughout pregnancy and these mechanisms of transfer vary at different stages of gestation. Placental stress states such as acidosis may cause placental cell damage, increasing placental permeability of substances (e.g., local anesthetics) (7).
Diffusion
Passive diffusion, a physicochemical process that requires no energy, occurs when substances pass from one area to another along a concentration gradient. The respiratory gases, oxygen and carbon dioxide, the fatty acids, and the smaller ions (e.g., Na+ and Cl-) are transported by this mechanism (6).
Facilitated diffusion describes the mechanism of passage of glucose and some other carbohydrates. With this mechanism, substances still pass down a concentration gradient, but the rate of passage is greater than can be explained by
the gradient alone. Possibly, carrier molecules are involved, and there may be need for energy expenditure. Facilitated diffusion does not use chemical energy in the form of ATP, but uses the gradient of another compound and a transporter. The system can be saturated at high concentrations relative to the Michaelis–Menten constant (Km).
the gradient alone. Possibly, carrier molecules are involved, and there may be need for energy expenditure. Facilitated diffusion does not use chemical energy in the form of ATP, but uses the gradient of another compound and a transporter. The system can be saturated at high concentrations relative to the Michaelis–Menten constant (Km).
Glucose crosses the placenta via facilitated-diffusion carriers inserted in both microvillus and basal membranes. In this mode of transport, the movement of glucose down its concentration gradient to the fetus is dependent on blood flow and plasma concentrations, and also on cellular energy supply. Changes in glucose transport and utilization occur in several placental pathophysiologies including intrauterine growth restriction (IUGR), diabetes mellitus, and preeclampsia.
Active Transport
Active transport moves substances in a direction against the concentration gradient. Energy is required; carrier molecules are involved; and active transport is subject to inhibition by certain metabolites. The amino acids, water-soluble vitamins, and some of the larger ions (e.g., Ca+ + and Fe+ +) are transported by this mechanism.
Primary active transport: Movement of a substance takes place against its concentration gradient (i.e., uphill) via a specific protein carrier, which uses the energy in the form of adenosine triphosphate (ATP) to drive transport.
Secondary active transport: Movement of one transport substrate down its electrochemical gradient (e.g., sodium entry into cells) via a protein carrier provides the energy for movement of a coupled transport substrate via a carrier.
Amino acids are transported by a series of transporters with multiple substrate specificities. They can be divided into two types, those co-transported with sodium (secondary active transport) and those independent of sodium. Amino acids are transported “uphill” against a concentration gradient from the maternal circulation into the fetal circulation.
Alterations in amino acid transport have been postulated in a number of placental pathophysiologies. Animal and human studies have shown clear deficits in certain classes of amino acids in IUGR. Studies on placental amino acid transporters from term and preterm human tissues have shown a greater than 5-fold increase in transport capacity between 10 and 40 weeks of gestation and decreases in amino acid transport capacity in both IUGR and macrosomic diabetes.
Most drugs that are transported are similar in structure to endogenous substrates. Active drug transporters are located in the maternal-brush border and the fetal-basal membrane; so active transporters exist in both maternal to fetal as well as fetal to maternal directions. Transporters include P-glycoprotein, of the ATP-binding cassette (ABC) transporter family, which transports a wide variety of drugs, typically uncharged or basic, between 200 and 1,800 Daltons and transports in the maternal to fetal direction. Other examples include the ABC transporter multidrug resistance protein family, breast cancer resistant protein, monoamine transporters, and novel Na+ driven organic cation transporter 2.
The importance of efflux drug transporters in protection from fetotoxic effects of chemicals has been confirmed in transporter knockout animals (8) (Fig. 2-5). Transporter proteins are inducible by foreign compounds, natural compounds, and inflammatory diseases. Many cationic drugs like diphenhydramine, clonidine, and ranitidine compete with and inhibit choline uptake in a carrier-mediated fashion (9).
Smoking alters human placenta structure and transporters. Not all drugs transfer as predicted by simple models; buprenorphine, a highly lipophilic narcotic agonist–antagonist, has a low maternal to fetal transfer in vitro. Cocaine and amphetamines may compete with the norepinephrine transporter on placental surface. Elevated levels of maternal norepinephrine may cause uterine artery vasoconstriction and may induce uterine contractions.
Bulk Flow
This describes the passage of substances resulting from a hydrostatic or osmotic gradient. Water is transported by this
mechanism and may also carry some solutes with it under the influence of this mechanism.
mechanism and may also carry some solutes with it under the influence of this mechanism.
Water diffuses rapidly across the placenta in both directions. Fetal water acquisition (bulk flow) results from the maternal/fetal movement of water in response to an osmotic gradient. An osmotic gradient of less than 1 mOsm is sufficient to drive fetal water acquisition at term. The movement of water is dependent on the solute concentration and thus pumping of NaCl which requires ATP and may therefore be sensitive to conditions that produce reductions in cellular energy supply, such as hypoxia. Water permeabilities or the forces driving water movement may be altered in polyhydramnios, oligohydramnios, nonimmune fetal hydrops, or drinking excessive free water, as has been reported during labor. Transtrophoblastic channels which are usually 150 Angstroms wide, may become dilated when umbilical venous pressure rises and allow bulk pressure movement of water and proteins (e.g., albumin) (10).
Pinocytosis
Some large molecules such as the immune globulins are transported by being enclosed in small vesicles consisting of cell membranes. Phagocytosis and pinocytosis are thought to be relatively slow and not a primary determinant in transfer of drugs (11).
The cellular uptake of proteins (by endocytosis) involves binding of the protein to a specific receptor on the cell surface followed by the pinching off of a plasma membrane vesicle, which moves in the interior of the cell. Acidification of the endocytic vesicles releases the protein and the vesicle recycles to the cell surface. Efflux, or exocytosis, reverses this process. Sequestration of the specific protein into vesicles takes place followed by transfer of the vesicle to the cell surface, fusion with the plasma membrane, and release of the protein.
Iron circulates into an iron-binding protein, transferrin. Iron-bound transferrin binds specifically to transferrin receptors on the syncytiotrophoblast microvillus surface. These receptors are internalized in a plasma membrane (endocytic) vesicle and the iron is then released to the cell, while the iron-free transferrin, still bound to its receptor, is recycled back to the microvillus surface, along with the plasma membrane fragment.
Breaks
The delicate, filmy villi may at times break off within the intervillous space, and the contents may be extruded into the maternal circulation. It is also thought that maternal intravascular contents may be taken up by the fetal circulation at times. The most important result of this is seen when fetal Rh-positive red blood cells are deposited in the vascular system of an Rh-negative mother, resulting in alloimmunization and subsequent erythroblastosis fetalis. Fetal squamous cells have been reported in the maternal circulation without causing any maternal problems. When large amounts of fetal elements enter the maternal circulation, amniotic fluid embolism may occur.
Diffusion
When limitations of placental transfer occur in the human, they usually are first recognized as limitations of those substances that are exchanged by passive diffusion. For example, an acute decrease in placental function limits exchange of oxygen to and carbon dioxide between the fetus and the
mother, resulting in fetal asphyxia. A more chronic decrease in placental function may limit the transfer of substances necessary for growth (e.g., carbohydrates), thus leading to IUGR. Hence, the process of diffusion is examined in some detail.
mother, resulting in fetal asphyxia. A more chronic decrease in placental function may limit the transfer of substances necessary for growth (e.g., carbohydrates), thus leading to IUGR. Hence, the process of diffusion is examined in some detail.
Fick’s equation describes the physicochemical process of passive diffusion:
Rate of transfer (Vdiff) = (concentration gradient × area × permeability)/membrane thickness
Each of the factors determining rate of passage of substances by diffusion is considered in turn. Note that some factors affect the steady state concentrations and while others have an effect predominantly on the immediate-, short-term transfer rates.
Concentration Gradient
The concentration gradient of a substance across the placenta is equal to the difference between the mean maternal blood concentration and the mean fetal blood concentration within each of the exchanging areas. This gradient varies throughout the placenta because of the placenta’s peculiar circulatory anatomy. It probably varies from place to place and also from time to time in any particular area. However, by considering a simplified 2-compartment model with an exchanging membrane and blood flowing in from each side, each of the factors that would affect the concentration gradient can be conceptually discussed (Fig. 2-6). These factors are
Concentration of free, unbound substance in maternal arterial blood
Concentration of free, unbound substance in fetal arterial blood
Maternal intervillous space blood flow
Fetal–placental blood flow
Note that pathologic conditions such as elevated umbilical venous pressure has been associated with reduced drug transfer.
5. Diffusing capacity of the placenta for the substance
6. Ratio of maternal to fetal blood flow in exchanging areas
This is analogous to ventilation-to-perfusion ratios as applied to the lung. Inequalities in the ratio give rise to decreased efficiency of transfer. Exchange of substances is optimal if the flows are evenly matched. The blood flow will affect the transfer of some substances. A freely diffusible substance is considered flow limited; the transfer is limited by the blood flow (e.g., carbon dioxide). A slowly transferred substance is not affected by changes in blood flow; the transfer is diffusion limited.
7. Binding of substances to molecules and dissociation rates
Depending on the rate of dissociation, this reaction time could limit the transfer of a substance. This does not appear to be limiting with regard to the dissociation of oxygen and hemoglobin. The ultimate ratio in fetal versus maternal circulation can be greatly influenced by the protein binding of the substance. Generally, albumin binds acidic, lipophilic drugs while α1-acid glycoprotein (AGP) binds basic, lipophilic drugs. Note that concentrations of these proteins change during pregnancy; the fetal:maternal albumin ratio changes from 30% in the first trimester to 120% at term. Fetal levels of AGP triple from first to third trimesters. The maternal form of albumin has a higher affinity for some drugs such as local anesthetics. Also, binding of drugs to albumin is a competitive process, between drugs as well as with free fatty acids (FFA). The maternal:fetal FFA ratio is 3:1 at term (11). High lipid solubility also can create a high uptake from the blood and potential drug depot in the placental tissue, as seen in vitro with sufentanil (12).
8. Geometry of exchanging surfaces with respect to blood flow
If the blood flows are traveling in the same direction during exchange, the system is called concurrent. If the blood flows are traveling in opposite directions, the system is called counter-current. This latter system is the most efficient from the exchange point of view. As seen in Figure 2-2, human placental intervillous blood flow is more complex—originally described as cross-current multiplier exchange, or more currently as a multivillus stream system (5). The evaluation of the mean concentration gradient of any nutrient in this system becomes extremely complex.
9. The metabolism of the substance
If a substance is consumed within the placenta, its rate of passage across the placenta will not be reflected by the concentration gradient. For example, oxygen is consumed in considerable quantities by the trophoblast and the rate of passage appears to be relatively inefficient when based on oxygen tension gradients alone. Although the human placenta contains enzymes related to drug oxidation, reduction, hydrolysis and conjugation, they are primarily geared to steroid metabolism; the fetal liver is more active in drug breakdown.
Area of the Placenta
The villous surface area of the human term placenta increases from 3.4 m2 at 28 weeks (11) to over 11 m2 at term (13). In comparison, the lung has an alveolar surface area of 70 m2. The area of actual exchange, the vasculosyncytial membrane – that is, the area where fetal capillaries approach closely
enough to the surface to exchange materials with maternal blood—is 1.8 m2.
enough to the surface to exchange materials with maternal blood—is 1.8 m2.
Placental area is decreased in a number of clinical situations. An acute decrease occurs with abruptio placentae. A minor separation of the placenta may not always lead to intrauterine fetal demise as a result of asphyxia. The ability of the fetus to survive depends on the placental reserve that existed before the onset of abruption. Some placentas, particularly those in cases of maternal hypertension or those that have infarcted fibrotic areas, have a reduced area available for exchange and, hence, lowered reserve. Thus, the placenta of a mother with long-term hypertension is likely to be smaller than expected, resulting in IUGR. The placental infarctions are thought to be caused by maternal arteriolar deficiencies leading to necrosis and fibrosis of certain villi and even entire cotyledons. Analogous to hypoxic pulmonary vasoconstriction in areas of lung V/Q mismatch, villous fibrosis may improve exchange by fibrosis of nonperfused areas. In addition, in certain cases of intrauterine infection or congenital defects, the placentas are decreased in size and area. Large placentas are found in erythroblastosis fetalis and in some diabetics. In the former case, most of the increased placental mass is thought to be hydropic in origin and, hence, is unlikely to improve the exchange characteristics of the placenta. In the latter case, it is not certain whether the increased area improves the transfer of nutrients to the fetus.
Permeability of the Placental Membrane
The permeability of a membrane to a substance depends on characteristics of both the membrane and the substance that is being exchanged. The units for permeability can be found by a transposition of Fick’s diffusion equation. There are three major determinants of permeability:
Molecular size. The term placental barrier is a relative misnomer because many substances pass through the placenta to reach the fetus. A molecular weight of 1,000 is a rough dividing line between those substances that cross the placenta by diffusion and those that are relatively impermeable by diffusion. Below a molecular weight of 1,000, the rate of passage of the molecule is related to its weight unless other properties (see below) prevent or hasten rate of passage. A common clinical example is found in cases in which it is necessary to use anticoagulants in a pregnant woman. If one uses heparin, with a molecular weight above 6,000, one does not concomitantly heparinize the fetus. However, warfarin (Coumadin), with a molecular weight of 330, will readily pass through the placenta and raise the fetal INR leading to intrapartum bleeding. Also, warfarin is a well-known teratogen in the first trimester.
Lipid solubility. A lipid-soluble substance traverses the lipid bilayers of cell membranes, including those in the placenta more rapidly than a substance that has limited lipid solubility. Most local anesthetic are weak bases with a pKa greater than 7.4. Thus, local anesthetics generally cross into nerves and the placenta in the more lipophilic, unionized form, but then equilibrate with the ionized form in the fetus. Fetal acidosis can increase transfer due to ion trapping of local anesthetics. High lipid solubility also can create a high uptake and potential drug deposition in placental tissue, as seen with sufentanil (12).
Electrical charge. This deters the passage of a substance across the placenta. For example, succinylcholine, commonly used for rapid sequence intubation, is highly ionized and is poorly diffusible across the placenta despite its molecular weight of 361. Thiopental, with a molecular weight of 264, is lipid soluble, relatively unionized, and moves very rapidly into the fetal circulation.
Substances are classified into those in which the rate of passage is either “permeability limited” or “flow limited” (14). A substance that has poor permeability is limited in its rate of passage across the placenta by permeability and not by rates of blood flow. Hence, increasing the rate of blood flow will not improve its rate of passage by much. The majority of biologic molecules are limited in their rate of passage across the placenta by resistance of diffusion. However, substances that are highly permeable are limited by the rate of blood flow. Oxygen and carbon dioxide are examples of this. Decreasing the rate of blood flow decreases the rate of exchange considerably.
Diffusion Distance
The average distance for diffusion across the placenta (13) has been measured as approximately 3.5 μm. This contrasts with the much smaller distance from alveolus to pulmonary capillary in the lung (0.5 μm). The diffusion distance decreases as the placenta matures, from 50–100 μm to less than 5 μm, meeting the increasing metabolic demands of the fetus. The distance is increased in several conditions, such as erythroblastosis fetalis and congenital syphilis. This increased distance probably is due to villous edema and presumably decreases the organ’s efficiency for exchange. Fibrous or calcified deposits in the placental vasculature, such as the ones found in diabetes mellitus or preeclampsia, presumably increase diffusion distance. Diabetic placentas have fibrin thrombi, villous edema, hyperplasia, and thickening of basement membranes (15). Insulin stimulates fetal aerobic glucose metabolism and thus oxygen demand. However, diabetic pregnancies have reduced oxygen delivery to the fetus due to higher oxygen affinity of glycosylated hemoglobin, thickening of placenta basement membrane, and reduced blood flow. Reduced oxygen stimulates angiogenesis and hypercapillarization of fetal vessels within the placenta lobules. Women with gestational diabetes mellitus (GDM) have 20% to 50% chance of developing type 2 diabetes 5 to 10 years after pregnancy and their offspring have greater incidence of diabetes and obesity (16). Even in well-controlled gestational diabetes, fetuses were significantly more hypoxic, with increased glucose and lactate at birth compared to nondiabetic controls (17).
Uterine Blood Flow
Since uterine blood flow is one of the prime determinants of passage of a number of critical substances across the placenta, its characteristics, the factors affecting it, and the effects of anesthesia on uterine blood flow are discussed in the latter portion of this chapter.
Uterine blood flow rises progressively throughout pregnancy from 50 mL/min at 10 weeks to approximately 700 mL/min at term (Fig. 2-7). This represents about 10% of the maternal cardiac output. Approximately 70% to 90% of the uterine blood flow passes through the intervillous space, and the remainder largely supplies the myometrium. About 150 mL blood is in the intervillous space at term.
The uterine vascular bed is thought to be almost maximally dilated under normal conditions, with little capacity to dilate further (18). It is not autoregulated, so flow is proportional to the mean perfusion pressure. However, it is capable of marked vasoconstriction by α-adrenergic action. The uterine blood flow is determined by the following relationship:

Many factors affect uterine blood flow. A number of causes of decreased uterine blood flow are shown in Table 2-1.
Uterine contractions decrease uterine blood flow as a result of increased uterine venous pressure brought about by increased intramural pressure of the uterus. There may also be a decrease in uterine arterial pressure with contractions. Uterine hypertonus or tachysystole (too frequent contractions) causes a decreased uterine blood flow through the same mechanism.
In sheep, if uterine arterial perfusion pressure is altered without changing the resistance of the uterine vascular bed, there is a direct relationship between uterine blood flow and the pressure (18). Hence, hypotension through any of the mechanisms noted in Table 2-1 will cause a decrease in blood flow.
In the case of maternal arterial hypertension, both chronic and acute, there is a concomitant increased vascular resistance by the uterine vascular bed, decreasing uterine blood flow. Either endogenous or exogenous vasoconstriction results in decreased blood flow because of increased uterine vascular resistance.
There are a few useful means of increasing uterine blood flow when it is known to be suboptimal. The most important clinical considerations are the avoidance or correction of factors responsible for an acute decrease in blood flow (e.g., excessive uterine activity or maternal hypotension). Uterine blood flow can generally be increased by decreasing uterine tone (if increased), by increasing maternal BP (if low), decreasing uterine vascular resistance (if increased), and by using hemodynamic alterations which increase cardiac output (see Table 2-2).
Some of the β-mimetic agents that are used as uterine relaxants for preterm labor may increase uterine blood flow, but this effect, if it occurs, is small and may only be a result of decreased uterine tonus. There are a number of experimental means of increasing uterine blood flow, sometimes transiently, but these have no real clinical use. Examples of such treatments include estrogens, acetylcholine, nitroglycerin, cyanide, ischemia, and mild hypoxia, the latter either acute or chronic (19). Antihypertensives such as hydralazine can improve uterine blood flow. Similarly, epidural anesthesia during labor will increase uterine blood flow.
Table 2-1 Factors Causing Decreased Uterine Blood Flow | |||||
---|---|---|---|---|---|
|
Clinically, it has been known for many years that maternal bed rest may improve the outcome in suspected fetal growth restriction. There is some evidence that bed rest does improve fetal growth, as evidenced by increasing estriol excretion (20).
Umbilical Blood Flow
The umbilical blood flow in the undisturbed fetus at term is about 120 mL/kg/min or 360 mL/min by noninvasive ultrasound techniques (21). Lower values are obtained immediately after birth, but are probably affected by cord manipulation during the birth process. Ultrasound can be used to calculate the peak systolic-to-diastolic (S/D) ratio, which is a reflection of vascular resistance distal to the point of measurement.
Table 2-2 Maximization of Uterine Blood Flow | |||||
---|---|---|---|---|---|
|
The umbilical blood flow in human is considerably less than that of sheep, where it is approximately 200 mL/kg/min (22). The differences may be explained by the somewhat higher metabolic rate of sheep (body temperature 39°C) and differences in hemoglobin concentrations (sheep, 10 g/dL vs. human, 15 g/dL). It is important to recognize this species difference because the bulk of our information regarding fetal circulatory physiology comes from the chronically instrumented sheep fetus. In sheep, the umbilical blood flow is approximately 45% of the combined ventricular output (22), and about 20% of this blood flow is “shunted,” that is, it does not exchange with maternal blood (5).
Umbilical blood flow is unaffected by acute moderate hypoxia but is decreased by severe hypoxia (23). Only the most proximal segment of the umbilical cord is innervated; however, the smooth muscle cells are responsive to paracrine effects and umbilical blood flow decreases with the administration of catecholamines. It is also decreased by acute cord occlusion. There are no known means of increasing umbilical flow in patients in whom it is thought to be decreased chronically. However, certain fetal heart rate patterns (i.e., variable decelerations) have been ascribed to transient umbilical cord compression in the fetus during labor. Manipulation of maternal position either to the lateral or Trendelenburg position can sometimes abolish these patterns, the implication being that cord compression has been relieved.
Blood Flow Studies in the Human Fetus
Blood Velocity Wave Forms
Real-time directed Doppler ultrasound has been used to investigate human fetal, placental, and uterine blood flows (24). Doppler ultrasound allows for measurement of velocity waveforms of red blood cells traveling in vessels. The velocity data can be used to make inferences about blood flow, vascular resistance, and myocardial contractility. Blood flow velocity waveforms have a characteristic appearance that varies from vessel to vessel. The observed waveform shape is affected by the pumping ability of the heart, the heart rate, the elasticity of the vessel wall, the outflow impedance, and the blood viscosity. Waveforms in arteries supplying low-resistance vascular beds have a characteristically high forward velocity during diastole, whereas absent or reverse diastolic flow is seen in arteries supplying high-resistance vascular beds. These observations prompted the definition of indices of flow that could be related to the vascular resistance of a downstream vascular bed. The most commonly used indices are:

where
Vmax = Point of maximal blood flow velocity/cardiac cycle
Vmin = Point of minimal blood flow velocity/cardiac cycle
Vmean = Mean blood flow velocity/cardiac cycle
Blood Flow
Doppler ultrasound permits the estimation of blood flows in the human fetus. Blood flow is calculated using the formula:

where V = Mean velocity as averaged over many cardiac cycles (cm/s)
A = Estimated cross-sectional area of the vessel (cm2)
θ = Angle between the Doppler beam and the direction of flow of the blood
This calculation is complicated by the variation in the velocity of blood cells across a vascular lumen. Cells flow faster in the center of the vessel and slower near the vessel wall. The overall flow in a vessel is the sum of the different flows across the lumen. For this reason, satisfactory volume flow measurements can best be made on large vessels (4 to 10 mm in diameter) with appropriate Doppler angles (30 to 60 degrees). The two-dimensional echo Doppler provides a means of estimating fetal cardiac output by quantifying blood flow volume at the atrioventricular valve orifices. The estimated cardiac output of the human fetus (553 mL-1 kg-1 min-1) is higher than that of the sheep (450 mL-1 kg-1 min-1). In addition, the right and left ventricular outputs are more similar in the human, as compared with the sheep. The ratio of right-to-left ventricular outputs decreases with advancing gestation, from 1.3 at 15 weeks to 1.1 at 40 weeks. In normal pregnancy, high forward velocity levels in the umbilical artery are maintained throughout diastole. A lowered diastolic flow, as seen in severe IUGR,
may reflect raised placental resistance (25). Marx et al. used Doppler ultrasound waveform analysis to demonstrate a significant reduction in umbilical artery vascular resistance (S/D ratio) with epidural analgesia in healthy laboring women, a beneficial effect (26). Youngstrom et al. (27) investigated the effect of more extensive epidural anesthesia (and maternal sympathetic blockade) on umbilical artery flow velocity waveforms in healthy, nonlaboring women undergoing elective cesarean section. They found no statistically significant change in umbilical artery resistance (S/D ratio), probably due to the lack of pain and associated release of catecholamines. Recent studies have suggested that changes in PI/RI/uterine artery notching at 24 weeks’ gestation may be predictive of preeclampsia development later in pregnancy (28).
may reflect raised placental resistance (25). Marx et al. used Doppler ultrasound waveform analysis to demonstrate a significant reduction in umbilical artery vascular resistance (S/D ratio) with epidural analgesia in healthy laboring women, a beneficial effect (26). Youngstrom et al. (27) investigated the effect of more extensive epidural anesthesia (and maternal sympathetic blockade) on umbilical artery flow velocity waveforms in healthy, nonlaboring women undergoing elective cesarean section. They found no statistically significant change in umbilical artery resistance (S/D ratio), probably due to the lack of pain and associated release of catecholamines. Recent studies have suggested that changes in PI/RI/uterine artery notching at 24 weeks’ gestation may be predictive of preeclampsia development later in pregnancy (28).
Oxygen Transfer to the Fetus
Most stillbirths and cases of fetal depression are likely the result of inadequate exchange of the respiratory gases. Oxygen has the lowest storage-to-utilization ratio of any nutrient in the fetus. From animal data, it can be calculated that a term fetus has approximately 42 mL of oxygen with a normal oxygen consumption of approximately 21 mL/min (23). In theory, the fetus has a 2-minute supply of oxygen. However, fetuses do not consume the total quantity of oxygen in their body within 2 minutes, nor do they die after this time. In fact, irreversible brain damage does not start to occur until about 10 minutes have elapsed (29). This is because the fetus has a number of important compensatory mechanisms that enable it to survive on a lesser quantity of oxygen for longer periods. Clinical situations in which there is total cessation of oxygen delivery are rare. These include sudden total abruption of the placenta or complete umbilical cord compression, generally after prolapse of the cord.
Animal experiments show that the compensations that occur in the hypoxic fetus are (a) redistribution of blood flow to vital organs, including heart, brain, and placenta; (b) decreased total oxygen consumption (e.g., with moderate hypoxia, the fetal oxygen consumption drops to 50% of the normal level); and (c) dependence of certain vascular beds on anaerobic metabolism. These compensatory mechanisms appear to be initiated with mild hypoxia and result in the maintenance of oxygen supply to vital organs during times of oxygen limitation (23).
The factors that determine oxygen transfer from mother to fetus are listed in Table 2-3. Since the transfer of oxygen to the fetus depends on rates of blood flow and not limitations to diffusion, the respective blood flow on each side of the placenta assumes major importance for maintenance of fetal oxygenation. Animal studies suggest that in the normal placenta there is a “safety factor” of approximately 50% of the total uterine blood flow. That is, the uterine blood flow will drop to half its normal value before severe fetal acidosis becomes evident (30) and oxygen uptake declines (31). This applies only to the normal situation with normal placental reserve and is unlikely to be the case in pathologic situations, such as in the infant of a hypertensive mother. In such situations, the placental function may be adequate for oxygenation but not for fetal growth, and a growth-restricted infant may result from such a pregnancy. Furthermore, with superimposition of uterine contractions on such a fetus, there may be transient inadequacy of uterine blood flow during the uterine contractions; this may be recognized by responses of the fetal heart rate (i.e., late decelerations).
During labor, uterine contractions reduce uterine blood flow and can lead to episodes of hypoxia–reperfusion stress; increased levels of lipid peroxidation were noted compared to elective cesarean section (2). The increased activity of xanthine oxidase, a marker of hypoxia–reperfusion, and decreased vitamin C levels, which typically scavenge ROS, help compensate. Preeclamptic placentas also show these changes (2). Indeed, the oxidative stress that occurs in preeclampsia leads to release of proinflammatory cytokines and angiogenic factors that affect the maternal endothelial cells. Normal placental tissue exposed to hypoxia showed similar metabolites as preeclamptic placental tissue, supporting the role of hypoxia and oxidative stress, as well as possible future diagnostic testing (32).
Table 2-3 Factors Affecting Oxygen Transfer from Mother to Fetus | ||||||||||||||
---|---|---|---|---|---|---|---|---|---|---|---|---|---|---|
|
Additional important determinants of fetal oxygenation include oxygen tension in maternal arterial and fetal arterial blood. In general, maternal arterial oxygen tension depends on adequate ventilation and pulmonary integrity. Disruptions of this function are relatively rare in obstetrics, although they can occur with pulmonary diseases such as asthma, with congestive heart failure, or in mothers with congenital cardiac defects. The oxygen affinity and oxygen capacity of maternal and fetal blood are also important determinants of fetal oxygen transfer. At a given oxygen tension, the quantity of oxygen carried by blood depends on the oxygen capacity, which depends on the hemoglobin concentration and oxygen affinity. The oxygen affinity of fetal hemoglobin (p50 at 18 mm Hg) is greater than that of maternal hemoglobin (p50 at 27 mm Hg) (Fig. 2-8). That is, the oxygen dissociation curve of the fetus is to the left of that of the mother. In addition, the hemoglobin concentration of fetal blood is approximately 15 g/100 mL in the term fetus, whereas that of the mother is approximately 12 g/100 mL. Both of these factors, an increased oxygen affinity and higher oxygen capacity, confer advantages to the fetus for oxygen uptake across the placenta (Fig. 2-9). During acidosis and increased tissue oxygen demand, fetal hemoglobin was more efficient in delivering oxygen to tissues (33).
Both the Bohr and Haldane effects enhance the exchange of oxygen and carbon dioxide across the placenta (Fig. 2-10). The Bohr effect describes the shift of the hemoglobin dissociation curve to the right by hydrogen ions, which reduces the affinity of hemoglobin for oxygen. The Haldane effect
describes the increased ability of deoxygenated blood to carry more carbon dioxide. The carbon dioxide from the fetal side diffuses into the maternal blood, causing an increase in maternal intervillous hydrogen ion, which reduces the affinity of maternal hemoglobin for oxygen, increasing oxygen transfer to the fetus. At the same time, the relative decrease in carbon dioxide on the fetal side causes the fetal blood to become slightly more alkaline, increasing the fetal hemoglobin uptake of oxygen. Since the Bohr effect occurs on both sides of oxygen delivery/uptake, it has been called the double Bohr effect. Likewise, the double Haldane effect describes maternal and fetal changes in carbon dioxide and oxygen uptake. The fetal hemoglobin becomes oxygenated and releases carbon dioxide, which has increased binding to the maternal hemoglobin that has just deoxygenated. The double Bohr effect occurs functionally by the slight opening and closing of the hemoglobin chain allowing or blocking entry of oxygen to the iron-heme–binding site. Carbon dioxide binding to the sentinel histidine on the hemoglobin chain can block access of oxygen to the heme-binding site (see Fig. 2-10).
describes the increased ability of deoxygenated blood to carry more carbon dioxide. The carbon dioxide from the fetal side diffuses into the maternal blood, causing an increase in maternal intervillous hydrogen ion, which reduces the affinity of maternal hemoglobin for oxygen, increasing oxygen transfer to the fetus. At the same time, the relative decrease in carbon dioxide on the fetal side causes the fetal blood to become slightly more alkaline, increasing the fetal hemoglobin uptake of oxygen. Since the Bohr effect occurs on both sides of oxygen delivery/uptake, it has been called the double Bohr effect. Likewise, the double Haldane effect describes maternal and fetal changes in carbon dioxide and oxygen uptake. The fetal hemoglobin becomes oxygenated and releases carbon dioxide, which has increased binding to the maternal hemoglobin that has just deoxygenated. The double Bohr effect occurs functionally by the slight opening and closing of the hemoglobin chain allowing or blocking entry of oxygen to the iron-heme–binding site. Carbon dioxide binding to the sentinel histidine on the hemoglobin chain can block access of oxygen to the heme-binding site (see Fig. 2-10).
Since most measurements have been made in the human fetus during or after labor, the values of oxygen saturation, oxygen tension, and pH are generally decreased compared with those of the mother. In fact, investigations on chronically instrumented animals have shown that the oxygen saturation and content of fetal blood and acid–base status is very close to that of maternal blood; only the Po2 is lower. Note that the quantity of oxygen delivered or taken up by each 100 mL of circulating blood in the placenta is approximately equal in the mother and fetus. A number of additional miscellaneous factors determine the rate of oxygen transfer across the placenta; they are listed in Table 2-3 as the last six determinants. They appear to be relatively minor compared with the major factors already outlined.
Carbon Dioxide and Acid–Base Balance
Carbon dioxide crosses the placenta even more readily than does oxygen; the diffusion coefficient is 20 times greater than oxygen. In general, the determinants for oxygen transfer also apply to carbon dioxide transfer across the placenta. It is limited by rate of blood flow and not by resistance to diffusion. The carbon dioxide tension in fetal blood in the undisturbed state is close to 40 mm Hg (5). It is well known that the maternal arterial carbon dioxide tension is approximately 34 mm Hg, and the mother is in a state of compensated respiratory alkalosis (lowered serum bicarbonate by the kidneys). The pH of fetal blood under undisturbed conditions is probably close to 7.4, and the bicarbonate concentration is close to that of maternal blood.
Bicarbonate and the fixed acids cross the placenta much more slowly than does carbon dioxide; that is, equilibration takes a matter of hours rather than seconds. There is a situation analogous to “respiratory acidosis” that occurs in the fetus when blood flow, either uterine or umbilical, is acutely compromised. In such cases, carbon dioxide tension acutely increases causing a drop in pH, but the metabolic acid–base status remains unchanged. This occurs during severe or profound fetal decelerations (called variable decelerations) in association with certain uterine contractions, especially during the second stage of labor. These acid–base changes are generally rapidly resolved with cessation of the contraction and
the bradycardia. However, as noted earlier, if there is a significant oxygen lack that is unrelieved, the fetus will decrease its oxygen consumption, redistribute blood flow, and depend partly on anaerobic metabolism to supply its energy needs, albeit with decreased efficiency. Under these conditions, lactate (an end product of anaerobic metabolism) is produced, resulting in metabolic acidosis. The acidosis may also be aggravated by combined respiratory acidosis because of retained carbon dioxide. Unlike carbon dioxide, lactate is lost slowly from the fetus.
the bradycardia. However, as noted earlier, if there is a significant oxygen lack that is unrelieved, the fetus will decrease its oxygen consumption, redistribute blood flow, and depend partly on anaerobic metabolism to supply its energy needs, albeit with decreased efficiency. Under these conditions, lactate (an end product of anaerobic metabolism) is produced, resulting in metabolic acidosis. The acidosis may also be aggravated by combined respiratory acidosis because of retained carbon dioxide. Unlike carbon dioxide, lactate is lost slowly from the fetus.
Lactate is transported by specific, pH-dependent carriers, while protons pass to the maternal circulation via channels, lipid diffusion, co-transport, and specific proton-pumping ATPases.
Clinical Implications
Fetal compromise results from an alteration of normal placental exchange mechanisms. With a knowledge of the components involved in exchange of nutrients and waste materials across the placenta, potential problems can be recognized and corrections made.
The most important components of placental exchange are the rates of blood flow on each side of the placenta and the area available for exchange. Uterine blood flow will decline in the presence of factors causing decreased perfusion pressure or increased uterine vascular resistance. Common clinical occurrences are hypotension, hypertension, endogenous or exogenous vasoconstriction, and severe psychological stress. The uterine vascular bed is not autoregulated and normally has little capacity to dilate further. However, hypertensive states (e.g., preeclampsia) and high catecholamine levels (e.g., stress, pain) can cause increased uterine vascular resistance. During labor, it is most likely that the rate of uterine blood flow is a common limiting factor in cases of fetal compromise because of the intermittent decline in uterine blood flow with each uterine contraction. In addition, transient or persistent umbilical cord compression may cause fetal asphyxia.
Obstetric Anesthesia and Uterine Blood Flow
Obstetric anesthesia and analgesia may directly affect uterine blood flow or may alter the response of the uteroplacental circulation to noxious stimuli and to various pharmacologic agents (Table 2-4). Uterine blood flow varies directly with the perfusion pressure (i.e., uterine arterial minus
uterine venous pressure) and inversely with uterine vascular resistance. Obstetric anesthesia may affect uterine blood flow by (a) changing the perfusion pressure, that is, altering the uterine arterial or venous pressure; or (b) changing uterine vascular resistance either directly through changes in vascular tone or indirectly by altering uterine contractions or uterine muscle tone; or (c) changing the maternal cardiac output.
uterine venous pressure) and inversely with uterine vascular resistance. Obstetric anesthesia may affect uterine blood flow by (a) changing the perfusion pressure, that is, altering the uterine arterial or venous pressure; or (b) changing uterine vascular resistance either directly through changes in vascular tone or indirectly by altering uterine contractions or uterine muscle tone; or (c) changing the maternal cardiac output.
Table 2-4 Drug Effects on Uterine/Placental Blood Flowa | |||||||||||||||||||||||||||||||||||||||||||||||||||||||||||||||||||||||||||||||||||||||||||||||||||||||||||||||||||||||||||||||||||||||||||||||||||||||||||||||||||||||||||||||||||||||||||||||||||||||||||||||||||||||||||||||||||||||||||||||||||||||||||||||||||||||||||||||||||||||||||||||||||||||
---|---|---|---|---|---|---|---|---|---|---|---|---|---|---|---|---|---|---|---|---|---|---|---|---|---|---|---|---|---|---|---|---|---|---|---|---|---|---|---|---|---|---|---|---|---|---|---|---|---|---|---|---|---|---|---|---|---|---|---|---|---|---|---|---|---|---|---|---|---|---|---|---|---|---|---|---|---|---|---|---|---|---|---|---|---|---|---|---|---|---|---|---|---|---|---|---|---|---|---|---|---|---|---|---|---|---|---|---|---|---|---|---|---|---|---|---|---|---|---|---|---|---|---|---|---|---|---|---|---|---|---|---|---|---|---|---|---|---|---|---|---|---|---|---|---|---|---|---|---|---|---|---|---|---|---|---|---|---|---|---|---|---|---|---|---|---|---|---|---|---|---|---|---|---|---|---|---|---|---|---|---|---|---|---|---|---|---|---|---|---|---|---|---|---|---|---|---|---|---|---|---|---|---|---|---|---|---|---|---|---|---|---|---|---|---|---|---|---|---|---|---|---|---|---|---|---|---|---|---|---|---|---|---|---|---|---|---|---|---|---|---|---|---|---|---|---|---|---|---|---|---|---|---|---|---|---|---|---|---|---|---|---|---|---|---|---|---|---|---|---|---|---|---|---|---|---|---|---|---|---|---|---|---|---|---|---|---|---|---|---|---|---|---|---|---|
|
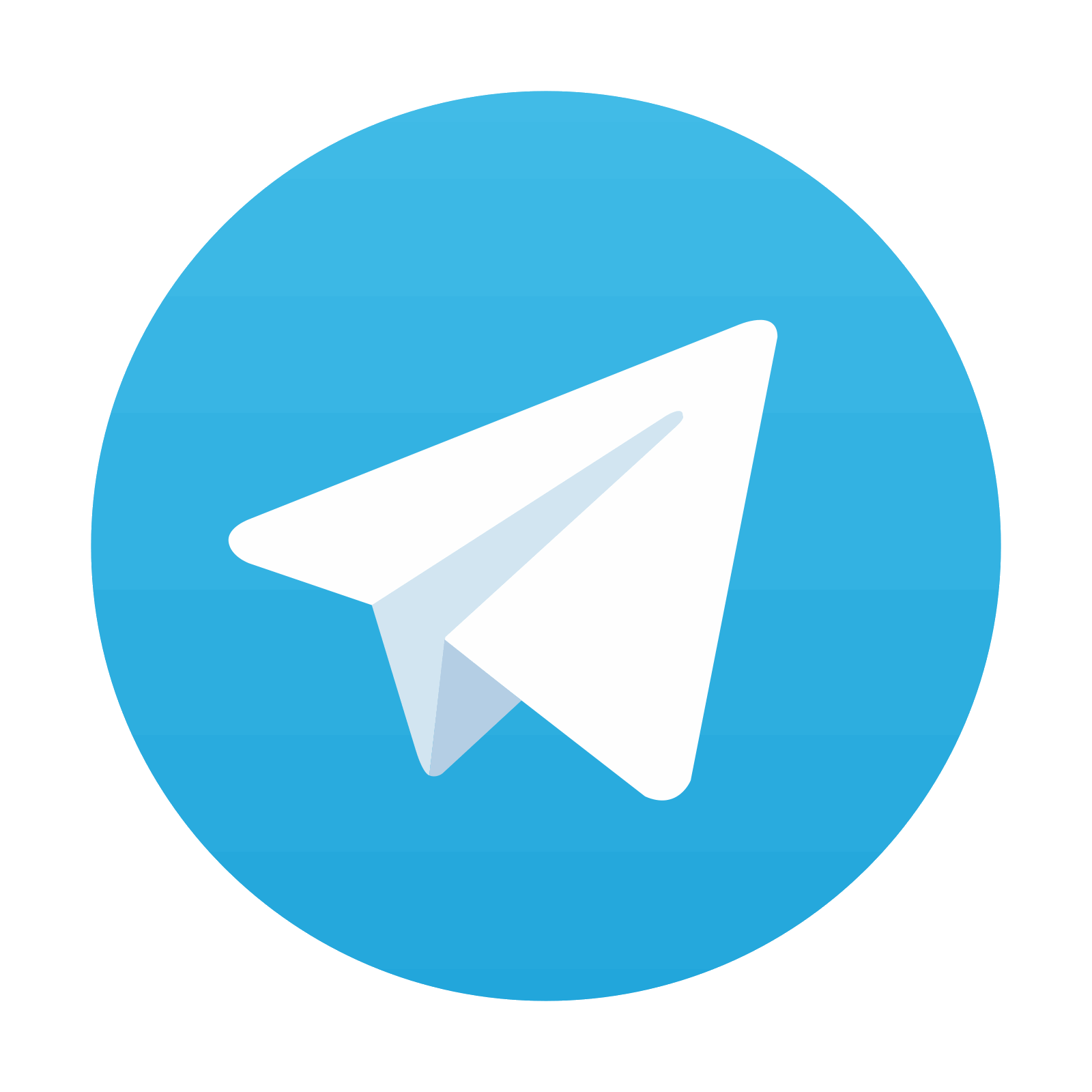
Stay updated, free articles. Join our Telegram channel
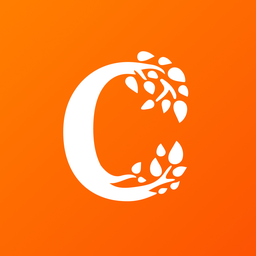
Full access? Get Clinical Tree
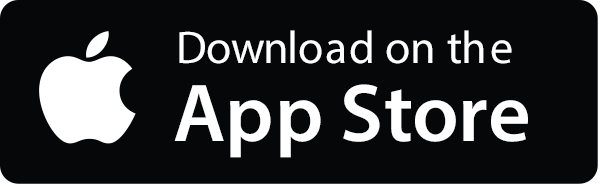
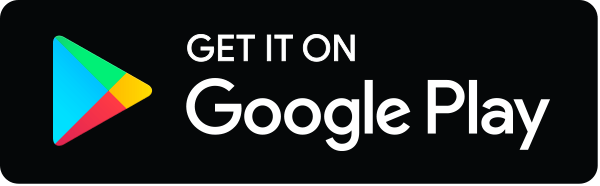