1. The Monro–Kellie hypothesis states that the sum of all intracranial contents—brain and interstitial fluid (80%), cerebrospinal fluid (CSF; 10%), blood (10%)—remains constant.
2. Maintenance of cerebral perfusion pressure (CPP) is essential to prevent cerebral ischemia.
3. A normal value for intracranial pressure (ICP) does not rule out pathology nor does a normal value indicate normal intracranial compliance.
THE CENTRAL NERVOUS SYSTEM (CNS) is a vastly complicated network that receives and processes sensory input from the body’s external and internal environments. This network consists of nerve fibers that connect the brain and spinal cord to the periphery. In addition to the nerves themselves, an intricate system of support structures aids in the maintenance of the communication pathways. These support structures involve cells such as neuroglia that provide a physical framework for the communication network. Perturbations in normal CNS development can result in devastating problems that present tremendous challenges to anesthesiologists. In order to successfully care for children undergoing neuroanesthesia, it is essential to understand the interaction between the disordered CNS and anesthetic milieu.
DEVELOPMENT OF THE CNS
BEGINNING OF THE NERVOUS SYSTEM
The neural plate is the earliest identifiable neural structure, formed during the third week from ectoderm (see Fig. 14.1). The neural plate then begins to fold in upon itself, creating the neural groove in the midline of two neural folds along each side of the groove. Toward the beginning of the fourth week, the neural folds begin to fuse dorsally to create the neural tube in a process known as neurulation (see Fig. 14.2). As the neural tube continues to fold and close, neural crest cells are separated from the closing neural folds and go on to form ganglia of spinal and cranial nerves as well as components of the peripheral and autonomic nervous systems. Fusion occurs in both cranial and caudal directions terminating when only small areas remain open at each end of the neural tube. These holes close between days 25 and 27 concomitantly with the vascular development for the neural tube. The walls of the developing neural tube subsequently thicken, resulting in the development of the brain and spinal cord while the neural canal develops into the ventricular system of the brain and the central canal of the spinal cord.
DEVELOPMENT OF THE BRAIN
The fourth pair of somites marks the delineation between tissue that develops into the brain (rostral to the fourth pair of somites) and tissue that develops into the spinal cord (caudal to the fourth pair of somites). The fusion of the cranial neural folds and closure of the rostral neuropore result in the formation of the three primary brain vesicles. These primary brain vesicles differentiate to form the forebrain (prosencephalon), midbrain (mesencephalon), and hindbrain (rhombencephalon). Two of the secondary brain vesicles, the diencephalon and telencephalon, form during the 5th week of gestation from the division of the prosencephalon. The mesencephalon does not undergo further division. The final two secondary brain vesicles, the metencephalon and the myelencephalon, are formed from the division of the rhombencephalon. Ultimately, five secondary brain vesicles exist.
FIGURE 14.1 The neural plate develops anterior to the primitive node (A), with lateral edges arising to form the neural folds and the incipient neural groove (B).
The five secondary brain vesicles subsequently undergo further division to form the mature brain structures. The telencephalon splits into the cerebral hemispheres. The diencephalon differentiates to become the thalamus and the hypothalamus. Additionally, components of the diencephalons become the mammillary bodies, pineal gland, and pituitary gland. By the middle of the fourth week, the hypophysial diverticulum (the Rathke pouch) reaches up from the oral epithelium to the ventral wall of the diencephalon. As this diverticulum pinches off at the oral epithelium, components of the adenohypophysis are developed. A pathophysiologic consequence of problems with abnormal development of this structure is the development of a craniopharyngioma.
The mesencephalon becomes the midbrain although this structure remains relatively unchanged as it develops into the adult brain. The structures formed include the superior and inferior colliculi, the tegmentum, the substantia nigra, and the cerebral peduncles. The metencephalon further develops into the pons and cerebellum, while the myelencephalon becomes the medulla.
The cavities of the secondary brain vesicles become the ventricular system in the adult brain. The lateral ventricles form from the cavities of the telencephalon. The third ventricle forms from the cavity of the diencephalon. The aqueduct derives from the cavity of the mesencephalon. The cavity of the metencephalon contributes to the upper part of the fourth ventricle, while the cavity of the myelencephalon contributes to the lower part of the fourth ventricle as well as part of the central canal.
There is a vascular area of pia mater in the roof of the fourth ventricle that differentiates to become the choroid plexus that secretes CSF. CSF absorption into the venous circulation occurs through the arachnoid villi, which arise from the epithelium of the arachnoid.
DEVELOPMENT OF THE SPINAL CORD
The mature spinal cord develops from the caudal end of the neural tube. As the caudal neural tube folds and closes, the lateral walls become thickened, reducing the size of the neural canal. The remnants of this neural canal become the central canal of the spinal cord by approximately 9 to 10 weeks of gestation. The neuroepithelial cells that form the walls of the neural tube eventually differentiate into the ependymal layer and the marginal zone. The ependymal layer gives rise to all neurons and neuroglial cells, including astrocytes and oligodendrocytes. The marginal zone gives rise to the white matter of the spinal cord as axons grow into this area from the nerve cell bodies in the cord itself.
FIGURE 14.2 A: Neural tube formation at the 7 somite stage, approximately day 22. The cranial and caudal neuropores are beginning to approximate, and (B) have formed by day 23.
FIGURE 14.3 Development of the spinal cord. The alar plate gives rise to the dorsal sensory horns and the basal plate to the ventral motor horns. The sulcus limitans defines the borders of these plates (A). This separation becomes important later as the alar and basal plates give rise to afferent and efferent functions (B).
As the lateral walls of the developing spinal cord continue to differentiate, a longitudinal groove forms on each side of the cord that is called the sulcus limitans. The sulcus limitans separates the dorsal components (alar plate) from the ventral components (basal plate) of the cord. This separation becomes important later in development as the alar and basal plates become responsible for afferent and efferent functions (see Fig. 14.3).
The mesenchyme surrounding the neural tube develops to form the primordial meninx that further differentiates to form the dura mater, pia mater, and arachnoid mater. As the meninges develop, fluid-filled spaces within the meninges consolidate to form the subarachnoid space. By the 5th week of gestation, CSF is produced.
As the caudal portion of the neural tube completes the folding and the neural canal is formed, the cranial portion of the neural tube begins to undergo rapid expansion that will eventually result in the development of the cranial ventricular system. A number of authors have speculated that the closure of the caudal neural tube is necessary for initial expansion and development of the brain and ventricular system. Once the neural tube closes and a fluid-filled cavity is established, increasing intraluminal pressure provides the stimulus for brain enlargement and development. Experimental models decompressing this tube during this period of development demonstrate reduction of brain expansion and poor development of the cells in the walls of the neural tube. These facts help explain why patients with open neural tube defects (NTDs) such as myelomeningocele may have a myriad of associated CNS defects.
PHYSIOLOGY
INTRACRANIAL CONTENTS
It is useful to think of the cranial vault as a box that has a finite amount of space within. The intracranial space is occupied by three components: (i) the brain and interstitial fluid (80%), (ii) CSF (10%), and (iii) blood (10%). Under the Monro–Kellie hypothesis, the sum of all intracranial components is constant. Therefore, an increase in the volume of one component must involve a decrease in the volume of one or both of the other components, unless the skull can expand to accommodate these increases. Infants demonstrate this exception to the rule due to the presence of unfused sutures and open fontanelles. Increases in intracranial volume that occur slowly can be compensated by unfused sutures and fontanelles (1). Additionally, increases in head circumference may be associated with the presence of space-occupying lesions and hydrocephalus. Therefore, a child whose head circumference is greater than established norms should be evaluated for intracranial pathology. Despite these compensatory mechanisms, herniation can nevertheless occur as a result of acute elevations in ICP.
INTRACRANIAL PRESSURE
Elevations in ICP can cause secondary brain injury as a result of cerebral ischemia and/or herniation. Maintenance of CPP is paramount in the prevention of ischemia. CPP is defined as the difference between mean arterial pressure (MAP) and central venous pressure (CVP or ICP if ICP is greater than CVP). Therefore, as ICP increases, CPP decreases unless there is an increase in MAP. With a decrease in CPP, cerebral blood flow (CBF) decreases. This results in decreased delivery of metabolic substrates and oxygen. Cell damage then occurs, which leads to cell death and an increase in inflammatory mediators, a cascade causing further increases in extracellular water and exacerbating the elevation in ICP. As CPP falls, neuronal dysfunction and ultimately cell death occur (1).
If elevations in ICP continue unabated, herniation can result. Several types of herniation exist, with transtentorial herniation being the most common (see Fig. 14.4). This results in displacement of the temporal lobe into the infratentorial space. Clinical signs include hemiparesis, pupillary dilation (by compression of the third cranial nerve), and loss of consciousness. If this condition is not immediately relieved, apnea and ultimately death occur. Cerebellar herniation results from translocation of the cerebellar tonsils through the foramen magnum into the cervical spine, causing obstruction of CSF flow, acute hydrocephalus, and brainstem compression. This type of herniation also ultimately results in death if not corrected immediately.
ELEVATED ICP
CLINICAL PEARL The time course of an increase in the volume of an intracranial mass or fluid collection has a great effect of intracranial compliance. A slow-growing mass may be quite large and have relatively minor effects on ICP.
Normal ICP in children is <15 mm Hg. In full-term infants, normal ICP is 2 to 6 mm Hg; in premature neonates, the value of ICP is likely less. ICP may remain normal in children with open fontanelles despite the presence of significant intracranial pathology. Pressure waves may be seen in children with intracranial pathology but normal ICP. These waves are considered abnormal (2).
Clinical signs of intracranial hypertension are variable in children. In adults, signs such as papilledema, hypertension, and bradycardia often accompany elevated ICP. However, in children, these signs may occur with normal ICP. Alternatively, they may be absent in the setting of intracranial hypertension (2,3). The classic signs of chronically elevated ICP in children consist of headaches, increased irritability, decreased oral intake in infants, and morning emesis. Children do not often develop papilledema (4). Late signs of increased ICP include alterations in the level of consciousness and abnormal responses to painful stimuli (2). Evaluation of these patients most often involves radiographic imaging, and computed tomography (CT) or magnetic resonance imaging (MRI) will often reveal a decrease in the size or complete obliteration of the lateral ventricles, hydrocephalus, midline shift, and/or obliteration of the third or fourth ventricles.
FIGURE 14.4 Various types of brain herniation. M, midbrain; U, uncus (temporal lobe); T, tonsils (of cerebellum).
Use of ventricular catheters is the most accurate method for measuring ICP, with the additional benefit of draining and/or sampling CSF. If the placement of a ventricular catheter is difficult or contraindicated, as in a patient with obliterated or slit ventricles, a subarachnoid bolt is a technically easier alternative. They may, however, underestimate true ICP, especially in areas distant from the insertion site. Additionally, subarachnoid bolts can be difficult to secure in infants with a thin calvarium. A third general type of ICP measurement system is the epidural catheter. Many of these systems do not require a fluid interface to measure pressure, relying instead on fiberoptic technology. Therefore, many of the adverse issues associated with systems utilizing fluid interfaces are avoided (5,6). Disadvantages to this technique include an inability to recalibrate the system once the catheter is inserted if a fiberoptic system is employed. Also, because these catheters are placed outside of the CSF, there is no ability to sample or drain CSF. These types of catheters are more easily secured to open fontanelles in infants (see Fig. 14.5).
CEREBROSPINAL FLUID
CSF is produced by the choroid plexus at the rate of 0.35 mL per minute or approximately 500 mL per day in adults (7). In normal adults, approximately 100 to 150 mL of CSF is present at any given time in the subarachnoid space. CSF is, therefore, replaced several times throughout the day. In children, the overall volume of CSF is smaller due to a smaller subarachnoid space. However, the rate of CSF production is similar to the rates observed in adults (7,8). Elevated ICP does not dramatically alter the rate of CSF production.
CSF is absorbed through arachnoid villi into the venous system by one-way valves between the subarachnoid space and the sagittal sinus. There is also a limited amount of reabsorption that occurs through the ependymal lining of the ventricles. As ICP increases, so does the rate of reabsorption of CSF. Despite this fact, any pathologic process that obstructs the arachnoid villi or alters normal CSF flow can lead to decreased absorption of CSF. Therefore, intracranial hemorrhage, inflammation/infection, tumors, or congenital malformations can lead to decreased CSF absorption with possible elevations in ICP (2).
INTRACRANIAL COMPLIANCE
Intracranial compliance is defined as the change in ICP relative to the intracranial volume. Clearly, if ICP is significantly elevated, compensatory mechanisms have failed. The problem is that the absolute value of ICP does not necessarily indicate the degree of available compensation. Additionally, the presence of a normal value for ICP does not rule out a pathologic state. Figure 14.6 shows an intracranial compliance curve. The morphology of the curve depends on the time over which volume increases and the relative size of the compartments. At normal intracranial volumes (point 1), ICP is low but compliance is high and remains so despite small increases in volume. As volume increases rapidly, compensatory abilities are overwhelmed and further increases in volume result in increases in pressure. This can occur when the actual ICP is still within normal limits, but the compliance is low (point 2). When ICP is already high, a tipping point is reached where further volume expansion results in rapid ICP elevation (point 3). In clinical practice, compliance can be evaluated with a variety of devices that measure ICP.
Children have a lower intracranial compliance than adults. Contributory factors include a higher ratio of brain water content, less CSF volume, and a higher ratio of brain content to intracranial capacity (1). Consequently, when similar relative increases in ICP have occurred, pediatric patients may be at an increased risk of herniation compared with adults. Infants, on the other hand, have the advantage of a higher compliance due to their open fontanelles and sutures when ICP increases occur slowly.
CEREBRAL BLOOD VOLUME AND CBF
In addition to CSF, cerebral blood volume (CBV) represents another compartment in which compensatory mechanisms influence ICP. Despite the fact that CBV occupies only a small proportion (10%) of the intracranial space, changes related to dynamic blood volume occur, often precipitated by anesthesia or other procedures. As with vascular beds in other organ systems, most intracranial blood is contained in the low-pressure, high-capacitance, venous system. Increases in intracranial volume are initially met by decreases in CBV. This compensatory mechanism can be seen in hydrocephalic infants in whom venous blood shifts from intracranial to extracranial vessels producing distended scalp veins (9).
CBF is approximately 55 mL per 100 g of brain tissue per minute in normal adults (10–12). This represents approximately 15% of the total cardiac output for an organ that accounts for 2% of total body weight. Assessing CBF in children is less uniform. CBF in healthy awake children is approximately 100 mL per 100 g of brain tissue/minute and can represent up to 25% of cardiac output (13,14). The share of cardiac output to the brain varies with age, with infants and young children having a proportionately higher percentage of cardiac output. This phenomenon represents preferential blood flow to the growing brain during critical stages of development. Neonates and premature infants appear to have a lower CBF (~40 mL/100 g/minute) than children and adults (15,16). In infants, CBF is subject to modification by sleep states and feeding (17).
Cerebral metabolic rate for oxygen (CMRO2) is one of the principal factors regulating CBF. In adults, CMRO2 is approximately 3.5 to 4.5 mL O2/100 g/minute; in children, it is higher (13). Many of the drugs utilized to induce and maintain general anesthesia can depress CMRO2 as much as 50% (18). CBF and CMRO2 are considered coupled in awake, healthy subjects. This coupling is probably mediated by the effect of hydrogen ion concentration on cerebral vessels. Conditions that result in acidosis (hypoxemia, hypercarbia, and ischemia) cause cerebrovascular dilation, augmenting CBF and CBV. When autoregulation is impaired, CBF is determined by factors other than metabolic demand. Many pharmacologic agents act directly on the cerebral vasculature to alter CBF and CBV.
CLINICAL PEARL Normal autoregulation of CBF is disrupted in preterm newborns, after traumatic or hypoxic brain injury, intracranial hemorrhage, and inflammatory processes.
CEREBROVASCULAR AUTOREGULATION
Effects of Blood Pressure
Autoregulation refers to the ability of the brain to have a relatively constant CBF within an MAP range of 50 to 150 mm Hg. Autoregulation also enables brain perfusion to remain stable across moderate changes in ICP. Normally, with low ICP and low CVP, MAP approximates CPP. Outside the range of autoregulation, CBF becomes pressure dependent. Cerebral autoregulation can be abolished by a variety of physiologic disturbances, including acidosis, tumors, cerebral edema, vascular malformations, and various drugs.
Although autoregulation occurs at lower absolute values in infants and children than in adults, the values remain undefined (19). In adult patients, the lower limit of autoregulation (MAP) is approximately 50 mm Hg. In the newborn, however, this blood pressure (BP) is not usually reached until the first few weeks of life. A useful rule of thumb for neonates is that acceptable MAP may approximate gestational age. There is animal data demonstrating intact autoregulatory mechanisms within lower BP ranges in the newborn when compared to mature animals (20). In critically ill patients, there is evidence that cerebral autoregulation may be abolished (21).
Numerous conditions may affect a child’s ability to autoregulate CBF. These include prematurity, traumatic brain injury, neurovascular anomalies, hypoxic brain injuries, intracranial hemorrhage, inflammatory processes, and a variety of congenital cardiac lesions (22). As mentioned, the limits of cerebral autoregulation are poorly defined for infants and young children. Invasive monitors are not routinely used in such patients, and this limits our knowledge of the age and disease-related limits to autoregulation in these patients (23). Neonates are particularly susceptible to hypoxic events when MAP is too low. Further, these patients are particularly sensitive to drugs that depress myocardial function. The end result is that without meticulous management of BP and ventilation to normocapnia, neonates and infants can suffer significant neurologic injury (24). We have been conditioned to worry about the neurocognitive effects of anesthetic agents by the relatively recent myriad of literature. Anesthesiologists must first ensure that adequate physiologic parameters are maintained in order to achieve the best possible outcomes in neonates and small children.
Effects of Oxygen
Extremes in oxygenation can have an effect on CBF despite the fact that CBF remains constant across a wide range of oxygenation. When the partial pressure of arterial O2 (Pao2) falls below 50 mm Hg, CBF increases exponentially in adults such that at a Pao2 of 15 mm Hg, CBF is approximately four times normal (25). The elevation in CBF results in an increase in CBV that can then cause an increase in ICP if intracranial compliance is low. It should be noted that O2 delivery is more important than actual Pao2 level. CBF can be significantly decreased by hyperoxia. Early studies by Kety and Schmidt demonstrated only a 10% decrease in CBF in adults breathing 100% O2; however, decreases of 33% have been reported in neonates (26,27).
Effects of Carbon Dioxide
There is a linear relationship between the arterial partial pressure of carbon dioxide (Paco2) and CBF. In adults, a 1-mm Hg increase in Paco2 increases CBF by approximately 2 mL/100 g/minute (26). The ability of CBF and therefore of CBV to change with Paco2 is the basis for the reduction of ICP by hyperventilation. Likewise, increases in Paco2 result in elevated CBF, although it appears that the limits at which this occurs in neonates differ from the limits in adults (28). Similarly, there is little information about the extent and duration of cerebrovascular responsiveness to hyperventilation in brain-injured and critically ill patients, particularly children. Moderate hyperventilation is frequently utilized to lower ICP acutely. There is growing evidence demonstrating the ill effects of even moderate hyperventilation in victims of head trauma. Recent reports have shown worsening cerebral ischemia in patients with compromised cerebral perfusion (29–31).
Autoregulation of CBF is impaired in areas of damaged brain (32). Blood vessels in an ischemic zone are subject to hypoxemia, hypercarbia, and acidosis, which are potent stimuli for vasodilatation. Furthermore, small localized lesions may result in impaired autoregulation in areas far removed from the site of injury (10). The extent of autoregulatory impairment is variable in brain-damaged patients. Techniques that measure global CBF may not detect localized changes. Although ICP may initially be normal, intracranial compliance is significantly reduced.
NEUROANESTHETIC PRINCIPLES AND MANAGEMENT: PREOPERATIVE EVALUATION
HISTORY
1. Because significant developmental delays or neuromuscular impairments are common in pediatric neurosurgical patients, a focused but thorough history of neural development is important. This should consist of an evaluation of the patient meeting appropriate motor milestones. Significant delays in meeting these milestones may be a sign of occult neuromuscular disease (33).
2. Many neurosurgical patients will have been completely healthy until the onset of their symptoms. The presenting symptoms should be elicited because this information can be relevant to the formulation of the anesthetic plan. For example, if the patient first presented with seizures and was then found to have a temporal lobe mass, the patient may require perioperative seizure prophylaxis. Alternatively, the patient may already be on anticonvulsant drugs that can alter normal metabolism of some commonly used agents such as muscle relaxants (34,35).
3. Space-occupying intracranial lesions can lead to hydrocephalus that can precipitate vomiting.
4. Lesions of the pituitary can lead to enuresis, whereas chronic hydrocephalus, seizures, or tumors can lead to anorexia. The presence of these diagnoses or symptomology should prompt careful evaluation of hydration status and electrolytes.
5. Diabetes insipidus or syndrome of inappropriate secretion of antidiuretic hormone (SIADH) is more common in this patient population.
6. Steroids are often utilized as first-line treatment for newly diagnosed intracranial tumors. If a patient comes to the operating room (OR) already on steroids, they should be continued and often augmented in the perioperative period.
7. Therapeutic levels of anticonvulsants should be verified preoperatively and maintained perioperatively. Patients on long-term anticonvulsants may develop toxicity, especially if seizures are difficult to control; this is frequently manifest with abnormalities in hematologic and/or hepatic function. Patients on chronic anticonvulsant therapy may also require increased amounts of sedatives, nondepolarizing muscle relaxants, and narcotics because of enhanced metabolism of these drugs (34–36).
PHYSICAL EXAMINATION
Many diagnoses that ultimately lead to neurosurgical procedures have a number of physical findings that are relevant to successful perioperative management. The preoperative physical examination should include the following:
1. An appropriate neurologic evaluation, which may involve level of consciousness, motor and sensory function, normal and pathologic reflexes, integrity of the cranial nerves, and signs and symptoms of intracranial hypertension.
2. A preoperative airway and respiratory assessment to evaluate the effects of motor weakness, impaired gag and swallowing mechanisms, and evidence of active pulmonary disease such as aspiration pneumonia that can be present in patients with impaired ability to protect their airway.
3. Noting of special circumstances for patients who have had strokes or transient ischemic attacks (TIAs). These patients may have some degree of muscle atrophy and weakness, which should be noted. This is important because upregulation of acetylcholine receptors may result in hyperkalemia following succinylcholine administration and induce resistance to nondepolarizing muscle relaxants in the affected limbs (37).
LABORATORY AND RADIOLOGIC EVALUATION
1. A baseline hematocrit is routinely obtained for all but the most minor procedures. Because of the risk of unexpected, uncontrolled bleeding, blood typing and cross matching should be performed for any major procedure.
2. The need for additional studies, such as evaluation of coagulation parameters, serum electrolyte levels and osmolality, blood urea nitrogen and creatinine values, arterial blood gas analysis, chest radiograph, or electrocardiogram, is determined by the individual considerations of the given patient and the concurrent pathology.
3. Recent liver function tests and a hematologic profile should be available for any patient who is on chronic anticonvulsant therapy.
4. Specific neuroradiological studies are usually obtained by the neurosurgeon and should also be reviewed by the anesthesiologist. A review of the radiographic images preoperatively can aid the anesthesiologist in planning for potential problems. For example, some children with a ventriculoperitoneal (VP) shunt have slit ventricles, and these patients have special risks in the perioperative period (38).
PREOPERATIVE DISCUSSIONS
Preoperative discussion should occur with the care team, patient, and family. The care team may consist of neurosurgeons, nurses, intensivists, and neurophysiologists. Each member of the team has specific concerns relating to his or her role in the care of the patient such as positioning of the patient during surgery, equipment needed, and type of anesthetic technique to be used. Communication is crucial so that everyone understands and can successfully accomplish the plan. Equally important is a frank and candid discussion with the family and when appropriate, with the patient. They should be able to confirm their understanding of the planned procedure, including the site of surgery, and be aware, when applicable, of the specific needs for invasive monitoring, blood products, postoperative care expectations, and potential complications.
INDUCTION: GENERAL CONSIDERATIONS
CLINICAL PEARL ICP can be affected by many variables, including PaCO2, PaO2, CBF, CBV, and cerebral venous drainage.
In the presence of intracranial hypertension, the primary goals during induction are to minimize severe increases in ICP and decreases in BP. In general, most intravenous (IV) drugs decrease CMRO2 and CBF, which consequently decreases ICP (39). Barbiturates are often the induction agents of choice because they do not cause pain with injection through small IV catheters. Sodium thiopental (4 to 8 mg/kg) is most frequently used. Propofol (2 to 4 mg/kg) appears to have similar cerebral properties, plus an antiemetic effect; however, its antiemetic effect is not well characterized. Etomidate, a possible neuroprotective agent, can also be used if hemodynamic stability is a concern (40–42). However, etomidate is associated with a high incidence of nausea and vomiting and may lower seizure threshold (43). Ketamine should be used with caution because of its known ability to increase cerebral metabolism, CBF, and ICP. Sudden increases in ICP have been reported following ketamine administration, especially in infants and children with hydrocephalus (44,45).
Other measures to lower ICP during induction include controlled hyperventilation, administration of fentanyl and supplemental barbiturates before laryngoscopy, and intubation and IV lidocaine (1.0 to 1.5 mg/kg), which has been shown to limit the elevation of ICP when administered several minutes before laryngoscopy (46).
Sevoflurane has essentially replaced halothane for inhaled inductions because of its more rapid onset, high patient acceptability, and general hemodynamic stability. There is a generation of anesthesiologists being trained who will never use halothane in clinical practice. Sevoflurane, like other volatile agents, does cause myocardial depression. However, sevoflurane causes less myocardial depression compared with halothane (47). Similar to isoflurane in its cerebral physiologic effects, sevoflurane with controlled ventilation blunts the increase in ICP secondary to cerebral vasodilatation from volatile anesthetic agents alone (48–50). However, sevoflurane has also been demonstrated occasionally to produce epileptiform activity, even in patients with no history of clinical seizure activity (51).
A common problem is an uncooperative toddler who has an intracranial tumor and moderately decreased intracranial compliance, yet is agitated and resistant to separation from parents. Some clinicians would argue that a crying, agitated child has demonstrated a tolerance to increased ICP and that IV induction is safer. Fortunately (for the anesthesiologist, though not for the patient), patients who have severe intracranial hypertension generally have a decreased level of consciousness and it becomes easier to insert an IV catheter in those situations when it is most necessary.
AIRWAY MANAGEMENT
It is crucial that airway management proceeds smoothly to avoid the ICP-increasing effects of laryngoscopy, hypoxemia, hypercarbia, and coughing. Narcotic administration and supplemental hypnotics before intubation minimize increases in ICP caused by laryngoscopy and intubation.
1. Nasotracheal tubes are often used for pediatric patients who will be in the prone position and whose airway will be inaccessible during the surgical procedure (such as a posterior fossa craniotomy). If a fiberoptic intubation is necessary, a nasal route is often technically easier. Contraindications to nasal intubation include choanal stenosis, basilar skull fracture, transsphenoidal procedures, and risk for sinusitis.
2. The nasal mucosa is highly vascular. Consequently, if nasotracheal intubation is planned, it is advantageous to prepare the nares with topical vasoconstrictors to minimize the risk of bleeding. Concentrated topical vasoconstrictors should not be sprayed directly into the nose because they are rapidly and effectively absorbed and can produce hypertension or even death (52). Placing a few drops of 0.25% phenylephrine (neosynephrine) on cotton-tipped applicators and positioning them in the nares, against the nasal mucosa, allows a controlled application and has the added benefit of helping gauge the patency of the nasal passage. In small children, this should be performed once anesthesia has been induced. Whichever route is chosen for intubation, it is important to secure the endotracheal tube with liberal amounts of tincture of benzoin and waterproof tape; waterproof adhesive dressings can also protect the tape from surgical preparation solutions.
3. If an oral endotracheal tube is used, it should be placed on the side of the mouth that will be upward (generally the same side as the craniotomy) so that draining oral secretions will not loosen the tape.
MUSCLE RELAXANTS
1. Succinylcholine is often used for rapid sequence intubation when prompt securing of the airway is necessary.
a. Although succinylcholine can cause an elevation in ICP, this does not appear to be clinically significant (53) and can be attenuated by pretreatment with a nondepolarizing muscle relaxant (54). Succinylcholine is contraindicated in those situations when life-threatening hyperkalemia may result from an upregulation of acetylcholine receptors following denervation injuries, including severe head trauma, crush injury, burns, spinal cord dysfunction, encephalitis, multiple sclerosis, muscular dystrophies, stroke, or tetanus (55).
2. Nondepolarizing muscle relaxants such as rocuronium, pancuronium, cisatracurium, or vecuronium may be used when succinylcholine is contraindicated, but all have a slower onset than succinylcholine.
a. When rocuronium is administered in sufficient doses (1.2 mg/kg), the onset of action is hastened and approaches that of succinylcholine (56).
b. If the neurosurgeon plans direct nerve stimulation (for seizure surgery or spinal cord detethering), muscle relaxants should be allowed to dissipate after induction and the return of muscle function verified before stimulation.
c. The dose of most nondepolarizing muscle relaxants necessary to maintain paralysis is increased in patients receiving chronic anticonvulsant medications, although cisatracurium has not shown this tendency, probably because of its unique metabolism by Hoffman elimination (57).
POSITIONING
1. Children with raised ICP should be transported to the preoperative holding area and OR with the head elevated in the midline position to maximize cerebral venous drainage. Once a patient is in the OR, the neurosurgeons and anesthesiologists must all have adequate access to the patient. This can be particularly challenging in infants and small children, for whom slight displacement of the head or small movements of the endotracheal tube can result in tracheal extubation or endobronchial intubation; so extra care must be taken to ensure the airway is secured after final positioning.
2. During prolonged procedures, it is important for the anesthesiologist to be able to visually inspect the endotracheal tube and circuit connections and to have access to suction the endotracheal tube when necessary.
3. It is also important to position the head to avoid soft tissue and ischemic nerve damage. Generally, this is not a problem because older patients are placed in pins in a Mayfield head holder. However, neonates and small infants have a thin calvarium; so head pinning systems are often avoided in these patients. Instead, there are a variety of non pin–based headrests available for these patients. Adequate padding should be used in such situations.
4. Extreme head flexion can cause brain stem compression in patients with posterior fossa pathology, such as a mass lesion or Arnold–Chiari malformation. Extreme flexion can also cause high cervical spinal cord ischemia, endotracheal tube obstruction by kinking, and displacement of the endotracheal tube to the carina or the right mainstem bronchus (58).
5. Extreme extension may be hazardous for patients with unstable cervical spines, including trauma victims and children with Down syndrome.
6. Intravascular catheters should ideally be accessible for visual inspection as they may infiltrate, causing local soft tissue damage and extravascular administration of fluids, blood products, and medications. A failure of the IV catheter to properly infuse may be even more devastating if the anesthetic technique of choice is a total IV anesthetic.
7. The patient’s eyes should be closed and covered with a waterproof dressing to prevent corneal abrasion.
8. Additional protective padding such as gauze pads or foam can be placed over the face, eyes, and extremities as needed.
9. It is important to avoid placing the patient in a position where stretching of peripheral nerves may occur.
10. Prevention of skin and soft tissue pressure injury due to direct contact with surgical accessories such as instrument stands and grounding wires is imperative.
11. It is also important to ensure that any extremities that are not directly visible to the anesthesiologist (such as the arm on the opposite side of the OR table) are secured and cannot fall off the table during the procedure, even if the position of the table is changed during surgery.
12. In older children and adolescents undergoing prolonged procedures, deep vein thrombosis prophylaxis should be considered using compression or pneumatic stockings.
Prone Position
The prone position is commonly used for accessing the posterior fossa and spinal cord.
1. The torso is usually supported by chest rolls and, often, pelvic support. These rolls are placed laterally on each side of the patient’s chest running from the shoulders toward the pelvis. A separate silicone roll or rolled blanket under the pelvis may occasionally be necessary in larger children. It is important to ensure free abdominal wall motion because increased intra-abdominal pressure may impair ventilation, cause vena cava compression, cause VP shunt malfunction, and increase epidural venous pressure and bleeding.
2. Depending on the requirements of the procedure, the head can be positioned in a variety of ways. It may simply be rotated and supported by padding, with care taken to avoid direct pressure on the eyes and nose and to keep the pinna of the ears flat. For small children, head frames that do not require pin fixation are often utilized when the cranium is too thin for pins. When these types of systems are utilized, it is necessary to ensure that there is no direct pressure on the eyes. Most surgeons prefer head pins and a head frame for older patients.
3. During posterior fossa surgery, the head is often placed in some degree of flexion. Therefore, it is necessary to ensure that the endotracheal tube is properly positioned and does not result in a mainstem intubation during positioning for surgery. This can be ensured before positioning and pin placement by flexing the patient’s head onto the chest while the patient is still in the supine position.
4. Care should be taken that the upper nares are free from pressure caused by an upturned nasotracheal or nasogastric tube, and the tape used to hold other tubes (gastric, esophageal) should not adhere to the endotracheal tube tape so that accidental dislodgment of these other devices does not result in tracheal extubation.
5. One should always have an emergency plan to turn the patient to the supine position should this suddenly become necessary; therefore, the patient’s bed should always be readily available (57).
6. Significant dependent edema may develop in a patient who is in the prone position for a lengthy period of time. This can cause problems during emergence as well as postoperatively when the airway itself also becomes edematous. The tongue, especially, can swell to enormous proportions when lingual venous drainage is inhibited. Oral airways are best avoided for this reason because they can also result in significant lingual edema from pressure on the base of the tongue. Instead, a folded piece of gauze can be placed between the patient’s teeth to prevent tongue extrusion. Rarely, patients may remain intubated postoperatively due to significant swelling. A bellwether for this event occurs when no air leak can be heard around an endotracheal tube when the cuff is deflated.
7. Recently, postoperative vision loss has been linked with the prone position. Ischemic optic neuropathy appeared to be the diagnosis for this complication and occurred in cases with significantly longer anesthetic duration, blood loss, and fluid administration (59–61). Therefore, avoidance of direct pressure on the globe and maintenance of stable hemodynamics should be assured in prone patients.
Sitting Position
The sitting position is sometimes used to provide optimal surgical exposure to the posterior fossa or other posterior craniotomies.
1. The sitting position has become uncommon in pediatric neurosurgical procedures and is rarely used in children younger than 3 years of age. However, this position may be used for patients who cannot tolerate being placed in the prone position due to excessive intrathoracic and abdominal pressure.
2. Precautions to prevent hypotension and air embolism must be meticulously followed.
3. The lower extremities are wrapped in elastic bandages, and the patient positioned gradually, with continuous monitoring.
4. Care must be taken in positioning the head. Extreme flexion may result in kinking of the endotracheal tube, advancing it into a bronchial position, or compressing the chin on the chest (which can block venous and lymphatic drainage of the tongue). Extreme flexion can also result in brain stem or cervical spinal cord ischemia, or both.
5. As in the prone position, nasotracheal tubes are often inserted because they are more secure.
6. The patient’s upper extremities are supported and padded in the patient’s lap.
7. All pressure points and extremities must be well padded to prevent peripheral nerve (especially sciatic, brachial plexus, and peroneal) damage and to avoid pressure sores.
8. Ideally, an electronic bed is utilized to allow the anesthesiologist easy manipulation of the head position and bed, unencumbered by wires and drapes.
LOCAL ANESTHESIA
CLINICAL PEARL The doses of local anesthetic and epinephrine used by the neurosurgeon must be carefully calculated since absorption from the vascular scalp is quite rapid.
It is useful for the neurosurgeon to infiltrate a combination of local anesthetic and dilute epinephrine into the proposed incision site before incision. This not only aids the anesthesiologist by providing additional analgesia, but also the epinephrine contained within the solution will reduce cutaneous blood loss.
1. If 0.25% bupivacaine with 1:200,000 epinephrine is utilized, a dose of 0.5 mL per kg is safe to administer. This will deliver 1.25 mg per kg of bupivacaine and 2.5 µg per kg of epinephrine, both well within the safe range, even if some of the drug is accidentally administered intravenously.
2. When greater volumes are required, the solution can be diluted with an equal volume of normal saline. This is still effective for both vasoconstriction and a prolonged sensory block in the postoperative period.
3. Skull nerve blockade has been demonstrated to attenuate the hemodynamic response to head pinning in adult patients (62).
4. In small children, more specific blocks of supraorbital and supratrochlear nerves can provide analgesia from the frontal area to the midcoronal portion of the occiput (63). Blockade of the greater occipital nerve will provide analgesia from the posterior to the midcoronal occiput (59,61).
MAINTENANCE
All inhaled anesthetics increase CBF. Further, the volatile anesthetics also uncouple CBF and CMRO2. Therefore, the normal relationship between CBF and CMRO2 is disturbed such that CBF increases while CMRO2 decreases.
1. Lower doses of isoflurane, sevoflurane, or desflurane, combined with hyperventilation, cause minimal changes in CBF and ICP (48,49,64). Isoflurane is often used as part of a maintenance regimen during neuroanesthesia. At two times the minimum alveolar concentration (MAC), it can induce a level of anesthesia associated with an isoelectric electroencephalogram (EEG) while, unlike several other volatile agents, still maintaining hemodynamic stability.
a. It should be noted that at this concentration, isoflurane will cause elevated CBF and consequently elevated ICP.
b. When induction is completed and the airway controlled, isoflurane is most commonly used for maintenance because it is relatively inexpensive, especially when used with low-flow anesthesia. It can be discontinued during surgical closure, permitting early awakening and neurologic examination.
c. All halogenated agents cause dose-dependent myocardial depression, some degree of peripheral vasodilatation, and systemic hypotension. These effects make their use in the sitting position more challenging.
2. The routine use of nitrous oxide during intracranial neurosurgical procedures remains a source of controversy.
a. Because patients who had undergone neurosurgery are already at a relatively elevated risk of postoperative nausea and vomiting (PONV), it would be advantageous not to add agents to the anesthetic regimen that increase the incidence of PONV further. Nitrous oxide may increase the incidence of PONV (65), or it may not (66).
b. Another potential problem with nitrous oxide is that it can increase CBF in a dose-dependent manner through cerebral vasodilatation, (67,68) leading to an increase in ICP (69). Controlled ventilation may be able to attenuate this problem.
c. Nitrous oxide, especially in high concentrations, can adversely affect somatosensory evoked potentials. This can impact intraoperative monitoring and decision making when such monitoring techniques are utilized (i.e., spine surgery) (70–72).
d. Finally, there is some animal data demonstrating that nitrous oxide can counteract the protective effects of thiopental in a model of cerebral ischemia (73).
e. Proponents of the use of nitrous oxide for intracranial procedures cite its long-track record of safety.
f. Nitrous oxide is clearly contraindicated, however, if the patient has undergone a recent craniotomy because air can remain in the head for up to 3 to 4 weeks following previous intracranial surgery (74). In this situation, high-dose opioids combined with an anxiolytic (benzodiazepine) or a higher-dose volatile agent in oxygen may be used initially, and nitrous oxide may be added once the dura is opened to avoid the risk of a tension pneumocephalus.
g. Despite the various concerns over its use, there are no outcome studies in humans showing a difference between using and not using nitrous oxide.
3. Many practitioners employ narcotic-based anesthetics during neurosurgical procedures. The reasons for this include the minimal effect narcotics have on cerebral hemodynamics as well as their relative overall hemodynamic stability.
a. The choice of agent is only important insofar as the anesthesiologist understands the relevant pharmacokinetics and pharmacodynamics.
b. Fentanyl is often utilized because it is easily titrated with minimal side effects.
c. A common loading dose is 10 µg per kg, although it is best to give this amount in divided doses (part at induction and part before head fixation or incision) because of the delay generally associated with neurosurgical preparation.
d. A dose of 2 to 3 µg/kg/hour is then usually adequate for maintenance, although some children, particularly those receiving chronic anticonvulsants, may require more (>3 µg/kg/hour).
e. An ultrashort-acting narcotic such as remifentanil may also be used.
f. Short-acting narcotics such as remifentanil offer no long-term postoperative analgesia.
i. Therefore, hypertension due to the onset of pain can rapidly develop when these infusions are discontinued unless other longer-acting narcotics have been administered in advance (75).
Several investigators have demonstrated that commonly used anesthesia drugs result in accelerated neurodegeneration in the immature CNS in a variety of species of rodents and rhesus monkeys (76–78). The data generated in animal models in the laboratory have provoked a heated debate on its relevance to the practice of pediatric anesthesia (79–81), which has been extended to the popular press (82). There is no doubt that this phenomenon is valid in the experimental paradigms used in these laboratory investigations. However, there are a number of factors that make extrapolation of these findings to humans in the daily practice of clinical pediatric anesthesiology questionable.
1. Most, if not all, of the animal and in vitro studies on this issue have significant limitations with regard to experimental model, agent dosage or concentration, duration of exposure (both absolute and in comparison to human), species, and developmental age and stage.
2. There are no defined clinical markers or syndromes associated with exposure to general anesthetics as a neonate. In contrast to general anesthetic agents, exposures to alcohol or anticonvulsant drugs during gestation have clearly characterized syndromes.
3. Furthermore, although the ability to induce accelerated neurodegeneration has been verified by several investigational groups, discrepancies in neurocognitive outcomes exist (77,83).
4. Finally, most neonatal and infant surgery is urgent in nature, and anesthesia care is essential to proceed safely.
5. It has been clearly demonstrated that inadequate anesthesia leads to poor postoperative outcomes in infants (84). It is crucial to attenuate the stress response associated with surgical stimulation.
6. Therefore, the clinician should be aware of the rapid developments in the area on anesthetic-induced neurodegeneration, but keep in context the “required” nature of virtually all infant-related surgical procedures and the more significant effect of hypoxic and cardiovascular mechanisms of brain injury and death during anesthesia and surgery in these patients.
BLOOD AND FLUID MANAGEMENT
CLINICAL PEARL Normal saline, with an osmolality of 308 mOsm/L is the preferred solution for neurosurgical procedures. Infusion of large volumes of NS can lead to hyperchloremic acidosis, however.
Meticulous management of fluids and blood products to minimize cerebral edema is a cornerstone of pediatric neuroanesthesia. In any pediatric surgical patient, it is possible to accidentally administer an excessive amount of IV fluid. In pediatric neurosurgical patients, the detrimental effects of such a mistake are magnified. In addition to other systemic complications of excessive IV fluids, the exacerbation of cerebral edema could be a devastating consequence. Blood loss can be extremely difficult to assess during neurosurgical procedures.
1. There is a limited ability of the anesthesiologist to visualize the operative field.
2. Additionally, a large amount of blood can be “hidden” in the drapes and collecting systems used during intracranial procedures. An overhead camera that provides a view of the operative field can be extremely useful in such situations.
3. A large percentage of the surgical blood loss occurs during incision and exposure due to the vascularity of the scalp and cranium. Although bleeding from bone is difficult to control, blood loss from the scalp can be decreased by the subcutaneous infiltration of dilute local anesthetic and epinephrine solutions before surgical incision. There have been some encouraging reports of the antifibrinolytic agent, tranexamic acid, being used to ameliorate blood loss in certain patient populations such as children undergoing major orthopedic and craniofacial procedures. (85,86) This drug may have some use in particularly bloody neurosurgical procedures as well including hemispherectomies although its use in these populations has not been clinically demonstrated at this time.
4. Anesthetic technique is an integral part of the creation of an optimal surgical field. Disruption of the blood–brain barrier by underlying pathologic processes, trauma, or surgery predisposes neurosurgical patients to cerebral edema that may be exacerbated by excessive administration of IV fluids. The choice and management of IV fluid may affect (i) cerebral perfusion, (ii) cerebral edema, (iii) water and sodium homeostasis, and (iv) serum glucose concentration.
5. Controversy remains over the optimal fluid choice—crystalloid versus colloid—for neurosurgical patients.
a. Most investigators believe that osmotic pressure gradients are more important than oncotic pressure gradients when trying to avoid cerebral edema. Therefore, unless indicated by a specific situation, crystalloid solutions are the main IV solutions administered.
b. Following the axiom of water moving toward the compartment with higher osmolality, a solution that is isotonic or hypertonic will not lead to cerebral swelling. However, solutions such as lactated Ringer’s, which has an osmolality of 273 mOsm per L (normal serum osmolality being 285 to 290 mOsm/L), may contribute to cerebral edema.
c. In contrast, normal saline is slightly hypertonic (308 mOsm/L) and is generally the fluid of choice for neurosurgical procedures. However, rapid infusion of large volumes of normal saline has been associated with hyperchloremic acidosis (87).
6. Inducing dehydration with osmotic and loop diuretics is a useful strategy when there is concern about cerebral edema that is compromising surgical exposure or is contributing to a dangerous elevation of ICP. However, hypotension and rebound effects may be associated with their use. Rapid administration of hypertonic solutions can cause profound but transient hypotension due to peripheral vasodilation (88).
7. Glucose-containing solutions are generally unnecessary during neurosurgical procedures and are probably best avoided except in situations in which hypoglycemia is a concern, such as in diabetic patients, patients receiving hyperalimentation, premature infants and newborns, and malnourished or debilitated children. In such situations, it is important to administer glucose solutions at or slightly below maintenance rates (by constant infusion pump) and to monitor serum glucose periodically throughout surgery to avoid extreme glucose fluctuations. Blood glucose levels are usually well maintained even in small children in the absence of IV glucose administration during typical (balanced) neurosurgical anesthetics.
8. The potential association of cerebral infarct size with hyperglycemia (blood glucose values in excess of 250 mg/dL) during ischemia is of particular concern (89).
TEMPERATURE CONTROL
Neonates and small children are at particular risk for hypothermia during general anesthesia. This fact is highlighted during neurosurgical procedures because the head accounts for a large proportion of an infant’s body surface area. Therefore, special attention should be focused on maintaining normal temperature from the time the patient is brought into the OR. Ambient room temperature should be maintained relatively high during positioning, preparation, and draping in order to minimize heat loss during this period.
1. Radiant warming lights and warming blankets are helpful, particularly in infants and small children.
2. Heated or passive humidifiers can be included in the airway circuit and will assist in maintenance of body temperature as well.
3. Forced hot air warming mattresses are the most effective means of maintaining body temperature (90).
VENOUS AIR EMBOLISM
CLINICAL PEARL Venous air embolism (VAE) is a real possibility in neurosurgical procedures. The risk of VAE is related to the pressure difference between the surgical site and the right atrium. The danger associated with a VAE is even greater if the child has any connection between the right and left sides of the heart.
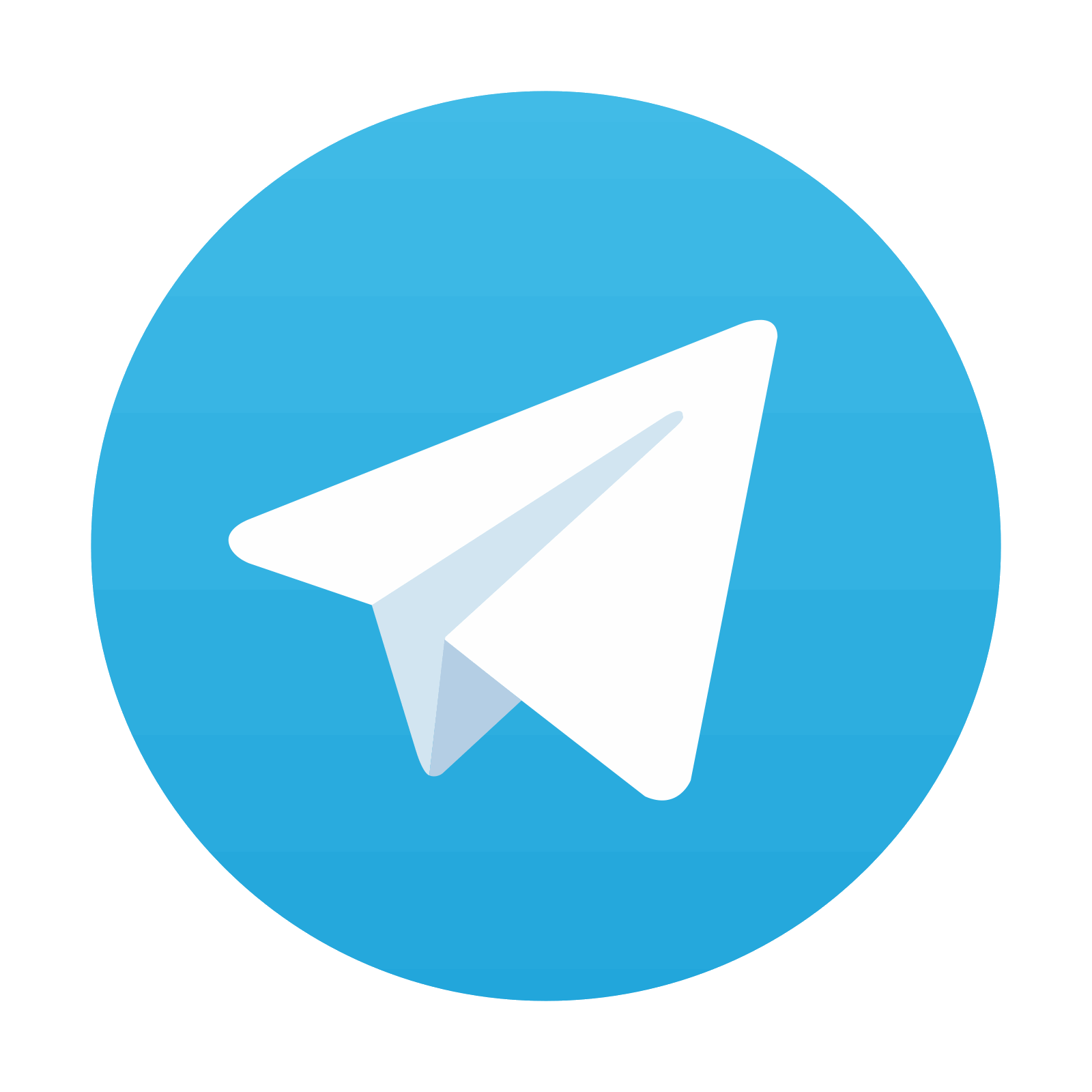
Stay updated, free articles. Join our Telegram channel
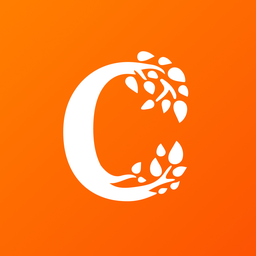
Full access? Get Clinical Tree
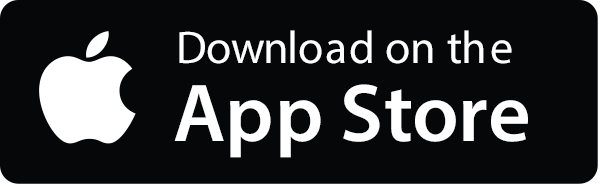
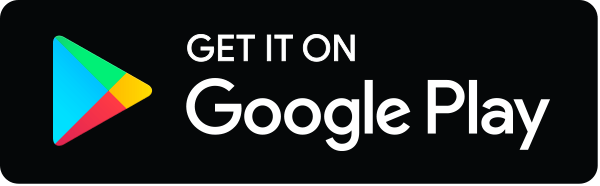