1. Much of our understanding of cardiac development is derived from research in nonhuman species with the expectation that the same processes occur in humans.
2. The etiology of congenital heart disease (CHD) is multifactorial and probably due to the interactions between several genes and modulating environmental factors, including known teratogens, infectious agents, or maternal disease.
3. Embryonic cells that contribute to cardiovascular development arise from cardiogenic mesoderm, neural crest, and the proepicardium.
4. The heart is the first organ to function, with contractions of the primitive heart tube starting around day 22 and circulation through the embryo beginning around day 26.
5. It is currently thought that the primitive heart tube gives rise mainly to the left ventricle and that precursor cells (from the secondary heart field) for the other cardiac components are added to the venous and arterial poles during looping.
6. Cardiac looping is the first indication of left–right asymmetry in the developing vertebrate, is established during gastrulation by cilia of the primitive node, and is essential for bringing the tubular configuration into the correct conformation for chamber specification, septation, and formation of systemic and pulmonary pathways.
7. The neonatal heart is characterized by reduced contractile capacity, reduced ventricular compliance, limited preload reserve, and afterload mismatch.
8. Anesthesia and operative interventions can have a significant effect on the direction and magnitude of shunting, with the pulmonary vascular resistance (PVR) being an important determinant of shunt magnitude and direction with certain defects.
9. Hematocrit is elevated in direct relation to the degree of hypoxemia with cyanotic heart disease.
10. Myocardial ischemia in the absence of coronary artery lesions can occur in neonates when low aortic diastolic blood pressure (20 to 25 mm Hg) exists in conjunction with tachycardia (heart rate [HR] >160 to 170 bpm).
11. The pediatric cardiologist is usually the best source for details of the patient’s current clinical status and previous interventions and complications.
DEVELOPMENT OF THE HEART AND VASCULAR SYSTEM
Cardiovascular development follows the same general pattern in all vertebrates and is exceptionally vulnerable to malformations (1). Much of our understanding of cardiac development is derived from research in nonhuman species with the expectation that the same processes occur in humans. Progress in genomics, proteomics, transgenesis, imaging, and integrative systems biology over the past two decades has provided new insight into the understanding of normal and abnormal cardiovascular development (2).
Although a descriptive segmental anatomic approach is used to describe cardiac embryology, cardiovascular development is a three-dimensional spatiotemporal process with many elements occurring simultaneously. An anatomic approach provides a foundation of how congenital heart defects arise when development goes wrong, and an understanding of this can inform anesthesia decisions.
The cardiovascular system is the first organ system to develop and function, beginning during the 3rd week of life when diffusion from the uteroplacental circulation is inadequate to meet the nutritional requirements of the embryo. Embryonic cells that contribute to cardiovascular development arise from cardiogenic mesoderm, cardiac neural crest, and the proepicardium (Fig. 18.1).
HEART FIELDS
Cardiac progenitor cells are located in the epiblast just lateral to the primitive streak (Fig. 18.2). After gastrulation, these cells are mesodermal and migrate laterally and cranially to the lateral plate mesoderm. The pericardial coelom splits the lateral plate mesoderm into splanchnic (ventral) and somatic (dorsal) layers. Cells in the somatic layer give rise to the pericardium. Cells that will form the myocardium are located in the splanchnic layer and constitute the heart or cardiogenic fields (3) (Fig. 18.3A,B). The heart fields unite in the midline cranial to the stomatopharyngeal (buccopharyngeal) membrane to form the cardiac crescent. Within the heart fields are at least two distinct lineages of cells that segregate and differentiate at different times (4,5). One population is referred to as the first heart field (FHF) and the other as the second heart field (SHF). The SHF is contiguous with the FHF and located dorsally and medially. It is currently thought that the FHF will give rise to the linear heart tube and that cells from the SHF differentiate later, and are added to the inflow (venous) and outflow (arterial) poles during cardiac looping.
FIGURE 18.1 Schematic presentation of cellular contribution to heart development with special focus on role of epicardium and EPDCs during normal development, disease, and repair processes. Four mesodermal cell lines (cardiomyocytes, endocardium, epicardium, and endothelium) are considered to form the main building blocks of the heart. The differentiation of each line is depicted together with the main interactions with the other cell lines. The most frequent EPDC-related congenital malformations and (acquired) disease processes are boxed in green, while three cardiac (stem) cell populations that may become reactivated are presented on the far right side. aPEO, arterial proepicardial organ; CMPCs, cardiomyocyte progenitor cells; ECs, endothelial cells; EPDCs, epicardially-derived cells; vPEO, venous proepicardial organ; VSMCs, vascular smooth muscle cells. (From Gittenberger-de Groot AC, Winter EM, Bartelings MM, et al. The arterial and cardiac epicardium in development, disease and repair. Differentiation. 2012;84:41–53; Figure 1 (p. 43), with permission.)
FIGURE 18.2 Location of cardiogenic cells in the epiblast prior to gastrulation. (From Kirby ML. Cardiac Development. Oxford, UK: Oxford University Press; 2007:273; Figure 3.2 (p. 23), with permission.)
FORMATION OF THE HEART TUBE
The primitive linear heart tube is formed during the fourth week of development from the FHF cells. Cephalocaudal and lateral folding of the embryo brings the heart fields ventrally into a rostrocaudal orientation ventral to the foregut, caudal to the stomatopharyngeal membrane (future mouth) and cranial to the diaphragm (6,7). It is no longer thought that the cardiogenic fields fuse in a zipper-like fashion cranially to caudally, but that the cranial portions of the cardiogenic fields which become caudal after folding are the first to fuse (Fig. 18.3C–G). A population of cells from cardiogenic mesoderm (hemangioblasts) concomitantly undergoes de novo vasculogenesis to form ‘blood islands’ of endothelial cells that coalesce to form a hollow endothelial tube that comes to lie between the developing myocardium and the endoderm (8).
The heart tube is a two-layered structure with an endothelial layer forming the endocardium and surrounded by cells forming the myocardium. The myocardium is primitive in that the myocytes have few contractile elements, a poorly developed sarcoplasmic reticulum, high automaticity, and few gap junctions (9). The endocardium becomes continuous with the endothelium of the developing vasculature. Between the myocardium and endocardium is the cardiac jelly, a thick acellular matrix secreted by the myocardium. Flow in the heart tube is unidirectional, with cardiac contractions starting around day 22 and circulation through the embryo beginning around day 26 (10). Automaticity is highest at the venous pole so that a slow peristaltic contraction moves blood from the venous to the arterial pole.
Blood vessels are formed by coalescence of angioblasts (mesodermal cells) into networks of tiny endothelial-lined channels that connect to form vascular networks (vasculogenesis). These networks grow by sprouting and branching of vessels (angiogenesis). Cells from either mesoderm, neural crest, or epicardium (depending on body site) cover the endothelial cells and form vascular smooth muscle to stabilize the vessel wall (arteriogenesis) (11,12).
CARDIAC LOOPING
Looping (days 23 to 28) is an essential process for bringing the tubular configuration into the correct conformation for chamber specification, septation, and the formation of systemic and pulmonary pathways (13,14). Cardiac looping is the first indication of left–right asymmetry in the developing vertebrate and is established during gastrulation by cilia of the primitive node (15). The heart tube is initially straight in the ventral midline with paired venous limbs at its caudal end and a single arterial outlet connecting the aortic sac to the aortic (pharyngeal) arch arteries at its cranial end (Fig. 18.4). Looping begins with ventral bending, loss of suspension from the dorsal mesocardium (which suspends the heart tube from the foregut), and rotation around the craniocaudal axis to the right (dextro- or D-looping), thereby bringing the left side of the tube to a ventral position (14). Subsequent looping produces a two-dimensional S-shaped loop when viewed ventrally. The heart tube lengthens by the addition of cardiomyocytes and endocardial cells as a continuous stream from the SHF to the venous (inflow) and arterial (outflow) poles. The poles are the only entryway for the addition of new cells from the SHF (16). The ventricular bend then shifts caudally and the primitive atria and inflow myocardium shift cranially, resulting in convergence of the inflow and outflow poles. The common atrium expands to the right and left forming two pouches that will become the right and left atrial appendages (14,17). After looping, there is rotation (untwisting) of the outflow tract that results in a leftward shift of the outflow tract. The process of wedging brings the aortic valve behind the pulmonary trunk and between the mitral and tricuspid valves (16) (Fig. 18.5). As shown schematically, at the end of looping the atrioventricular (AV) canal is connected to the primitive left ventricle and the outflow tract to the primitive right ventricle (Fig. 18.6). The variably named segments of the heart from inflow to outflow are the sinus venosus, common atrium, AV canal, presumptive left ventricle, presumptive right ventricle, conus arteriosus, and truncus arteriosus (often referred to as the conotruncus).
FIGURE 18.3 Folding of the embryo and formation of the heart tube. Panel A: The embryo starts as a flat disc containing the three germ layers, the ectoderm (Ecto), mesoderm (Meso), and endoderm (Endo). Panels A–A´´´: With ongoing folding of the embryo, the embryonic gut that runs from the stomatopharyngeal membrane (SM) to the cloacal membrane (CM) is formed. The heart (HT) becomes positioned ventrally to the foregut (FG), caudally to the head, and cranially to the umbilical cord and transverse septum (TS). HN Hensen’s node or primitive node. Panel B: Division of the heart-forming field into the FHF (1), which will give rise to the linear heart tube and the SHF (2), which will remain in continuity with the FHF during subsequent development and from which cardiomyocytes are added to the developing heart. In reality, the strict borders drawn here are gradual. PM pharyngeal mesoderm. Panels C–G: Formation of the heart tube from a flat horseshoe-shaped cardiac crescent to a tube. Folding of the embryo brings the lateral portions of the cardiac crescent (red line) together to form the ventral part of the heart tube, while the medial portions of the cardiac crescent (blue line) will form the dorsal part of the heart tube, which is suspended from the foregut by the dorsal mesocardium (DM). After the DM closes and the suspension from the FG is lost, cells of the SHF can only be added to the heart via the arterial and venous poles (AP and VP). (From Sylva M, van den Hoff MJ, Moorman AF. Development of the human heart. Am J Med Genet A. 2014;164:1347–1371; Figure 2 (p. 1350), with permission.)
FIGURE 18.4 Steps in looping of the heart tube. The heart tube forms ventral to the foregut and is open to it at first. Then the heart tube is suspended at its nascent inner curvature from the ventral foregut by the dorsal mesocardium which quickly disappears. Ventral bending occurs first, followed by rotation to the right which brings the left side of the tube to the front and the inner curvature to the left side. Subsequent looping to form the S-shaped loop involves addition of cells at the inflow and outflow poles. DM, dorsal mesocardium; FG, foregut. (From Kirby ML. Cardiac Development. Oxford, UK: Oxford University Press; 2007:273; Figure 7.1 A (p. 88), with permission.)
CARDIAC SEPTATION
Cardiac septation begins after looping between 27 and 37 days with four simultaneous processes dividing the heart into four chambers and separate systemic and pulmonary circulations (18).
Atrial septation occurs in phases to maintain right-to-left atrial shunting and encompasses the septum primum, septum secundum, and AV canal septum. The septum primum grows as a muscular crescent from the craniodorsal wall of the atrium toward the AV endocardial cushions (Fig. 18.7). The leading edge is covered by a mesenchymal cap derived by epithelial-to-mesenchymal transformation of the septal endocardium (19). The mesenchymal cap is continuous with the dorsal mesenchymal protrusion and is critical for fusion of the septum primum with the fused AV cushions (20). The interatrial communication between the leading edge of the septum primum and the AV cushions is the ostium primum. Before fusion of the septum primum with the AV cushions is complete, the septum primum detaches from the roof of the atrial cavity to produce a secondary atrial foramen, the ostium secundum. With incorporation of the sinus venosus and its right horn into the dorsal wall of the right atrium (RA), a new crescent-shaped fold called the septum secundum forms between the septum primum and the left leaflet of the sinoatrial valve. The septum secundum does not fuse with the AV cushions but remains open as the foramen ovale. The foramen ovale is an obliquely elongated cleft through which blood passes from the RA to the left atrium (LA). The upper part of the septum primum remains as a flap valve which postnatally fuses with the edges of the foramen ovale to form the fossa ovalis. The muscular septum between the inferior rim of the fossa ovalis and the AV valves is the AV canal septum. The left horn of the sinus venosus becomes smaller (with regression of the left superior vena cava [SVC]) and is incorporated into the AV groove to become the coronary sinus. The dorsal wall of the LA enlarges with incorporation of the pulmonary vein and its surrounding myocardium.
FIGURE 18.5 Steps in aortic wedging. The aortic side of the outflow tract nestles between the mitral and tricuspid valves as the outflow myocardium is remodeled. AVC, atrioventricular canal. (From Kirby ML. Cardiac Development. Oxford, UK: Oxford University Press; 2007:273; Figure 7.1 C (p. 88), with permission.)
FIGURE 18.6 Ventricular looping. Schematic drawing (frontal views) of the four components of normal ventricular looping: (1) ventral bending transforms a straight tube into a curved tube whose outer curvature is formed by its ventral wall; (2) torsion around its original craniocaudal axis transforms a curved tube into a helically wound loop which appears c-shaped in frontal views; (3) caudal shift of the ventricular segment; (4) untwisting is characterized by ventral and leftward shift of the proximal outflow tract, ventral shift of the primitive right ventricle, and rightward shift of the AV canal. A, common atrium; LV, embryonic left ventricle; O, outflow tract; RV, embryonic right ventricle. (From Männer J. The anatomy of cardiac looping: a step toward the understanding of the morphogenesis of several forms of congenital cardiac malformations. Clin Anat. 2009;22:21–35; Figure 8 (p. 29), with permission.)
FIGURE 18.7 Schematic drawing illustrating septation of the atria and primary foramen. Panel A: Chamber-forming heart with the AV canal (AVC) and outflow tract (OFT) cushions and ridges. The two arrows going down through the AVC into the ventricle represent blood flow during diastole. The two arrows pointing toward the OFT represent blood flow during systole. Note that the primary foramen (PF) is the cross road of the blood running from the RA to the right ventricle (RV), and the blood running from the left ventricle (LV) to the OFT. Panels B-F: Sagittal sections at the level of the dotted line in Panel A. Panel B: The ventral and dorsal endocardial cushions (1 and 2, respectively) are growing toward each other. Panel C: The primary atrial foramen (PAF, ostium primum) is closing due to ingrowing of the primary atrial septum (PS, septum primum), with its mesenchymal cap (MC), the dorsal mesenchymal protrusion (DMP), and the endocardial cushions. Panels C and D: In the PS small holes appear and merge to form the secondary foramen (SF, ostium secundum). Panels D-F: The secondary septum (SS, septum secundum) grows to the right side of the PS covering the SF and the rest of the PS, and leaving at the right surface of the atrial septum only the oval fossa (OF) uncovered. A, atrium; LA, left atrium; V, ventricle; 3 and 4 septal and parietal outflow tract ridges. (From Sylva M, van den Hoff MJ, Moorman AF. Development of the human heart. Am J Med Genet A. 2014;164:1347–1371; Figure 9 (p. 1360), with permission.)
Ventricular septation begins with differentiation and reinitiation of cell division by the primary myocardium of the outer curvature. Disappearance of the cardiac jelly leads to formation of trabeculations on the luminal side to produce a spongy-type myocardium. In the ballooning model of chamber formation, the ventricles expand (“balloon outward”) caudally in a pouch-like fashion on either side of the bulboventricular groove (21) (Fig. 18.8). Growth of the trabeculae is by proliferation at the bases. Formation of the compact myocardium occurs later with infiltration of epicardially-derived fibroblasts, at which time proliferation in the trabeculations ceases. The muscular portion of the ventricular septum is formed by apposition of the outwardly ballooning ventricles. The primary interventricular foramen is the space between the free rim of the muscular septum and the fused AV cushions. The foramen initially provides the entire outflow from the primitive left ventricle into the primitive right ventricle. Only after development of the AV canal will the RA communicate directly with the right ventricle (Fig. 18.7). Closure of the interventricular foramen occurs by fusion of three structures: the muscular ventricular septum, the AV endocardial cushions (AV septum), and the endocardial cushions of the outflow tract (conal septum). The site of fusion is represented by the membranous part of the mature ventricular septum, suggesting a mechanistic rationale for the high frequency of membranous or perimembranous ventricular septal defects (VSDs). Ventricular septation is usually complete by 42 days of gestation (7).
The AV canal initially connects the primitive atrium exclusively to the primitive left ventricle. Myocardial synthesis of extracellular matrix forms mounds of cardiac jelly at the AV junction. Endocardial cells overlying these mounds invade the cardiac jelly and undergo endocardial-to-mesenchymal transformation (EMT) into fibroblast-like mesenchymal cells to form the AV endocardial cushions (22). The superior cushion is associated with the inner curvature and the inferior cushion with the outer curvature of the looped heart, while the lateral cushions are at the left and right heart borders (23). The superior and inferior cushions fuse in the midline to form the AV septum, resulting in separation of the ventricular inflow into left (mitral) and right (tricuspid) orifices. The atrial portion of the AV septum extends from the valve annuli to fuse with the septum primum, and the ventricular portion extends from the valve annuli to fuse with the muscular ventricular septum, thereby bringing the atrial and ventricular septa into continuity. Expansion of the AV canal and right ventricle to the right results in direct communication between the presumptive RA and presumptive right ventricle (Fig. 18.7). The fibroblast-like cells of the cushions are replaced by myocardial cells (myocardialization). The AV valves are derived from the endocardial cushions; the septal leaflet of the tricuspid valve and the anterior leaflet of the mitral valve are derived from the fused inferior and superior endocardial cushions, while the mural leaflets are derived from the lateral cushions (24,25) (Fig. 18.9). The valve leaflets are formed by separation of endocardial cushion tissue from the myocardium by the process of delamination. Delamination of the myocardial layer together with coalescence of trabeculae forms the papillary muscles, while thinning of the cushion tissue forms the valve leaflet and chordae tendineae. AV valve morphogenesis is one of the most prolonged aspects of human cardiac development (5th to 12th weeks).
FIGURE 18.8 Formation of the cardiac chambers. Panels A–D: Developmental series of mouse embryos. Whole mount RNA in situ hybridization for the embryonic chamber marker atrial natriuretic factor (ANF) is used as a marker for differentiation into chamber myocardium. Panels E–G and I–L: Schematic drawings of these chamber-forming hearts. Gray, primary myocardium; blue, chamber-forming myocardium; arrows in K indicate the expansion of the chambers eventually leading to the adult configuration, with the ventricles positioned ventrocaudally to the atria. Panel H: Electron micrograph of a CS14 human heart, demonstrating the similarity with the mouse E11.5 heart and the schematic shown in Panel L. For didactic purposes, in the schematic in Panel L, the outflow tract is hinged toward the right side, in vivo it is positioned ventrally to the heart, as depicted in Panels D and H. Panels A, E, and I: The heart tube (HT) consists solely of primary myocardium from venous (VP) to arterial pole (AP). Panels B, F, and J: The first chamber to start ballooning is the embryonic ventricle (V) at the outer curvature of the heart. Panels C, G, and K: The heart tube has started to loop and acquire an S shape. An embryonic left and right ventricle are now visible. The atria (A) also start to balloon toward the left and right side. The myocardium of the outflow tract (OFT), inner curvature (IC), and AV canal (AVC) remains as primary myocardium. RA, right atrium; LV, left ventricle. Equivalent Carnegie stage (CS) noted on left margin of figures. (From Sylva M, van den Hoff MJ, Moorman AF. Development of the human heart. Am J Med Genet A. 2014;164:1347–1371; Figure 4 (p. 1355), with permission.)
The outflow tract is a tubular structure arising from the primitive right ventricle that must gain alignment with the primitive left ventricle and undergo septation to form the pulmonary artery (PA) and aorta. It is traditionally divided into the conus (proximal), the truncus (middle), and the aortic sac (distal). Neural crest cells are essential for outflow tract septation, remodeling of the aortic arch arteries, semilunar valvulogenesis, and formation of the cardiac ganglia and neurons, and possibly the conduction system (26). The conal and truncal cushions are endocardial cushions, also formed by EMT of the overlying endocardium, that spiral into the outflow tract. The aorticopulmonary septum begins as a shelf in the dorsal wall of the aortic sac between the fourth and six pairs of aortic arch (pharyngeal arch) arteries and grows toward the truncus (27). Cardiac neural crest cells migrate through pharyngeal arches 3, 4, and 6, and with mesenchymal cells from the pharynx, invade the truncal cushions to form two centrally placed columns in the shape of an upside down “U” (28). Neural crest cells also invade the conal cushions (Fig. 18.10). The truncal columns will fuse with the conal cushions proximally and the aorticopulmonary septum distally. Myocardial cells invade the conal cushions (myocardialization) which fuse with each other as well as with the AV-cushion tissue and the crest of the muscular ventricular septum. Early in development both the subpulmonary conus and the subaortic conus are situated above the right ventricle. The developing pulmonary valve is initially posterior and to the left of the developing aortic valve. There is morphogenetic rotational movement of the pulmonary valve from posterior to anterior on the left side of the aortic valve to assume its final position of anterior and lateral to the aortic valve (Fig. 18.5) (29). This movement of the pulmonary valve is thought to be caused by development of the subpulmonary conus (infundibulum), which also causes the pulmonary valve to be in a superior position relative to the aortic valve. Resorption and shortening of the subaortic conus causes the aortic valve to sink inferiorly and posteriorly so that it comes to lie directly over the left ventricle and in fibrous continuity with the mitral valve (30). Pulmonary valve rotation is associated with spiraling of the aorticopulmonary septum so that the developing aorta will connect with the rightward and cranial component of the aortic sac, and the developing pulmonary trunk will connect with the leftward and caudal component of the aortic sac (31).
The semilunar valves develop shortly after outflow tract septation by remodeling in the truncal cushions and contributions by neural crest (Fig. 18.9). Three ridges of mesenchymal tissue covered by endocardium protrude into the lumen on each side of the divided truncus. The valve sinuses are formed by excavation on the arterial (distal) face of the ridges, after which the leaflets remodel by mesenchymal apoptosis along with blood flow and shear stress into the delicate fibrous tissue of the mature valve. Valve fibroblasts are predominantly derived from the endocardium, with some contribution by fibroblasts derived from the epicardium (26).
EPICARDIUM AND CORONARY ARTERY DEVELOPMENT
The epicardium and coronary arteries arise from the extracardiac proepicardial organ and splanchnic mesoderm of the ventral pharynx and not from cardiogenic mesoderm (32–34). The venous proepicardial organ is derived from mesenchyme near or in the liver, giving rise to protrusions at the venous pole that will cover the atria, AV canal, and ventricles (Fig. 18.10). Epicardium that will cover the outflow tract is derived from splanchnic mesoderm of the ventral pharynx near the arterial pole. A subset of epicardial cells undergoes EMT and migrates into the subepicardial space. These epicardially-derived cells (EPDCs) invade the myocardium and endocardial cushions and differentiate into fibroblasts (interstitial, adventitial coronary, and annulus fibrosus), coronary smooth muscle cells, and coronary endothelial and hematopoietic cells. The epicardium is essential for myocardial growth and formation of the thick compact myocardium by the interstitial fibroblasts.
FIGURE 18.9 Development of the AV and outflow tract (OFT) valves and their contributing tissues. Panels A–H: Sections through mouse hearts in a plane comparable to the schematic heart in Panel S. Panels B, D, F, and H: The lineage contributions of the epicardium is displayed at different developmental stages. The epicardial lineage marker WT1 was used; epicardial lineage is depicted in red, myocardium in green. Panels I–P: Schematic drawings of valve development in both the AV canal and outflow tract. Red, epicardium (Ep); gray, primary myocardium; and yellow, endocardial cushion tissue (EC). Note that in the outflow tract the cells are primarily neural crest derived and not endocardial derived as in the AV canal. Panel P: The contribution of the different cushions and ridges to the eventual valves (Panel T) is depicted. Panels Q and R: Display the proepicardium (PE) in a 3-day-old chick embryo. Panel R: The PE is attached to the heart tube (HT) and spreading out to form the epicardium. Ao, aorta; DAVC, dorsal AV-cushion; IR, intercalated ridge; LA, left atrium; LC, lateral cushion; LV, left ventricle; MC, mesenchymal cap; PR, parietal ridge; PT, pulmonary trunk; RA, right atrium; RV, right ventricle; S, septum; SR, septal ridge; VAVC, ventral AV-cushion. Equivalent Carnegie stage (CS) noted on left margin of figures. (From Sylva M, van den Hoff MJ, Moorman AF. Development of the human heart. Am J Med Genet A. 2014;164:1347–1371; Figure 11 (p. 1364), with permission.)
The early myocardium is initially avascular with nutrients diffusing from venous sinusoids (trabecular channels) in the inner trabecular zone. After looping and with formation of the epicardium, a subepicardial endothelial plexus forms from EPDCs and undergoes vasculogenesis, angiogenesis, and arteriogenesis (coating of endothelial channels by smooth muscle and pericytes) (35). These vessels penetrate the atrial and ventricular walls to establish a midmyocardial network, which has a higher density on the outer epicardial side (33). Some of the channels will communicate with the intratrabecular venous sinusoids. The subepicardial vascular plexus remodels into the coronary arteries and veins with adult branching characteristics. Finally, there is ingrowth of the peritruncal coronary plexus into the base of the aorta, but not the PA (36,37) (Fig. 18.11). The multiple small vessels coalesce to form the main stems and orifices of the right and left coronary arteries in the right and left aortic sinuses, respectively, while the channels in the remaining (noncoronary) sinus regress.
FIGURE 18.10 Schematic representation of the developing heart. Simplified model of a heart prior to ventricular septation. The roof of the atrium (A, light brown) is closed; therefore, atrial septation is not shown here. The anterior walls of the left ventricle (LV) and right ventricle (RV) are opened to reveal the inside. The interventricular foramen (primary foramen) is present. The inlet septum (IS) is connected to the inferior AV-cushion (iAVC). The epicardium (olive green) covers most of the surface of the heart, but the venous proepicardial organ (vPEO; between liver and venous pole) and the arterial PEO (aPEO) surrounding the arterial pole are still present. The SHF-derived part of the RV is shown in yellow, the endocardial cushions in light blue, neural crest cells in lavender, and the gut (G) including liver in green. AVC; atrioventricular canal; M, mitral ostium; OFT, outflow tract; PV, pulmonary vein; sAVC, superior AV-cushion; T, tricuspid ostium; VCI, inferior cardinal vein. (From Gittenberger-de Groot AC, Bartelings MM, Poelmann RE, et al. Embryology of the heart and its impact on understanding fetal and neonatal heart disease. Semin Fetal Neonatal Med. 2013;18:237–244; Figure 1b (p. 238), with permission.)
CONDUCTION SYSTEM
The primitive pacemaker is located at the junction of the sinus venosus and primitive atrium and is composed of the fastest beating cardiomyocytes rather than morphologically distinct conducting cells (38). A depolarizing impulse spreads from this region toward the outflow tract, producing a peristaltic contraction wave that pushes blood from the venous pole to the arterial pole. Myocardial cells of the atria and ventricles differentiate into working and conducting phenotypes during elongation of the heart tube (39). The conducting phenotypes are located in the inflow tract, AV canal, and outflow tract, and differ from the working phenotype by having sparse gap junctions and slow conduction. The sinoatrial node (SAN) appears around 32 days and develops from slow-conducting myocardial cells at the junction of the right common cardinal vein and atrium, becoming comma shaped with the head at the junction of the SVC and RA and the tail in the crista terminalis (40). Specific conduction pathways through the atrium are no longer thought to exist (38,41). The AV node (AVN) appears around 33 days and develops from slow-conducting myocardium in the dorsal AV canal. The AV (His) bundle, right and left bundle branches, and Purkinje fibers develop from fast-conducting ventricular working myocardium (39). Electrical continuity between the atrial and ventricular myocardium is lost when epicardially-derived fibroblasts populate the AV groove and invade the myocardium inferior to the AVN to form the annulus fibrosus. The AV conduction system then provides the only electrical continuity between the atria and ventricles.
FIGURE 18.11 Left (LCA) and right (RCA) coronary artery development. Panel A: Multiple channels penetrate the aortic (Ao) wall in all of the aortic sinuses from the peritruncal ring. Panel B: Only the ones in the right and left sinuses survive and coalesce to form the main stems of the LCA and RCA. PT, pulmonary trunk; g, cardiac ganglia; S, aorticopulmonary septum. (From Waldo KL, Willner W, Kirby ML. Origin of the proximal coronary artery stems and a review of ventricular vascularization in the chick embryo. Am J Anat. 1990;188:109–120, with permission.)
GREAT ARTERIES
The dorsal aortae form as parallel longitudinal channels in the mesoderm dorsal to the foregut on either side of the notochord, and gain connection to the arterial (outflow) pole of the developing heart. During development of the pharyngeal arches, each arch receives its own artery and cranial nerve. The aortic sac, the most distal part of the outflow tract, connects to the left and right dorsal aortae by the artery of each pharyngeal arch, the pharyngeal arch or aortic arch arteries (PAAs) (42). Cardiac neural crest cells migrate into the mesoderm of pharyngeal arches 3, 4, and 6, and surround the endothelial cells to form smooth muscle (43–46). The PAAs are not all present simultaneously, but develop in a cranial to caudal sequence in a symmetric pattern as the heart migrates caudally into the chest (Fig. 18.12). The fifth aortic arch is rudimentary in humans and does not contribute to the mature arterial system. Transformation and regression of the symmetric dorsal aortae and PAAs into the asymmetric adult pattern is critically dependent on neural crest cells. All the outflow of the heart is initially carried by the first and second arches, which regress and remodel on days 26 and 29 to form part of the external carotid artery and parts of the hyoid and stapedial arteries, respectively. The third arch appears on day 28 and persists as the common carotid and proximal internal carotid arteries (distal internal carotid arteries derived from dorsal aorta). The fourth arch also appears on day 28; the left fourth arch produces the arch of the aorta between the left common carotid artery and the left subclavian artery, while the right fourth arch persists as the proximal right subclavian artery. The subclavian arteries are derived from the seventh intersegmental arteries. The sixth arch (also known as the pulmonary arch) forms on day 29. The proximal part of the sixth arch on each side becomes the proximal segment of the left and right PA. The distal sixth arch regresses on the right (eliminating continuity of the sixth aortic arch on the right with the dorsal aorta) and persists on the left as the ductus arteriosus. The normal left aortic arch pattern develops because of regression of the right dorsal aorta between the origin of the seventh intersegmental artery and the junction with the left dorsal aorta. The left dorsal aorta distal to the sixth arch forms the descending aorta distal to the left subclavian artery. The course of the recurrent laryngeal nerves become different on the left and right because of caudal shift of the heart and regression of various parts of the aortic arches.
The dorsal aortae remain paired in the region of the pharyngeal arches, but fuse into a single dorsal aorta in a caudal to cranial direction up to the seventh somite. Intersegmental arteries arise from the dorsal aortae to supply the spinal cord and developing somites (47). Longitudinal anastomoses form between the intersegmental arteries, with those from the first to the seventh forming the vertebral artery. Regression of the first six intersegmental arteries leads to the vertebral artery arising from the seventh intersegmental artery which will form part of the subclavian artery.
FIGURE 18.12 Graphical representation of the arterial changes during transformation of the truncus arteriosus, aortic sac, PAAs (pharyngeal arch arteries), and dorsal aortas into the adult arterial pattern. Vessels not colored are not derived from these structures. Panel A: PAAs at 6 weeks; by this stage the first two pairs of PAAs have largely regressed. Panel B: PAAs at 7 weeks; the parts of the dorsal aortas and PAAs that normally regress are indicated with broken lines. Panel C: Arterial arrangement at 8 weeks. Panel D: Arterial vessels of a 6-month-old infant. Note that the ascending aorta and pulmonary arteries are considerably smaller at 8 weeks of development than in infancy, representing the relative flow through these vessels at the different stages of development. (From Moore KL, Persaud TVN, Torchia MG. The Developing Human: Clinically Oriented Embryology with Student Consult Online Access. 9th ed. Philadelphia, PA: Saunders; 2013:540; Figure 13.39 (p. 326), with permission.)
PULMONARY VEINS
The primary (common) pulmonary vein arises by formation of an endothelial-lined channel within the dorsal mesocardium (17). By the end of the first month, it connects the pulmonary venous plexus within the lung buds to the left atrial wall just to the left of the septum primum. The pulmonary venous plexus loses its connections to the splanchnic plexus once connection to the atrium is established. Incorporation of the common pulmonary vein to the second bifurcation into the back wall of the LA results in four separate pulmonary vein orifices (16). Muscularization of the pulmonary veins from the atrium to the lungs then occurs.
SYSTEMIC VEINS
Like the great arteries, the major systemic veins are formed by vasculogenesis and are initially bilaterally symmetric. Three pairs of venous systems, the cardinal, umbilical, and vitelline veins, appear in the fifth week and drain into the right and left horns of the sinus venosus (Fig. 18.13). While the arterial system regresses on the right, the venous system regresses on the left, that is, the connections of the left-sided cardinal, umbilical, and vitelline veins with the left horn of the sinus venosus regress, with the left horn becoming the coronary sinus.
The umbilical veins originate in the chorionic villi and pass on either side of the liver, carrying oxygenated blood to the embryo. The right umbilical vein regresses; the left umbilical vein loses its connection with the sinus venosus and forms a direct communication with the hepatic sinusoids. Eventually it forms the ductus venosus, which bypasses the sinusoidal plexus of the liver, and is the only vein carrying blood from the placenta. After birth, the left umbilical vein and ductus venosus are obliterated, forming the ligamentum teres and ligamentum venosum, respectively.
The vitelline veins drain the gut and its derivatives, forming a plexus around the foregut and connecting with the hepatic sinusoids. Following regression of the left vitelline vein, the venous system of the gut drains to the heart via the right vitelline vein. The connection of the right vitelline vein with the right horn of the sinus venosus persists as the terminal (suprahepatic) portion of the inferior vena cava (IVC). The right vitelline vein below the liver becomes the portal vein and superior mesenteric vein, with some of the left-to-right vitelline vein connections becoming the splenic and inferior mesenteric veins.
The cardinal veins drain the embryonic head, neck, and body wall. The anterior (superior) cardinal veins drain the head, neck, upper limbs, and part of the chest, while the posterior (inferior) cardinal veins drain the remainder of the embryo. On each side, the anterior and posterior cardinal veins join to form the common cardinal vein before entering the respective sinus horn. The left anterior cardinal vein loses its connection with the left horn of the sinus venosus and becomes connected to the right anterior cardinal vein to form the left brachiocephalic vein; the right brachiocephalic vein is that part of the anterior cardinal vein superior to this anastomosis. The cranial portion of the left anterior cardinal vein persists as the left internal jugular vein, while the remnant on the surface of the heart persists as the oblique vein of the LA. The SVC is formed by the right common cardinal vein and the proximal portion of the right anterior cardinal vein (between the intercardinal anastomosis and the RA). Development of the IVC is complex, with the posterior cardinal, supracardinal, and subcardinal veins providing the segments. Regression of the left-sided channels and persistence of the right-sided channels results in the typical right-sided location of the IVC.
CARDIAC INNERVATION
Development and maturation of cardiac innervation is slow and not fully functional until after birth. As reviewed by Kirby, all of the postganglionic autonomic neurons are derived from neural crest (48). Sympathetic postganglionic neurons originate in trunk neural crest and contribute to the primary sympathetic chain and dorsal root ganglia. Sympathetic fibers are found throughout the atria and ventricles. Parasympathetic postganglionic neurons originate from neural crest cells, between the midotic placode and somite 3, which migrate through the pharyngeal arches to the cardiac plexus and cardiac ganglia. Parasympathetic fibers are found throughout the atrial myocardium, but are scant in the ventricles. The primary neurotransmitters of the sympathetic and parasympathetic neurons are norepinephrine and acetylcholine, respectively. Parasympathetic innervation develops first, so that parasympathetic-cholinergic control becomes functional before sympathetic-adrenergic neural control. As autonomic receptor-mediated effector mechanisms are present before functional innervation, the heart can respond to circulating catecholamines to lessen the effects of hypoxia and bradycardia (49).
EPIDEMIOLOGY OF CHD
ETIOLOGY
Advances in genomics, proteomics, transgenesis, imaging, and integrative systems biology have increased our understanding of the etiology and mechanisms of CHD (2). Mostly based on research in nonhuman species, the inheritance of many lesions is multifactorial and probably due to the interactions between several genes and modulating environmental factors, including known teratogens (alcohol, retinoic acid, anticonvulsants, thalidomide, and lithium), infectious agents (rubella), or maternal disease (diabetes mellitus, systemic lupus erythematosus, phenylketonuria, and obesity) (50,51). Genetic alterations range from whole chromosome to single nucleotide changes (52), with some syndromes having more than one genetic etiology.
FIGURE 18.13 Development of the venous system. Dorsal views of the developing heart are shown. Panel A: During the 4th week, showing the primordial atrium, sinus venosus and veins draining into them. Panel B: At 7 weeks, showing the enlarged right sinus horn and venous circulation through the liver (organs not drawn to scale). Panel C: At 8 weeks, showing the adult derivatives of the cardinal veins depicted in Panels A and B. (From Moore KL, Persaud TVN, Torchia MG. The Developing Human: Clinically Oriented Embryology with Student Consult Online Access. 9th ed. Philadelphia, PA: Saunders; 2013:540; Figure 13.5 (p. 294), with permission.)
Chromosomal defects comprise excesses or deficiencies of multiple genes that vary from the aneuploidies (numerical departures from the norm) to deletion syndromes and microdeletions. The most common aneuploidies associated with CHD are the trisomies—trisomy 21 (Down syndrome), trisomy 18 (Edwards syndrome) and trisomy 13 (Patau syndrome); the monosomy 45,X (Turner syndrome); and the tetrasomies 22p (cat eye syndrome), and 12p (Pallister–Killian syndrome). The deletion syndromes define the position of loss of functionally dominant genes. The 22q11 deletion is the most common known autosomal deletion and is associated with abnormalities of neural crest migration, resulting in conotruncal malformations and pharyngeal arch defects. Syndromes associated with 22q11 deletion include DiGeorge, Shprintzen (velocardiofacial), and Opitz. Other deletion syndromes of importance to the pediatric anesthesiologist are Williams (7q11.23), Alagille (20p11), cri-du-chat (5p15), and Wolf–Hirschorn (4p-).
Gene mutations (gene(s) defects) may cause defects in more than one organ system and produce recognizable syndromes. The most well-known associated with cardiovascular involvement include CHARGE (CHD7, SEMA3E), Alagille (JAG1, NOTCH2), Cornelia de Lange (PTPN11, NIPBL, SMC1A), Noonan (PTPN11, KRAS, SOS1, RAF1, NRAS, BRAF, SHOC2), Holt–Oram (TBX 5), Opitz (MID1) Leopard (PTPN11, RAF1) Marfan (FBN1), Ehlers–Danlos (ADAMTS2, COL1A1, COL1A2, COL3A1, COL5A1, COL5A2, PLOD1, TNXB), Ellis van Creveld (EVC, EVC2), and some mucopolysaccharidoses (52).
Extracardiac malformations are present in approximately 25% of children with CHD (53,54). Many of these are not part of a syndrome, and it is unclear whether the associations arise from a common genetic origin or a disturbance during fetal development.
INCIDENCE AND PREVALENCE
CHD is the most common birth defect and accounts for almost one-third of all major congenital anomalies (55,56). The incidence of CHD is 75/1,000 live births if tiny muscular VSDs present at birth and other trivial lesions are included (56). If only moderate and severe forms of CHD are considered, the incidence is 6/1,000 live births. There is no evidence for differences in incidence in different countries. Although CHD is by definition present at birth (con = together, genitus = born (L.)), it may only present later in childhood or adult life. VSDs are the most frequent lesion (Table 18.1).
The incidence and prevalence of CHD are increasing (57,58). Factors accounting for this are a decrease in overall and infant mortality of patients with moderate to severe forms of CHD, an increased survival to adulthood, and the fact that children of parents with CHD have an increased likelihood of CHD (58,59). In developed countries, almost 90% of children with CHD have the prospect of surviving into adulthood (60).
CIRCULATORY PHYSIOLOGY
FETAL CIRCULATION
The fetal circulation differs both functionally and morphologically from that in the adult. Although most of the information regarding the fetal circulation has been derived from the sheep, observations made in previable human fetuses and ultrasonographic studies in the human fetus indicate that the course and distribution of the circulation are similar to the fetal lamb (61).
The fetal circulation is arranged in parallel, rather than in series, whereby the right ventricle delivers blood to both the pulmonary circulation and the systemic circulation (through the ductus arteriosus) and the left ventricle pumps blood to the systemic circulation and placental circulation. This pattern of circulation allows fetal survival despite complex cardiac lesions, with the postnatal changes directly impacting the clinical manifestations of CHD. Oxygenated blood from the placenta (PO2 = 32 to 35 mm Hg, oxygen saturation = 80%) passes through the umbilical vein to the liver; slightly more than half bypasses the hepatic veins and portal venous system by passing through the ductus venosus into the IVC. Streaming of blood occurs in the RA whereby the umbilical venous blood is preferentially directed across the foramen ovale into the LA, while the abdominal inferior vena caval blood joins the superior vena caval drainage (PO2 = 12 to 14 mm Hg, oxygen saturation = 40%) and coronary sinus returns to pass across the tricuspid valve into the right ventricle and PA. Because of the high PVR, >90% of blood from the right ventricle (PO2 = 18 to 20 mm Hg, oxygen saturation = 50%) passes from the PA across the ductus arteriosus into the descending aorta, with the remainder passing into the pulmonary circulation. The preferential streaming of umbilical venous blood into the LA distributes blood of higher oxygen content to the left ventricle and ascending aorta so that the coronary arteries and brain (and upper limbs) receive blood of higher oxygen content (PO2 = 25 to 28 mm Hg, oxygen saturation = 65%) than that in the descending aorta (PO2 = 20 to 22 mm Hg, oxygen saturation = 55%). The lower arterial oxygen partial pressure of descending aortic blood and the lower P50 of fetal hemoglobin facilitate oxygen uptake in the placenta.
TABLE 18.1 Incidence of CHD in live born children
The right ventricle has a higher output than the left ventricle in the fetal circulation. This is reflected in the size and thickness of the right ventricle before and after birth. The combined ventricular output is approximately 450 mL/kg/minute, with a ratio of right-to-left ventricular (LV) output of 1.3:1. The large communication (ductus arteriosus) between the aorta and PA allows for equalization of pressures in these vessels and hence the ventricles. Similarly, the LA pressure is only 1 to 2 mm Hg or less than the right atrial pressure.
POSTNATAL (TRANSITIONAL) CIRCULATION
After birth, the lungs take over the gas exchange function of the placenta. With the onset of spontaneous ventilation, there is physical expansion of the lungs with air and an increase in the alveolar oxygen concentration. Both these result in a reduction in the PV resistance and a marked increase in pulmonary blood flow (PBF). Removal of the low-resistance placental circulation from the newborn circulation results in an increase in systemic vascular resistance (SVR) and a rapid drop in systemic venous return to the IVC as the umbilical venous flow is cut off. Constriction of the ductus venosus occurs, and the right atrial pressure decreases, whereas the LA pressure increases with the increase in PBF and pulmonary venous return. When the LA pressure exceeds the right atrial pressure, the flap valve of the foramen ovale closes against the septum secundum, eliminating shunting at the atrial level. Closure of the ductus arteriosus proceeds slowly with contraction of its muscular wall (functional closure) occurring within 10 to 15 hours of birth. The mechanism of ductal closure is not well defined; increases in arterial oxygen tension, declines in circulating prostaglandins after birth, and bradykinin release from lung expansion are thought to play a role. Within a few hours of birth, the PV resistance and pulmonary arterial pressures fall below the SVR and systemic vascular pressure, resulting in a small left-to-right (aorta-to-PA) shunt. Cardiac output increases considerably (30% to 80%) after birth, partly due to increased metabolism.
The separation of the systemic and pulmonary circulations, characteristic of the mature series circulation, is achieved by closure of the foramen ovale and ductus arteriosus. The postnatal circulation is termed a transitional circulation because closure of the ductus arteriosus and foramen ovale are reversible during the first days and weeks of life. The foramen ovale closes functionally at birth, but increases in right atrial pressure can cause the “flap” to reopen and result in right-to-left shunting. Increased right atrial pressure may occur with crying, pain, or other factors known to increase PVR (hypoxia, hypercarbia, acidosis, lung disease, sepsis). Anatomic closure of the foramen ovale occurs between 3 months and 1 year by adhesion of the septum primum (valve of foramen ovale) to the left margin of the septum secundum. In approximately 20% of individuals, the fusion is incomplete resulting in a probe patent foramen ovale (PFO). Although functional closure of the ductus arteriosus occurs in the first 72 hours of life, anatomic closure and formation of the ligamentum arteriosum by endothelial destruction, subintimal proliferation, and connective tissue formation can take 1 to 3 months. Survival of some newborns with CHD is dependent on ductal patency, and as functional closure is a reversible event, prostaglandin E1 infusions can be used to reopen the ductus arteriosus. Similarly, decreases in arterial oxygen tension perinatally can cause reopening of the ductus and left-to-right or right-to-left shunting (62).
MYOCARDIAL FUNCTION IN THE NEWBORN
The fetal and postnatal myocardium is structurally and functionally immature with a limited ability to increase cardiac output, compared to the older child. Increases in cardiac output are mediated primarily through increasing HR, whereas increases in preload cause little or no change in cardiac output (63,64).
Neonatal cardiac physiology is explained by the following:
1. The neonatal myocardium has fewer contractile elements, resulting in limited ability to increase stroke volume (SV) by the Frank–Starling mechanism and difficulty in maintaining cardiac output with increased afterload.
2. A deficiency of elastic elements parallels the deficiency of contractile elements, resulting in reduced ventricular compliance.
3. The lower ventricular compliance and equal muscle mass of the right and left ventricles at birth produce increased ventricular interdependence in that changes in ventricular pressure impact the function of the other ventricle through the ventricular septum to a greater extent. Achievement of the left ventricle to right ventricle mass of 2:1, in response to the pressure and volume changes of the systemic and pulmonary circulations on each ventricle, occurs only several months after birth.
4. Autonomic innervation is incomplete at birth in that parasympathetic innervation, which slows the heart, is fully functional while sympathetic innervation, which increases HR and contractility, is incompletely developed.
PULMONARY VASCULAR RESISTANCE
The small arteries of the fetal lung have a thick medial smooth muscle layer and PVR is very high. Although the PVR falls at birth, the pulmonary vasculature is highly reactive to a number of stimulants. Pulmonary vasoconstriction induced by hypoxia or other pressor stimuli can result in resumption of right-to-left shunting across fetal cardiovascular channels and right-ventricular (RV) dysfunction. Remodeling of the pulmonary vessels occurs, most notably with thinning of the medial smooth muscle layer, so that the PVR reaches adult levels by approximately 6 months of age. Pathologic conditions, including CHD, may significantly interfere with this process of postnatal pulmonary vascular (PV) maturation.
GENERAL PRINCIPLES OF ANESTHETIC MANAGEMENT
The goal of anesthetic management is the application of the pathophysiologic principles of the cardiac lesion to basic clinical anesthesia. The approach is the same whether for cardiac or noncardiac surgery. For cardiac surgery, a detailed understanding of the specific issues related to the repair allows for anticipation of problems during the postbypass and postoperative periods.
THE PATHOPHYSIOLOGIC APPROACH
Identification and classification of defects on the basis of physiology provides an organized framework for the intra- and postoperative anesthetic management (65). Abnormalities of flow produce the pathophysiologic consequences and may be categorized as shunts, mixing, obstruction, and regurgitation. These categories may coexist in more complex disease. In addition to flow abnormalities, coronary ischemia occurs more commonly in CHD than is generally appreciated and needs to be considered in certain settings.
ABNORMALITIES OF FLOW
Shunt Lesions
1. Shunting is the hallmark of CHD. In an anatomic shunt, blood moves from one circulatory system to the other via a communication (orifice) at the level of the cardiac chambers or great vessels. Recirculation of blood produces a physiologic shunt. A physiologic shunt can exist in the absence of an anatomic shunt (e.g., transposition of the great vessels).
2. The direction and magnitude of shunts are variable, both within the cardiac cycle and over long periods of time. For example, with a moderate to large VSD, the normal fall in LV pressure during diastole precedes the fall in RV pressure so that transient right-to-left shunting occurs (66).
3. Anesthesia and operative interventions can have a significant effect on the direction and magnitude of shunting. Factors influencing shunt flow are given by the Hagen–Poiseuille equation: Q = Pπr4/8ηL where Q is flow, P is pressure gradient, r is radius, η is fluid viscosity, and L is tube length.
4. The most important determinants of flow through the shunt are the size of the shunt orifice and the relative outflow resistances on either side of the shunt. Outflow resistance is determined by the pulmonary and SVR, ventricular compliance, and any anatomic obstruction.
5. Anatomic shunts can be characterized as simple or complex.
a. In a simple shunt, there is no fixed obstruction to outflow from the vessels or chambers involved in the shunt. When the shunt orifice is small (restrictive shunt), a large pressure gradient exists across the orifice, and variations in outflow resistance (PVR, SVR) have little effect on shunt magnitude and direction (Table 18.2). In this instance, the magnitude of the shunt is affected by the size of the shunt orifice. When the shunt orifice is large, the shunt becomes nonrestrictive, and the outflow resistance becomes the primary determinant of the magnitude and direction of shunting. When the shunt orifice is very large, no pressure gradient exists between the vessels or chambers involved. In this instance, bidirectional shunting occurs, resulting in complete mixing and a functionally common chamber. Net systemic and PBF is then determined by the ratio of systemic-to-pulmonary vascular resistance.
b. A complex shunt is a simple shunt plus obstruction to outflow. Outflow resistance is a combination of the resistance across the obstructive lesion and the resistance across the pulmonary or systemic vascular bed. When an obstruction is severe, the SVR or PVR distal to the obstruction will have little effect on shunt magnitude or direction (Table 18.3) With complete obstruction to flow, there must be two shunt pathways: one proximal and one distal to the obstruction to provide blood flow around the obstruction.
6. Recirculation of pulmonary venous blood produces a left-to-right (L → R) shunt, that is, flow of blood from the pulmonary venous atrium to the PA produces recirculation of pulmonary venous blood. When the PVR is low, L → R shunting is increased. When pulmonary flow is greater than systemic flow (QP:QS >1), the additional flow does not increase arterial oxygen content but rather places a volume load on the heart (causing systolic and diastolic dysfunction) and necessitates an increase in ventricular volume proportional to the QP:QS increase. When the reserves of HR, preload and contractility have been reached, which will occur sooner with the immature myocardium, further increases in QP:QS will occur at the expense of systemic cardiac output. Pulmonary consequences of left-to-right shunts include:
TABLE 18.2 Simple shunts, no obstructive lesions
Restrictive shunts (small communications) |
Nonrestrictive shunts (large communications) |
Common chambers (complete mixing) |
Large pressure gradient |
Small pressure gradient |
No pressure gradient |
Direction and magnitude more independent of PVR/SVR |
Direction and magnitude more dependent on PVR/SVR |
Bidirectional shunting |
Less subject to control |
More subject to control |
Net QP /Qs totally depends on PVR/SVR |
Examples: small VSD, small PDA, Blalock shunts, small ASD |
Examples: large VSD, large PDA, large aortopulmonary shunts |
Examples: single ventricle, truncus arteriosus, single atrium |
PVR, pulmonary vascular resistance; SVR, systemic vascular resistance; QP, pulmonary blood flow; Qs, systemic blood flow; VSD, ventricular septal defect; PDA, patent ductus arteriosus; ASD, arterial septal defect.
From Odegard KC, DiNardo JA, Laussen PC. Anesthesia for congenital heart disease. In: Gregory GA, Andropoulos DB, eds. Gregory’s Pediatric Anesthesia. 5th ed. Oxford, UK: Wiley-Blackwell; 2012, with permission.
TABLE 18.3 Complex shunts (shunt and obstructive lesion)
Partial outflow obstruction |
Total outflow obstruction |
Shunt magnitude and direction largely fixed by obstructions |
Shunt magnitude and direction totally fixed |
Shunt depends less on PVR/SVR |
All flow goes through shunt |
Orifice and obstruction determine pressure gradient |
Pressure gradient depends on orifice |
Examples: tetralogy of Fallot, VSD and pulmonic stenosis, VSD with coarctation |
Examples: tricuspid atresia, mitral atresia, pulmonary atresia, aortic atresia |
PVR, pulmonary vascular resistance; SVR, systemic vascular resistance, VSD; ventricular septal defect.
From Odegard KC, DiNardo JA, Laussen PC. Anesthesia for congenital heart disease. In: Gregory GA, Andropoulos DB, eds. Gregory’s Pediatric Anesthesia. 5th ed. Oxford, UK: Wiley-Blackwell; 2012, with permission.
a. Increased work of breathing resulting from decreased lung compliance and increased airway resistance (67).
b. Progressive elevation in PVR, particularly in association with high pressures, leads to structural changes in the pulmonary vasculature (PV occlusive disease).
c. Extrinsic compression of the airways or lungs due to dilated pulmonary arteries, left atrial enlargement, massive cardiomegaly, and intraluminal bronchial obstruction from increased intracardiac pressures causing bronchial and lymphatic vessel enlargement (68).
7. Recirculation of systemic venous blood produces a right-to-left (R → L) shunt, that is, flow of blood from the systemic venous atrium to the aorta produces recirculation of systemic venous blood. Right-to-left shunts may be due to right-sided obstructive lesions or increases in PVR above SVR. The physiologic consequences are: (1) a decrease in PBF with admixture of deoxygenated blood to the systemic circulation producing hypoxemia and cyanosis, and (2) impedance to RV ejection resulting in ventricular pressure overload that ultimately can lead to RV dysfunction.
8. Calculation of QP:QS in the presence of a low FIO2 (<0.5) (in order to ignore the contribution of dissolved O2) can be simplified to the following equation: QP:QS = SaO2 − SSVCO2 / SPVO2 − SPAO2, where a = arterial, SVC = superior vena cava, PV = pulmonary vein (assumed to be 98% in absence of significant pulmonary disease), and PA = pulmonary artery.
Mixing Lesions
1. The majority of cyanotic defects are mixing lesions.
2. The intracardiac communication is so large that the two chambers or great vessels effectively become a common chamber (Table 18.2). Bidirectional shunting is present, and there is usually some degree of hypoxemia despite a normal or increased PBF. A parallel circulation exists in that a single ventricle contributes blood to both the systemic and pulmonary circulations.
3. Intercirculatory mixing is the unique situation that exists in transposition of the great vessels (TGA). Ventriculoarterial discordance in conjunction with AV concordance produces a parallel circulation, that is, blood flow will consist of parallel recirculation of pulmonary venous blood in the pulmonary circuit and systemic venous blood in the systemic circuit. Survival is dependent on one or more communications (atrial septal defect [ASD], PFO, VSD, patent ductus arteriosus [PDA]) between the parallel circuits to allow intercirculatory mixing.
4. The QP:QS is dependent on vascular resistance and outflow obstruction.
a. With no outflow obstruction, flow to the systemic or pulmonary circulation is dependent on the ratio of PVR to SVR. As PVR typically is lower than SVR, the predominant physiology is L → R shunting with increased PBF (QP:QS >1). If PVR exceeds SVR, the predominant physiology is R → L shunting with decreased PBF and hypoxemia (QP:QS <1).
b. Mixing with LV outflow obstruction results in excessive PBF and systemic hypoperfusion (predominantly L → R shunting with QP:QS >1).
c. Mixing with RV outflow obstruction varies from balanced flow to significantly decreased PBF and hypoxemia (predominantly R → L shunting with QP:QS <1).
5. Single ventricle hearts have mixing physiology.
CLINICAL PEARL Total systemic blood flow (QS) is the combination of effective systemic blood flow (blood from the pulmonary veins to the aorta) and any physiologic right-to-left shunt (blood from the systemic veins recirculated to the aorta).
Total pulmonary blood flow (QP) is the combination of effective pulmonary blood flow (blood from the systemic veins to the PA) and any physiologic left-to-right shunt (blood from the pulmonary veins recirculated to the PA).
The volumes of effective systemic blood flow (QS eff) and effective pulmonary blood flow (QP eff) are ALWAYS equal.
Obstructive Lesions
1. The physiology is dependent on the site (left- or right-sided), severity (mild to severe), and nature (fixed or dynamic) of the obstruction.
2. Obstruction may be valvar, subvalvar, supravalvar, or a combination thereof.
3. Valvular atresia represents the most extreme form of obstruction. With severe ventricular hypoplasia, because the ventricle does not contribute to the circulatory physiology, these lesions are better approached as single ventricle physiology.
4. Dynamic obstruction results from apposition of structures during ventricular systole such that the diameter of the outflow tract is narrowed. Lesions producing dynamic obstruction include hypertrophic cardiomyopathy and tetralogy of Fallot.
5. Critical neonatal left-sided obstruction is characterized by:
a. Ductal-dependent circulation with systemic perfusion dependent on blood flow from the RV and PA.
b. Systemic hypotension and hypoperfusion.
c. LV dysfunction.
d. Impaired coronary perfusion with retrograde flow from the descending aorta.
e. Systemic hypoxemia (desaturated blood from RV via PDA).
6. Critical neonatal right-sided obstruction is characterized by:
a. Ductal-dependent circulation with pulmonary perfusion dependent on blood flow from the LV and aorta.
b. Decreased PBF
c. Systemic hypoxemia
d. RV dysfunction.
7. Mild to moderate outflow tract obstruction manifests in infants and children with ventricular pressure overload, which can be well tolerated for many years.
Regurgitant Lesions
1. Isolated regurgitant valves, aside from Ebstein anomaly, are uncommon as primary congenital defects.
2. Regurgitant lesions lead to ventricular volume overload, with progression to ventricular dilation and failure. The time course over which irreversible ventricular dysfunction develops is variable, but if surgical intervention to correct the volume overload is undertaken within the first year of life, residual dysfunction is uncommon (69,70).
3. General goals of hemodynamic management for valvar regurgitation are:
a. Maintain increased HR—decreased diastolic time with aortic/pulmonary regurgitation reduces regurgitant fraction; with mitral/tricuspid regurgitation higher rates decrease ventricular volume and regurgitant fraction.
b. Maintain normal to increased preload.
c. Maintain contractile state of the ventricle.
d. Decrease afterload of the corresponding ventricle (PVR, SVR).
e. Maintenance of sinus rhythm may not be as important as with stenotic lesions.
PBF AND MANIPULATION OF PVR
1. Intracardiac shunting and alterations in PBF are a major characteristic of congenital cardiac defects. Lesions producing increased PBF (via L → R shunt or mixing with low PVR/LV outflow obstruction) include ASD, VSD, PDA, AV canal defects, anomalous pulmonary venous connection, truncus arteriosus, TGA, and single ventricle lesions. Lesions producing decreased PBF (via R → L shunt or mixing with RV outflow obstruction) include tetralogy of Fallot, pulmonary atresia, tricuspid atresia, single ventricle lesions, TGA with LV outflow obstruction, and severe Ebstein anomaly. Shunt-related arterial desaturation can occur when PBF is greater than, equal to, or less than systemic blood flow (71).
2. The PVR is an important determinant of shunt magnitude and direction with certain defects. As a consequence, manipulation of PVR allows some measure of control over the shunting (Table 18.4).
The anesthesiologist can easily control ventilation so that manipulation of PaO2, PaCO2, pH, and lung volumes are excellent methods for altering PVR independently of SVR. A combination of a high inspired FIO2, a PaO2 higher than 60 mm Hg, a PaCO2 of 30 to 35 mm Hg, a pH of 7.50 to 7.60, and low inspiratory pressures without high levels of PEEP, will produce reliable reductions in PVR. Conversely, a reduced FIO2, a PaO2 of 50 to 60 mm Hg, a PaCO2 of 45 to 55 mm Hg, and the application of PEEP, can be used to increase PVR.
There is no intravenous drug that selectively acts as a pulmonary vasodilator. Pulmonary vasodilators (PGE1, nitroglycerin, sodium nitroprusside, and milrinone) induce systemic vasodilation as well. Inhaled nitric oxide (NO) selectively produces pulmonary vasodilatation, and has been used to treat pulmonary hypertension in both the pre- and post-CPB period in a variety of congenital heart lesions and in treatment for persistent pulmonary hypertension of the newborn. Generally, approximately 20 to 40 parts per million inhaled NO has been used. Some caution is warranted because both NO dependency and rebound pulmonary hypertension following NO discontinuation have been reported. Use of NO is not warranted to treat pulmonary hypertension when there is a downstream mechanical obstruction to PBF. An alternative to inhaled NO is aerosolized prostacyclin (PGI2), its analogs (iloprost, epoprostenol) and PGE1. Sildenafil is a cGMP-specific phosphodiesterase (PDE5) inhibitor that by the oral, intravenous, and aerosolized routes is effective in reducing PVR.
3. The increased resistance and reactivity of the pulmonary vasculature in the neonate and some cardiac defects can result in an enhanced response to vasoconstricting influences. Unrestricted left-to-right shunting with increased PBF produces a volume load on the heart and structural changes (medial hypertrophy progressing to necrotizing arteritis) in the PV bed (PV occlusive disease (PVOD) (72,73). The end result is pulmonary hypertension (mean PA pressure >25 mm Hg) and a decrease in myocardial function. The time course for developing PVOD depends on the size and site of the shunt and age at surgery. Progression is more rapid when both the volume and pressure load on the pulmonary circulation is increased, such as with a large VSD. Pulmonary hypertension develops more slowly with increased PBF in the absence of elevated PA pressures, as with an ASD. For the majority of infants with an unrestrictive shunt, repair of the defect within the first year of life is usually associated with regression of the PV changes. Severe pulmonary hypertension (Eisenmenger physiology) imparts a major anesthetic risk, even for minor procedures (74–76).
TABLE 18.4 Manipulations altering PVR
Increased PVR |
Decreased PVR |
Hypoxia |
Inspired oxygen |
Hypercarbia |
Hypocarbia |
Acidosis |
Alkalosis |
Hyperinflation or atelectasis |
Normal lung volume (functional residual capacity) |
Sympathetic stimulation (pain, agitation) |
Blunting sympathetic stimulation (sedation, analgesia) |
Polycythemia |
Anemia |
Surgical constriction of PA |
Pulmonary vasodilator agents |
Modified from Odegard KC, DiNardo JA, Laussen PC. Anesthesia for congenital heart disease. In: Gregory GA, Andropoulos DB, eds. Gregory’s Pediatric Anesthesia. 5th ed. Oxford, UK: Wiley-Blackwell; 2012, with permission.
4. Cardiopulmonary bypass may produce elevations in PVR as a result of the whole body inflammatory response (including neutrophil sequestration, microemboli, platelet activation, and thromboxane A2 generation), decreased NO production, atelectasis, hypoxic pulmonary vasoconstriction, and adrenergic events (77–79).
MANIPULATION OF SVR
1. Manipulation of SVR and systemic arterial pressure is important in the following situations:
a. Complex shunt with fixed RV outflow obstruction and R → L shunting (e.g., tetralogy of Fallot). An increase in SVR will decrease the R → L shunting and increase arterial oxygen saturation (80).
b. Restrictive aortopulmonary shunt, for example, modified Blalock–Taussig shunt. An increase in SVR and systemic arterial pressure will increase flow across the shunt (PBF). Reducing PVR may further increase flow across the shunt.
c. Coronary ischemia
2. Maneuvers to increase SVR and systemic perfusion pressure:
a. Vasoconstrictor agents (alpha agonists) such as phenylephrine, norepinephrine, or vasopressin (refractory vasodilatation).
b. Fluid administration—crystalloid and/or 5% albumin, with packed red blood cells for low hematocrit.
c. Inotropic agents—dopamine is our first choice. Epinephrine is useful for short-term therapy when high systemic pressures are sought. Inotropic agents should be used with extreme caution in lesions with dynamic outflow obstruction.
SUMMARY OF PATHOPHYSIOLOGIC GOALS OF INTRAOPERATIVE HEMODYNAMIC MANAGEMENT
Cardiac output is the product of HR and SV, with SV determined by preload, contractility, and afterload. A practical approach to determining hemodynamic goals is to consider rate, rhythm, preload, contractility, and afterload (Table 18.5). Sinus rhythm is always preferable, particularly in settings of reduced ventricular compliance and single ventricle lesions where the atrial contribution to ventricular filling is very important. Failure to improve cardiac output after volume adjustments requires the use of an inotropic agent (81), commonly dopamine in doses of 3 to 10 µg/kg/minute.
MYOCARDIAL ISCHEMIA
Although abnormalities in the coronary circulation predispose to ischemia, in many congenital defects ischemia may occur secondary to imbalances in myocardial oxygen supply and demand with normal coronary arteries. Subendocardial perfusion is largely determined by coronary perfusion pressure (CPP), which is the aortic diastolic pressure minus the ventricular end-diastolic pressure. In addition, the time interval available for perfusion (predominately diastole) is critical. As a result, the relationship between diastolic blood pressure, ventricular end-diastolic pressure and HR will determine whether subendocardial ischemia occurs. These three factors place patients with CHD at risk of ischemia as follows:
TABLE 18.5 Intraoperative hemodynamic goals
1. Congenital lesions in which the systolic pressure in the ventricles is systemic, and in some cases the pulmonary ventricle may have suprasystemic pressures, perfusion of the ventricles is dependent on the rapid increase in early diastolic flow. In normal hearts, some subendocardial perfusion of the right ventricle can occur in systole as well as in diastole because RV systolic pressure is low.
2. Aortic diastolic pressure, which is normally low in neonates and infants, is compromised in ductal-dependent and single ventricle lesions because these lesions promote diastolic runoff of aortic blood into the lower resistance pulmonary circuit. Coronary perfusion is further compromised if the coronary ostia are perfused retrograde with desaturated blood down a segment of atretic ascending aorta (as in hypoplastic left heart syndrome [HLHS]).
3. Elevated ventricular end-diastolic pressure decreases CPP, and may be the result of impaired systolic function, impaired diastolic function (reduced ventricular compliance and relaxation), increased ventricular end-diastolic volume (volume overload), or a combination thereof. Ventricular volume overload occurs as a result of lesions with increased QP:QS, regurgitant valve lesions, and single ventricle lesions. Ventricular pressure overload occurs as a result of outflow obstruction, with the resultant hypertrophy impairing diastolic function.
4. Increases in HR geometrically reduce the duration of diastole (duration of systole stays relatively constant), so that the time available for coronary perfusion falls. Consequently, a higher diastolic pressure is necessary to maintain subendocardial perfusion at higher HRs. The combination of a high HR and low diastolic blood pressure can produce significant ischemia. For example, in an infant with truncus arteriosus an aortic diastolic pressure of 25 mm Hg and a HR of 130 to 140 beats per minute (bpm) may well be tolerated without evidence of subendocardial ischemia whereas it is unlikely that a HR of 170 to 180 bpm will be tolerated at the same diastolic pressure.
5. Coronary artery anomalies are discussed below.
PREOPERATIVE EVALUATION
PSYCHOLOGICAL CONSIDERATIONS
The pediatric patient has unique psychological concerns that depend on age and previous surgical and anesthetic experience (82). Complex CHD may have a profound impact on the patient’s psychosocial development such that the effect on parents and other family members cannot be minimized and must be addressed sensitively during the preoperative evaluation. The preoperative psychological preparation must be appropriate to the age of the patient, and although neonates require no psychological preparation, the perioperative process can overwhelm the parents. Fear of separation from parents (“stranger anxiety”) can be manifest in the infant at 8 to 12 months with an intense aversion to strangers. Very few toddlers, even if playful and cooperative in the presence of the parent, will leave their parent happily to go to the operating room. Older children have fears of disfigurement or that they will “wake up” or feel pain during and after surgery. Even the withdrawn, seemingly complacent adolescent often is in great internal turmoil regarding the impending surgery. Excess stress, as with adult cardiac patients, has the potential for adverse alterations in hemodynamic function. Psychological issues also influence how much detail is discussed in the presence of the patient when obtaining informed consent. Alternatively, weak promises of “no needles” or little discussion about what the child can expect may be counterproductive, especially if the child is to undergo future additional procedures.
HISTORY
The history should focus on the cardiopulmonary system and include medications, allergies, past hospitalizations, and operations (including cardiac catheterizations), and prior anesthetic experiences. General health and performance of age-appropriate activities will aid in the evaluation of cardiac function and reserve. Failure to gain weight (cardiac cachexia) implies significant disease and is often an indication for surgery. Low cardiac reserve in the infant will be reported by parents as poor feeding, sweating, tiring, dyspnea, and circumoral cyanosis during feeding. The observation by a parent that the child cannot keep the same pace as siblings is often a reliable indication that cyanosis or congestive heart failure (CHF) is significant. Frequent pulmonary infections may occur as a result of increased PBF in otherwise asymptomatic patients. Any intercurrent illness such as an upper respiratory tract infection or pneumonia may require delaying of surgery because of the negative impact on airway reactivity and PVR (83). Newly observed clinical signs should raise suspicion of the possible need for further or more recent diagnostic tests. The patient’s cardiologist is often the best source for the details of previous interventions and complications.
PHYSICAL EXAMINATION
Interpretation of vital signs is age specific, particularly with respect to HR, blood pressure, and respiratory rate. CHF will sequentially inhibit age-appropriate gains in weight, height, and head circumference. Interestingly, cyanotic children often do not manifest this failure to thrive. Physical examination may reveal cyanosis, pallor, clubbing, or signs of CHF similar to those seen in adults (tachypnea, use of accessory respiratory muscles, hepatomegaly, ascites, and edema). Rales may not be heard in infants and children with CHF. Cool peripheries and skin mottling (often only evident with crying) suggests significantly reduced cardiac reserve. Differentiation between CHF and an upper respiratory tract infection can sometimes be very difficult. Both conditions may show tachypnea, wheezing and upper airway congestion. A history of infection in other family members and a raised white cell count may help distinguish between the two. Proceeding to cardiac surgery may be necessary even when the differentiation between worsening failure and a respiratory tract infection cannot be made with certainty.
Physical examination should include an evaluation of the limitations to vascular access and monitoring sites. A child who has undergone a previous palliative shunt procedure (classic Blalock–Taussig shunt) or repair of coarctation of the aorta may have a diminished pulse or unobtainable blood pressure in the arm in which the subclavian artery has been used. This obviously has implications for blood pressure monitoring and arterial catheter placement. Finally, the child who has undergone multiple palliative procedures may have poor venous access, which can influence the mode of induction. As extracardiac anomalies occur in 25% of infants seen during the first year of life for significant cardiac disease, a focused airway examination and evaluation of other organ systems is essential.
LABORATORY DATA
The hematocrit is elevated in direct relation to the degree of hypoxemia with cyanotic heart disease due to secondary erythrocytosis. Phlebotomy and exchange transfusion should only be considered to improve hemostasis or to treat hyperviscosity symptoms in the presence of a hematocrit >65%. Iron deficiency, common in patients with cyanotic CHD, is associated with symptoms similar to those of hyperviscosity and must be ruled out prior to phlebotomy or exchange transfusion (84). Cyanosis affects the coagulation process, and it is not unusual to observe erythrocytosis and/or a functional platelet defect and prolongation of the prothrombin, partial thromboplastin, and bleeding times. In the face of reoperation with extensive dissection, the anesthesiologist should plan adequate blood component therapy for each patient. Patients with severe cyanosis and anemia may require transfusion at higher levels of hematocrit than are typically considered.
Plasma electrolyte concentrations should be screened in patients receiving diuretics or digitalis. Hypocalcemia may be observed in infants with DiGeorge syndrome. Although severe CHF may be accompanied by jitteriness and irritability, it is important to rule out hypoglycemia as a potential cause.
The chest radiograph serves to confirm other clinical and diagnostic findings, and provides information on heart size, degree of PBF, situs of the aortic arch, pulmonary disease (hypoplasia, pneumonia, atelectasis, hyperinflation, pleural effusion), and previous surgical procedures. Left-to-right shunt lesions typically have increased pulmonary arterial markings, whereas cyanotic lesions with decreased PBF typically have dark, underperfused lung field.
The electrocardiogram demonstrates rate, rhythm, conduction abnormalities, chamber enlargement, cardiac malpositions, ventricular strain, ischemia, and severe electrolyte abnormalities. A patient may have had a 24-hour Holter or loop recorder if there were symptoms suggestive of an arrhythmia.
Two-dimensional echocardiography has revolutionized the field of pediatric cardiology over the last decade. Echocardiography is a noninvasive means for assessment of intracardiac anatomy, blood flow patterns, and estimates of ventricular function and physiologic status. Unlike adults who may have limited transthoracic echocardiographic windows, images in pediatric patients are easily obtained and are critical to a clear understanding of the pathophysiology and surgical plan. Many patients proceed to surgery without catheterization studies. Three-dimensional echocardiography and cardiac magnetic resonance imaging (MRI) have become important imaging modalities. Cardiac MRI is particularly useful for vascular anatomy and pulmonary regurgitation with RV function assessment (85).
Cardiac catheterization data should be reviewed for:
1. Anatomic diagnosis.
2. Interpretation of oxygen saturations—saturation step-ups or step-downs between vessels and chambers are used to detect shunts, saturation data are used to calculate the ratio of pulmonary to systemic blood flow (QP:QS), and can be used to differentiate intrapulmonary shunting and V/Q mismatch (atelectasis, hypoventilation, pulmonary disease) from intracardiac shunting.
3. Interpretation of pressure data—pressures are used to compare right and left heart function (particularly ventricular end-diastolic pressure), systemic and pulmonary arterial pressures are measured, as are pressure gradients across valve and shunt orifices.
4. Interpretation of angiographic data—to assess ventricular wall motion (systolic function), intracardiac and great vessel blood flow patterns, coronary anatomy, and aortopulmonary collateral anatomy.
5. Anatomy, location, and function of previously created shunts or conduits. This information will allow for intelligent decisions regarding monitor placement and anesthetic management.
6. Effect of interventions—interventions in the catheterization laboratory to palliate congenital heart lesions, including balloon valvuloplasty, balloon septostomy, balloon angioplasty, conduit dilation, and device placement are common. The effects of these interventions on the underlying lesion must be assessed. The reversible component of PA hypertension may be assessed by the response to 100% inspired oxygen or NO.
PREOPERATIVE PREPARATION
PREOPERATIVE FLUID THERAPY
Preoperative fasting follows standard American Society of Anesthesiologists (ASA) guidelines, that is, 2 hours for clear liquids, 4 hours for breast milk, and 6 to 8 hours for infant formula, nonhuman milk, and solids (86). As dehydration may have deleterious effects on hemodynamic function (particularly intraoperatively) or on blood viscosity (cyanotic lesions), in the absence of intravenous hydration young children should remain NPO for the shortest possible interval. Intravenous maintenance fluids before surgery should be considered in certain settings, for example patients with severe polycythemia or Fontan circulations. In patients receiving nutritional support as part of medical stabilization prior to surgery, high-calorie total parenteral nutrition and intralipid therapy should be discontinued and replaced with an appropriate dextrose infusion prior to transport to the operating room. Dextrose infusions should be discontinued prior to commencement of cardiopulmonary bypass (CPB) as CPB generally produces mild hyperglycemia.
PREOPERATIVE MEDICATIONS
The goal of premedication is to achieve a sedated, calm patient who has adequate ventilation and circulation. Premedication facilitates separation of the young child from the parents, alleviates anxiety prior to induction, greatly facilitates placement of an intravenous catheter when an inhalational induction is undesirable, and in the sicker patients reduces myocardial oxygen consumption and PVR. One must always consider how stressful it is to parents to see their child being taken to the operating room crying or upset. Premedication should always be administered and assessed in the continuous presence of the anesthesiologist, especially with substantial dosages in younger infants and/or those with airway abnormalities.
In general, infants younger than 6 months do not require premedication for separation, but some sedation does reduce the level of distress (particularly for the operating room staff) during intravenous catheter placement. Oral midazolam 0.5 to 0.75 mg per kg (maximum dose 20 mg) in infants and younger children who have not had prior cardiac surgery is usually very effective. Midazolam alone may be insufficient for patients who have undergone previous operative procedures and long intensive care admissions. These patients may be remarkably tolerant to sedative agents and can also have heightened anxiety. In these situations, oral ketamine (3 to 5 mg/kg for infants, 3 to 10 mg/kg for children) can be given in conjunction with oral midazolam (1 mg/kg). The intramuscular route can be used when the child refuses to take oral medication, particularly with the desire to avoid a traumatic mask induction or for placement of an intravenous catheter. Ketamine 3 to 5 mg per kg alone or in combination with midazolam 0.05 to 0.1 mg per kg seldom fails. Older children may choose placement of an IV and intravenous sedation, usually midazolam. Although some authors recommend clonidine premedication (4 µg/kg combined with atropine 0.02 mg/kg) via the oral or rectal route as an alternative to midazolam, data in children with CHD is lacking (87). In patients with known paradoxical responses to benzodiazepines, agents such as pentobarbital 2 to 4 mg per kg may be considered (88). Current induction techniques do not justify routine administration of atropine or glycopyrrolate as a premedicant.
Digoxin, diuretics, and ACE inhibitors are generally withheld during the morning of surgery. ACE inhibitors exacerbate hypotension occurring with anesthetic induction. Inotrope and prostaglandin infusions are continued into the operative period for critically ill neonates and children.
OPERATING ROOM PREPARATION
Meticulous preparation of the operating room prior to bringing in the patient is fundamental. In addition to the standard room setup for the pediatric patient, the anesthesia machine should have the capacity to provide air (low FIO2) to help balance pulmonary and systemic blood flow. Intravenous tubing should be free of air bubbles to reduce the potential for air embolism in all patients with shunts (not just those with right-to-left shunts). Air filters trap bubbles, but severely limit the rate of fluid administration. Resuscitative drugs, with age-appropriate dilutions, should be drawn up prior to induction and be ready for immediate administration (Table 18.6). These include atropine, epinephrine, ephedrine, calcium (gluconate or chloride), phenylephrine, and succinylcholine. For critically ill patients and patients with marginal reserve, selected inotrope infusions and antiarrhythmic agents should be prepared in anticipation of their use (Table 18.7).
TABLE 18.6 Vasoactive agents
Agent |
Dosage (intravenous) |
Calcium chloride |
10–20 mg/kg |
Calcium gluconate |
30–60 mg/kg |
Epinephrine |
0.05–0.5 µg/kg/min |
Norepinephrine |
0.05–0.5 µg/kg/min |
Phenylephrine |
0.1–0.5 µg/kg/min |
Vasopressin |
0.0003–0.002 µg/kg/min |
Isoproteronol |
0.01–0.05 µg/kg/min |
Dobutamine |
5–10 µg/kg/min |
Dopamine |
3–15 µg/kg/min |
Milrinone |
Bolus 50 µg/kg then 0.3–1.0 µg/kg/min |
Nitroglycerin |
0.5–5 µg/kg/min |
Nitroprusside |
0.5–5 µg/kg/min |
Fenoldopam |
0.1–0.5 µg/kg/min |
PGE1 |
Initiate at 0.05–0.1 µg/kg/min then 0.01–0.05 µg/kg/min |
From DiNardo JA, Zvara DA. Anesthesia for Cardiac Surgery. 3rd ed. Malden, MA: Blackwell Science; 2008, with permission.
TABLE 18.7 Antiarrhythmic agents
Agent |
Dosage (intravenous) |
Lidocaine |
Bolus 1 mg/kg then 20–50 µg/kg/min |
Procainamide |
Bolus 2–5 mg/kg then 20–50 µg/kg/min |
Diltiazem |
Bolus 0.1–0.2 mg/kg then 1–3 µg/kg/min |
Amiodarone |
Slow bolus of 2–5 mg/kg with extreme caution in neonates/infants then 7 µg/kg/min |
Esmolol |
Bolus 300–500 µg/kg then 50–300 µg/kg/min |
Adenosine |
0.05–0.2 mg/kg |
Magnesium |
10–25 mg/kg |
From DiNardo JA, Zvara DA. Anesthesia for Cardiac Surgery. 3rd ed. Malden, MA: Blackwell Science; 2008, with permission.
INTRAOPERATIVE MANAGEMENT
MONITORING
If the infant or child is awake or minimally sedated, application of multiple monitors may foil a smooth induction, even for an initially calm patient. Minimizing stimulation, including distracting discussions in the operating room, will avoid disturbing the child. Ideally, preinduction monitoring should include a noninvasive automated blood pressure cuff, ECG, and pulse oximeter, with the end-tidal carbon dioxide monitor ready for immediate use. In some cases, a pulse oximeter may be all that is practical in the early stages of induction, with other monitors added quickly as induction progresses.
1. ECG and Blood Pressure. A 5-lead ECG with leads II and V5 displayed allows rhythm and ischemia monitoring. Blood pressure monitoring consists of an upper or lower extremity noninvasive cuff as well as an intra-arterial catheter. An indwelling intra-arterial catheter is placed following induction and percutaneous access can usually be accomplished in even the smallest neonates. Cannulation of the radial artery is preferred, with a 24-gauge catheter for neonates and infants less than about 5 kg, a 22-gauge in young children, and a 20-gauge in adolescents. The femoral artery is selected if radial cannulation is not possible. After hypothermic CPB posterior tibial and dorsalis pedis arterial catheters function poorly and do not reflect central aortic pressure. Umbilical artery catheters may be used intraoperatively but are generally replaced with a peripheral arterial catheter and removed postoperatively. Consideration is given to any previous or proposed surgical procedure which may compromise the reliability of ipsilateral upper extremity intra-arterial pressure monitoring, such as a Blalock–Taussig shunt, modified Blalock–Taussig shunt, subclavian artery flap for repair of aortic coarctation, or sacrifice of an aberrant subclavian artery. Arterial cutdown should be considered if access is poor. Previous catheterization procedures, particularly those of an interventional nature, may result in femoral artery (or vein) occlusion. Brachial arteries are not used given the high risk of distal limb ischemia.
2. Pulse Oximetry. Oxygen saturation (SaO2) monitoring is accomplished with pulse oximeter probes on both an upper and a lower extremity; these can be placed in pre- and postductal locations if the physiology warrants. Pulse oximetry is useful for balancing shunt flow and providing data about surgically created shunts and PA bands.
3. Capnography. End-tidal carbon dioxide (ETCO2) monitoring is routinely employed with the caveat that the difference between PaCO2 and ETCO2 will vary as physiologic dead space varies and that in some circumstances the difference may be large (>10 to 15 mm Hg). Any acute reduction in PBF (decreased cardiac output, pulmonary embolus, increased physiologic intracardiac R–L shunting) will increase this gradient. In patients with high QP:QS prior to initiation of CPB, the right PA may be partially or completely occluded by the surgeon to mechanically limit PBF. This maneuver dramatically increases physiologic dead space and ETCO2 will vastly underestimate PaCO2.
4. Central venous pressure. Percutaneous central venous access can be reliably obtained, even in small neonates, and provides central venous pressure monitoring, reliable intravenous access for drug and fluid administration, and sampling of blood for mixed venous oxygen saturation. The use of real-time ultrasonography has helped improve the success rate of placement in neonates and infants (89,90). For neonates and patients <10 kg a 4-French, 5-cm double lumen catheter is suitable, while a 5-French, 5-cm double lumen catheter is suitable for young children >10 kg. Six- to nine-French catheters are available for use in older children and adults. A longer catheter should be used for femoral venous cannulation to avoid dislodgement, particularly in chubby infants and toddlers.
Central venous access is not without risks, particularly in neonates and infants. Risks include pneumothorax, carotid or subclavian artery puncture, thrombosis, air embolus, infection, and thoracic duct injury (left side). The risk of internal jugular or SVC thrombosis must be considered, as this complication can be devastating in patients being staged to a total cavopulmonary connection. Thrombosis and infection risk can be reduced by maintaining a heparin infusion (10 µ/kg/hour for internal jugular or femoral lines; 1 to 2 µ/hour for intracardiac lines) through the central line lumens postoperatively and by removing the lines as soon as possible (typically 1 to 2 days).
In some institutions neonates and infants are managed prior to CPB without central venous lines. Transthoracic intracardiac lines (RA, LA, PA) placed by the surgeon prior to termination of CPB can be used for pressure monitoring, infusion of vasoactive and inotropic agents, and blood product and volume replacement (in infants and young children when peripheral access is limited). Preoperative use of PA catheters for pediatric patients is extremely uncommon. The surgeon can place a transthoracic PA catheter when postoperative assessment of PA pressure is important.
5. Temperature. As cerebral and myocardial protection are maintained principally through hypothermia, temperature is monitored at three sites for all bypass cases—rectal, esophageal, and tympanic membrane (nasopharyngeal in some institutions). Rectal temperature is considered a peripheral temperature site and equilibration of rectal with tympanic and esophageal temperatures functions as the best index of homogenous somatic cooling and rewarming. Rectal temperature lags behind esophageal and tympanic membrane temperature during both cooling and rewarming. Esophageal and tympanic membrane are core temperature monitoring sites and changes at these sites generally mirror brain temperature changes. Even so, esophageal and tympanic membrane temperatures may under- or overestimate brain temperature by as much as 5°C during cooling and rewarming. This observation underscores the importance of providing an adequately long period of core cooling prior to commencement of low-flow CPB or DHCA.
6. Near Infra-Red Spectroscopy (NIRS). NIRS is still an evolving technology of a noninvasive, real-time, online monitor of cerebral oxygenation (91). NIRS technology is particularly suited to analysis of cerebral tissue oxygenation because wavelengths of light in the near infra-red range (650 to 900 nm) are capable of penetrating the scalp, skull, and cranial contents and because the peak absorption spectra of the chromophores reflecting tissue oxygenation, namely oxyhemoglobin (HbO2), deoxyhemoglobin (HHb), and oxidized cytochrome aa3 (CytOx), reside in this wavelength range. Cerebral oxygen saturation measured by NIRS is the combined oxygen saturation of an uncertain mix of arterioles, capillaries, and venules. Clinical use has expanded greatly in recent years, with current commercially available devices using continuous wave reflectance technology to measure cerebral and somatic tissue oxygen saturation. Devices marketed for clinical use include the INVOS 5100C (Covidien, Boulder, CO), FORE-SIGHT (Casmed, Branford, CT), EQUANOX 7600 (Nonin Medical, Plymouth, MN), and outside the USA the NIRO-200NX (Hamamatsu Photonics, Hamamatsu City, Japan). It is important to be aware that although all devices can detect decreases in cerebral oxygenation, in healthy people there is significant variability in baseline readings, both between subjects and between instruments (92). Normal (baseline) values are particularly variable in children with CHD, and critical values for cerebral oxygenation in humans have yet to be determined (93).
7. Transcranial Doppler (TCD) Sonography. TCD is a noninvasive technology that allows continuous measurement of cerebral blood flow velocity and detection of cerebral microemboli. It is ideally suited for use in neonates and infants as the thin skull combined with a low frequency (2 MHz) ultrasonic transducer allows transmission of ultrasonic energy into brain tissue with little signal attenuation. Placement of the probe over the temporal bone allows the ultrasound beam to be aligned fairly parallel with the proximal (M1) segment of the middle cerebral artery (MCA), and the ability of pulsed-wave Doppler to be gated to a specific depth allows determination of blood flow velocity in the MCA just distal to the takeoff of the anterior cerebral artery. The Doppler interrogation yields a velocity spectrum of the insonated artery for each cardiac cycle, the outline of which resembles an arterial waveform. As the diameter of the MCA is relatively constant, flow velocity should approximate cerebral blood flow (integration of the area under the velocity spectrum yields the time-velocity integral [TVI] in units of cm/cardiac cycle, and the product of vessel TVI and vessel cross-sectional area [units of cm2] is flow [units of cm3/cardiac cycle]). Methodology and normal values for pediatric patients have been described (94). TCD determination of cerebral blood flow can be useful as a continuous surveillance monitor for detection of inadequate flow or obstructed cerebral venous drainage during CPB (95). Cerebral microemboli produce transient increases in reflected Doppler energy (high intensity transient signals, HITS) compared to the background Doppler spectra. At present, cerebral emboli detection with TCD requires the constant attention of a skilled observer because TCD technology alone lacks sufficient sensitivity and specificity to distinguish between gaseous brain emboli, particulate brain emboli, and artifacts. Difficulty in maintaining a constant probe position without causing skin injury and operator expertise are significant barriers to its routine use as an intra- or postoperative monitor in children.
8. Electroencephalography. The standard raw EEG is impractical for intraoperative use so that devices using processed EEG technology are utilized. The real-time unprocessed EEG waveform and the suppression ratio of the BIS monitor can be helpful in identifying EEG burst suppression or electrical silence. The effect of hypothermia on the EEG varies between individuals and the reliability of the BIS in infants has been questioned (96). We have not found the BIS as a depth of anesthesia monitor to be useful in infant cardiac surgery (97). A 4-channel quantitative EEG has been used in multimodality neuromonitoring during pediatric cardiac surgery, and EEG monitoring is best combined with other modalities of neurologic monitoring (98,99).
9. Transesophageal echocardiography (TEE). Intraoperative TEE is useful for delineation of complex anatomy that cannot be completely defined with transthoracic echocardiography, for hemodynamic assessment, and for postrepair decision making. In the subset of patients undergoing valve repair and outflow tract reconstruction TEE provides the best immediate assessment of the adequacy of the operative procedure and if necessary directs its revision. TEE is used selectively (approximately 30% to 40% of CPB cases) in some institutions and routinely in others. Multiplane 7.5 MHz probes are available for use in neonates and infants (down to 2.5 to 3.5 kg). Particular caution must be exercised in the smallest patients as the probe in the esophagus may cause tracheal and bronchial compression with compromise of ventilation, inadvertent tracheal extubation, right main stem bronchial intubation, esophageal perforation, aortic arch compression with loss of distal perfusion, and compression of the LA resulting in left atrial hypertension or compromise of ventricular filling. While detection of retained intracardiac air is certainly facilitated by use of intraoperative TEE it remains to be determined what role the technology will play in improving cardiac de-airing algorithms in pediatric patients.
AIRWAY MANAGEMENT
Airway management is fundamental to the anesthetic care of every patient. Prompt control of the airway and of ventilation is essential in the patient with CHD and reduced cardiopulmonary reserve. PVR is affected by pH, PaCO2, alveolar PO2, and lung volume, and as discussed above, changes in PVR may dramatically affect shunt magnitude and direction as well as cardiovascular function and stability.
Children with high PBF and interstitial pulmonary edema have surprisingly poor lung compliance and increased airway resistance (67,100). Decreased dynamic compliance will necessitate higher than expected airway pressures, so that care must be taken not to insufflate the stomach during mask ventilation.
Nasal endotracheal tubes are generally utilized in infants and young children as they provide better stability intra- and postoperatively. In patients with cyanosis, high venous pressure, and/or receiving anticoagulants, such as those with a bidirectional Glenn shunt or Fontan, nasal endotracheal tubes must be passed cautiously as substantial nasal bleeding can be initiated. De-nitrogenation (preoxygenation) of the lungs is recommended prior to induction in all patients including those in whom high inspired O2 concentrations might temporarily reduce PVR and compromise systemic perfusion (101). With cyanotic lesions and arterial oxygen saturation in the 70% to 90% range, loss of the airway can result in rapid and profound desaturation (steep portion of the Hb–O2 dissociation curve). Pressure-control ventilation is generally used to avoid inadvertent barotrauma. This mode of ventilation requires vigilance as changes in chest wall or lung compliance will lead to instantaneous changes in tidal volumes. For example, when the chest cavity is opened it is usually necessary to decrease the ventilatory pressure to avoid hyperventilation and obstruction of the surgical field by the lungs Cuffed endotracheal tubes are generally utilized because poor pulmonary compliance secondary to lung water, chest wall edema, or abdominal distention can compromise minute ventilation. Cuff inflation will allow for higher airway pressures to be utilized if necessary and likely reduce the need to change out the endotracheal tube because of excessive leak.
INDUCTION OF ANESTHESIA
A variety of induction techniques provide hemodynamic stability and improved arterial oxygen saturation in patients with cyanotic heart disease. No single induction technique is suitable for all patients with CHD. The patient’s cardiopulmonary function, age, psychological development, and emotional state all play a role in the choice. An intravenous induction allows prompt airway control and clearly affords the greatest flexibility in terms of drug selection and drug titration. Intravenous induction is the preferred technique in newborns, with severely impaired ventricular systolic function, and systemic or suprasystemic PA pressures.
Inhalational (mask) induction can be accomplished safely in the subset of children without severe cardiorespiratory compromise. However, reduced PBF in cyanotic lesions will prolong the length of induction and the interval during which the airway is only partially controlled. In addition, even short intervals of airway obstruction or hypoventilation in these patients can result in hypoxemia. Sevoflurane is the inhalation induction agent of choice, with sevoflurane causing less myocardial depression, hypotension, and bradycardia than halothane. Isoflurane and particularly desflurane are unsuitable agents as their pungency causes copious secretions, airway irritation, and laryngospasm.
Sevoflurane, halothane, isoflurane, and fentanyl/midazolam in 1 or 1.5 MAC concentrations do not change pulmonary to systemic blood flow ratio in children with isolated atrial and VSDs when cautiously administered with 100% oxygen (102). LV systolic function was mildly depressed by isoflurane and sevoflurane at 1.5 MAC and depressed by halothane at 1 and 1.5 MAC.
Intravenous induction with high-dose synthetic narcotics (fentanyl 25 µg/kg) in combination with pancuronium (0.1 mg/kg) is commonly used in neonates and infants. The vagolytic and sympathomimetic effects of pancuronium counteract the vagotonic effect of synthetic opioids. In patients with a low aortic diastolic blood pressure and a high baseline HR, rocuronium (0.6 mg/kg), vecuronium (0.1 mg/kg) or cisatracurium (0.2 mg/kg) may be used to avoid increasing HR. In older children with mild to moderately depressed systolic function lower doses of a synthetic opioid can be used in combination with etomidate (0.1 to 0.3 mg/kg).
Ketamine (1 to 2 mg/kg) is a useful induction agent. Ketamine causes minimal increases in PA pressure in patients with normal or elevated baseline PVR provided the airway and ventilation are supported. The tachycardia and increase in SVR induced by ketamine makes it unfavorable in the setting of systemic outflow tract obstruction, but very useful when PBF is dependent on a systemic-to-PA shunt.
The myocardial depressant and vasodilatory effects of propofol and thiopental make them unsuitable as induction agents when cardiovascular function is moderately to severely decreased.
An alternative to an intravenous induction in patients with difficult intravenous access is an intramuscular induction with ketamine (3 to 5 mg/kg), succinylcholine (3 to 4 mg/kg), and atropine (0.02 mg/kg but not <0.1 mg). Atropine is necessary to prevent the bradycardia that may accompany succinylcholine administration. This technique provides for a hemodynamically stable induction with prompt airway control and tracheal intubation. It is suitable for neonates and small infants with CHF and excessive PBF or infants with aortopulmonary shunts where it is anticipated that initial intravenous access will have to be obtained via the internal or external jugular vein or the femoral vein. This technique is hampered by the short duration of action of succinylcholine with limitation of the period of patient immobility. An alternative technique combines ketamine (3 to 5 mg/kg) and rocuronium (1.0 mg/kg), but is hampered by the slightly longer time interval until attainment of adequate intubating conditions and the longer duration of action of rocuronium.
MAINTENANCE OF ANESTHESIA
Anesthesia is generally maintained using a synthetic opioid-based technique, either with high doses (25 to 100 µg/kg fentanyl or 2.5 to 10 µg/kg sufentanil) or low to moderate doses (5 to 25 µg/kg fentanyl or 0.5 to 2.5 µg/kg sufentanil). Opioids are used in combination with an inhalation agent (generally isoflurane 0.2% to 1.0% or sevoflurane 0.5% to 2.0%) or a benzodiazepine (generally midazolam 0.05 to 0.1 mg/kg) as tolerated. The combination of narcotics and benzodiazepines is synergistic in reducing SVR. The high-dose technique is particularly useful in neonates and infants. However, considering the limited contractile reserve in the immature myocardium, it is not surprising that the myocardial depressant and systemic vasodilatory effects of inhalation agents and the synergistic vasodilatory effects of benzodiazepines and opioids may be poorly tolerated in this patient group. Neonates and infants often have significant ventricular pressure and/or volume overload, and in many of these patients subendocardial and systemic perfusion is tenuous as a result of runoff into the pulmonary circulation and the associated low aortic diastolic blood pressure.
There are unique considerations for maintenance of anesthesia during CPB. Light anesthesia, particularly during cooling and rewarming, may lead to elevated SVR requiring a reduction in pump flow rate which compromises both systemic perfusion and the efficiency of CPB cooling and rewarming. Subclinical shivering due to inadequate neuromuscular blockade and light anesthesia are avoidable causes of increased systemic oxygen consumption. Increased systemic oxygen consumption during CPB will manifest as a lower than acceptable venous saturation (<65%) at what should be an adequate CPB flow rate for the patient. Furthermore, for a membrane oxygenator at or near its maximum flow capacity, a low venous saturation may result in a lower than acceptable arterial saturation.
Lower doses of opioid in conjunction with an inhalation agent or benzodiazepine are suitable for older patients with better cardiovascular reserve. In fact, carefully selected patients (>1 year old, no pulmonary hypertension, benign medical history) undergoing simple ASD or VSD closure are candidates for immediate tracheal extubation in the operating room or in the intensive care unit within 2 to 3 hours.
DISORDER: Malpositions
BACKGROUND
The heart may be abnormally located within the chest (left-sided (normal), right-sided, or midline) and/or have abnormal orientation of its base-to-apex axis (levocardia (normal), dextrocardia, mesocardia) (103). The heart may be displaced outside the thorax (ectopia cordis) or be abnormally located relative to the situs of the abdominal viscera (solitus (normal), inversus (mirror-image), or ambiguous/indeterminate). Cardiac malpositions may also be secondary to noncardiac malformations, such as diaphragmatic hernia, lung hypoplasia, scoliosis, or chest wall deformities. Primary cardiac malpositions are associated with a high incidence of complex cardiac anomalies.
EMBRYOLOGY/ANATOMY
1. Disturbance in the direction of looping of the tubular heart and left–right patterning by genes expressed during gastrulation.
2. Geva describes the necessary steps to determining the patient’s anatomy (104):
i. Determine the situs of the thoracoabdominal organs (solitus, inversus, or ambiguous)
ii. Determine the heart position and orientation (levocardia, dextrocardia, or mesocardia) within the chest
iii. Analysis of cardiac segmental anatomy from the venous entry to the arterial exit
iv. Atrial situs (solitus, inversus, or ambiguous)
v. Ventricular loop (D-loop, L-loop)
vi. AV alignments/connections (concordant, discordant, AV valve atresia, straddling, double inlet)
vii. Ventriculoarterial alignments (concordant, discordant (transposition of great arteries), double outlet, single outlet)
viii. Conal (infundibular) anatomy (subpulmonary, subaortic, bilateral, bilaterally absent)
ix. Relationship between semilunar valves (solitus, inversus, D-malposition, L-malposition, anterior malposition)
x. Associated anomalies
3. Levocardia, dextrocardia, and mesocardia are usually used to describe both cardiac position and orientation. Levocardia is the normal position of the heart (left-sided) with a leftward apex; in dextrocardia the heart is usually right sided with a rightward apex, and in mesocardia the heart is midline with the apex typically pointing anteriorly or inferiorly (Fig. 18.14). Each may be associated with normally located abdominal viscera, inversely located atria and abdominal viscera, or visceral heterotaxy (Fig. 18.15).
FIGURE 18.14 Cardiac position within the thorax. In levocardia, the heart is predominantly in the left hemithorax. In dextrocardia, the heart is predominantly in the right hemithorax. In mesocardia, the heart is midline and the apex typically points anteriorly and inferiorly. The orientation of the apex should be explicitly described because the position of the heart within the thorax and the orientation of the apex may not be concordant (e.g., dextrocardia with a leftward pointing apex). (Reproduced with permission from Geva T. Nomenclature and segmental approach to congenital heart disease. In: Lai WW, Mertens LL, Cohen MS, et al, eds. Echocardiography in Pediatric and Congenital Heart Disease: From Fetus to Adult. Oxford, UK: Wiley-Blackwell; 2009:22–33 (Figure 3.4, p. 25).)
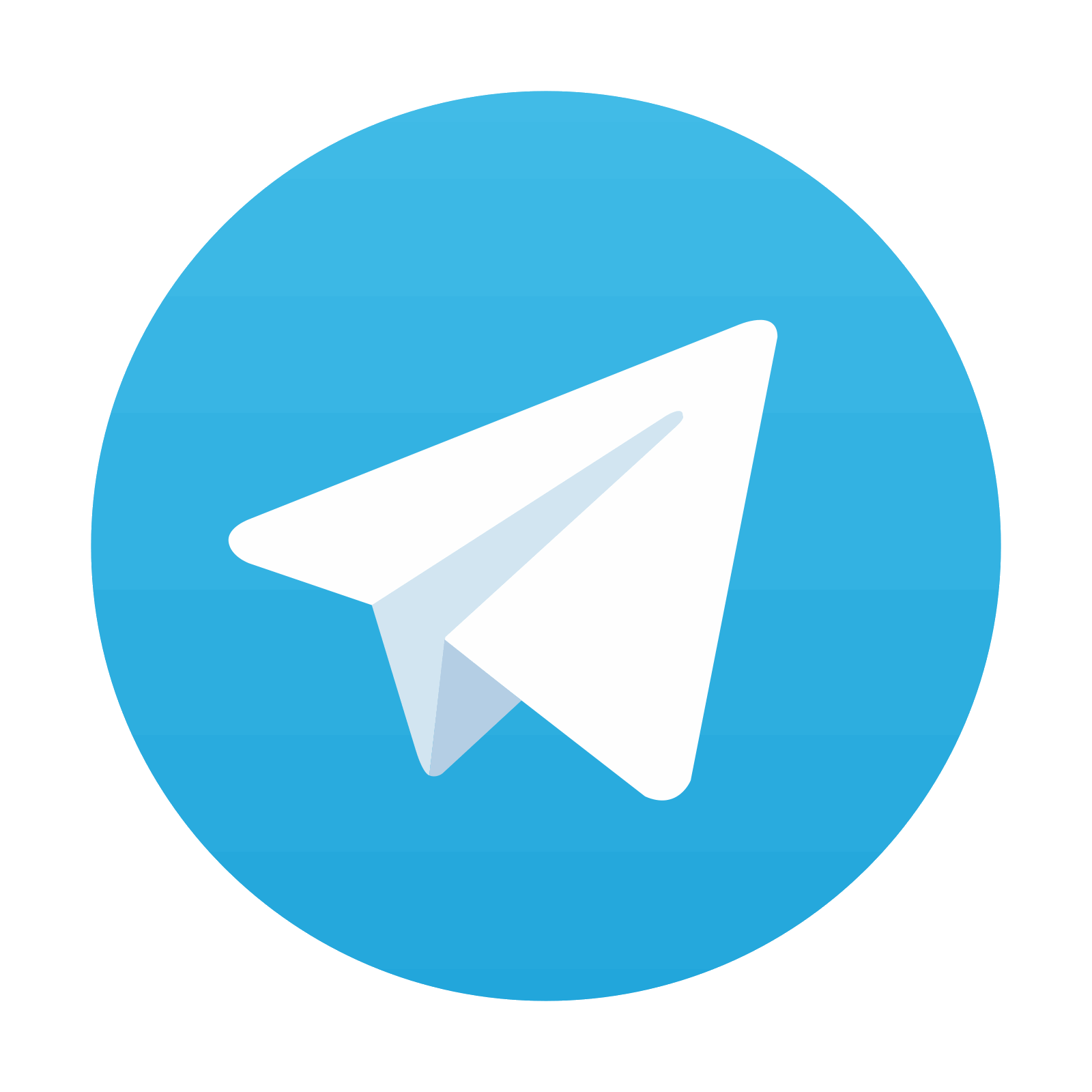
Stay updated, free articles. Join our Telegram channel
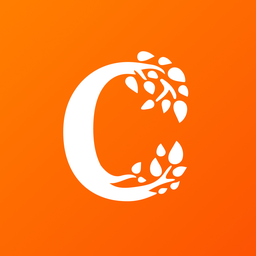
Full access? Get Clinical Tree
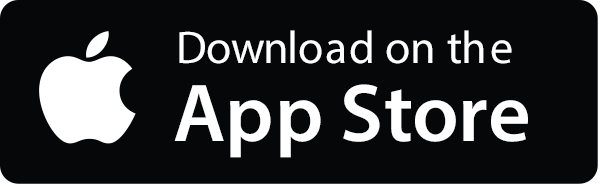
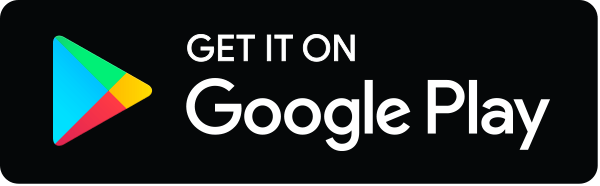