The Biology of Lung and Esophageal Cancer
Mark W. Onaitis
David H. Harpole
Over the past decades, it has become recognized that cancer is a genetic disease. Therefore, the biology of lung and esophageal cancer must be considered from the perspective of genetic changes in the epithelial cells of these organs. In this chapter, we will highlight many of these known individual genetic changes as well as groups of genetic changes.
ONCOGENES
Oncogenes are genes that lead to increased proliferative capacity of cells. Because they are activating mutations, mutation or overexpression of only one of two normal protooncogenes per cell is required for transformation. Oncogenes may lead to increased proliferation through a variety of mechanisms, including augmented growth rate, increased mitotic rate, and decreased rate of apoptosis.
Ras
Ras is perhaps the best-known oncogene. The Ras family of proteins consists of three members, HRas, NRas, and KRas, the last of which is implicated in 30% to 40% of human lung adenocarcinoma.1 Wild-type Ras is a membrane-associated G protein that serves as a link between tyrosine kinase receptors at the membrane and cytoplasmic second messenger molecules. These second messenger molecules participate in a proliferative signaling cascade that activates mitogen-activated protein kinase (MAPK) resulting in increased proliferation. This proliferation is caused by mutations in KRas codons 12, 13, and 61, all of which ablate KRas’ GTPase activity and constitutively activate KRas.
In both individual studies and a meta-analysis, the presence of mutated KRas in resected lung cancer portends a poor prognosis.2–4 Although the presence of these mutations may identify aggressive tumors, a portion of the prognostic impact may be explained by poor response to adjuvant chemotherapy. In the National Cancer Institute of Canada Clinical Trials Group (NCIC-CTG) North American intergroup study, the JBR.10 trial, the subgroup of patients with Ras mutations did not experience a survival advantage.5 Because localization of Ras to the membrane is dependent upon farnesylation, farnesyltransferase inhibitors may be more effective in patients with Ras mutation.
In addition to mutation of Ras, control of normal Ras protein levels may be important in lung cancer tumorigenesis. Recently, Ras was found to be an important target of the let-7 microRNA family. MicroRNAs are 22 nucleotide RNA molecules that negatively regulate gene expression by silencing messenger RNAs or by targeting microRNAs for destruction. Lung tumors exhibit lower levels of let-7 than normal lung,6 and low expression levels of let-7 have been demonstrated as predictive of poor survival.7–9
Interestingly, oncogenic Ras mutations occur almost exclusively in adenocarcinomas of smokers.10,11 This may relate to the effect of benzo-alpha-pyrenes on the bronchial epithelium.12 Perhaps smoking cessation efforts will eventually lead to fewer Ras-positive tumors in the future.
In esophageal cancer, Ras mutations have also been reported in patients with high-grade dysplasia and adenocarcinoma.9 These mutations are rarely found in Barrett metaplasia.
Epidermal Growth Factor Receptor (EGFR)
Unlike Ras, EGFR mutations most commonly occur in lung tumors of nonsmokers. They virtually never occur in the presence of Ras mutation and are most commonly present in adenocarcinomas of East Asian females.13,14 Along with Her2, Her3, and Her4, EGFR receptors comprise one of four types of ErbB receptors. Ligands of EGFR are varied, including both epidermal growth factor and transforming growth factor alpha.15 Ligand binding of the receptor leads to dimerization, phosphorylation, and activation of signaling pathways leading to proliferation.
Due to the availability of the EGFR-specific tyrosine kinase inhibitors, EGFR mutation has received much recent scrutiny. The majority of these mutations occur as either in-frame deletions of exon 19 or missense mutation in exon 21.13,16–21 Epithelial cells with mutant EGFR probably become physiologically dependent on the gene’s continued activity for maintenance of the transformed phenotypes.22 As Figure 7–1 reveals, patients with tumors expressing EGFR and Her2-neu may have poorer postoperative survival than those with one or neither positive.
Figure 7–1. Unpublished data from the Harpole laboratory demonstrating diminished survival in Stage I patients with tumors staining positive for both EGFR and Her2-neu.
In esophageal carcinoma, chromosome 7 (which contains EGFR) is frequently amplified in tumors that have metastasized to lymph nodes,23 and EGFR overexpression correlates with worse survival after induction treatment.24
TUMOR SUPPRESSORS
A tumor suppressor is a gene whose absence allows neoplasia to progress. Because even a small amount of wild-type protein may suppress transformation, homozygous deletion of a tumor suppressor is thought necessary for oncogenesis. When a patient is heterozygous for a gene, the term loss of heterozygosity (LOH) is used to describe deletion or inactivation of the wild-type allele.
p53
Perhaps the best-studied tumor suppressor gene is p53, which encodes a transcription factor with diverse biological functions, including the mediation of cell cycle arrest and cell death after DNA damage. The loss of wild-type (normal) p53 activity is the most common genetic abnormality in human cancer and can occur through allelic deletions or point mutations, or both, that result in amino acid substitutions that alter protein function. Abnormalities in p53 are also the most frequent genetic alterations in lung cancer, occurring in 90% of SCLCs and up to 60% of NSCLCs. These alterations primarily involve inactivating point mutations, but deletions, rearrangements, and splice mutations have also been described. As with Ras mutations, p53 mutations are more common in smokers: Denissenko and co-workers reported that benzo[a] pyrene, a major carcinogen in tobacco smoke, preferentially forms adducts at the guanine positions in p53 that are most frequently mutated in human lung cancer.25 In addition, Ahrendt and associates found that p53 mutations occur at a much higher frequency in lung cancers of patients with a history of significant alcohol and tobacco use.26
The prognostic significance of p53 abnormalities in lung cancer remains controversial. Since the half-life of mutant p53 protein is usually significantly longer than that of the wild-type protein, the detection of p53 by immunohistochemistry is considered a marker for p53 mutation, although Carbone and associates found that the concordance between positive p53 immunostaining and the presence of a p53 mutation was only 67%.27 Numerous studies have reported that p53 mutation or overexpression is predictive of poor prognosis, whereas others have found no association between p53 mutations and survival in either NSCLC or SCLC. Two recent meta-analyses concluded that the presence of p53 mutation or overexpression correlates with a poorer prognosis in patients with NSCLC.28,29 Data from the Harpole laboratory is in agreement with this (Figure 7–2).
Figure 7–2. Unpublished data from the Harpole laboratory revealing diminished survival in Stage I patients with Rb deficiency and p53 overexpression.
In esophageal cancer, p53 mutation is found in up to 80% of adenocarcinomas.30 LOH may also be important in risk stratification of patients with Barrett esophagus at risk for developing esophageal cancer.31
p16
Cytogenetic studies have identified chromosomal region 9p21 as one of the most commonly affected loci in human cancers, including lung cancer. The p16 (INK4a, CDKN2A) gene, located at 9p21, has been characterized as a tumor suppressor gene based on the high frequency of p16 mutations and deletions present in a wide variety of human tumors and the presence of germline mutations in families with hereditary melanoma. The p16 protein is one of a family of cyclin-dependent kinase (cdk) inhibitors that regulate progression through the cell cycle by inactivating cdk4 or cdk6, thereby inhibiting the phosphorylation of Retinoblastoma 1 (RB1) by cyclin D:cdk4/6 complexes. Unphosphorylated Rb1 binds E2F with resultant cell cycle arrest at the G1/S boundary. Therefore, the loss of p16 function or the overexpression of cyclin D1 would have the same net effect as the loss of RB1 function: unregulated passage through the G1/S checkpoint with resultant neoplastic proliferation. At least one of these three abnormalities—loss of RB1, loss of p16, or overexpression of cyclin D1—occurs in most, if not all, human cancers, suggesting that dysregulation of the RB–cyclin D–cdk4/6–p16 pathway controlling the G1/S transition may be a necessary step during malignant transformation.
One of these cell cycle-altering pathways is active in over 90% of NSCLC tumors.32 Although p16 inactivation is common in all NSCLCs, the mechanism of inactivation may differ between smokers and nonsmokers, with deletions and mutations primarily responsible in the former and only promoter hypermethylation being noted in the latter.33 However, others have found hypermethylation of the gene in smokers.34
In esophageal cancer, alterations in p16 are common.35,36 As with p53, p16 alterations may portend higher risk of adenocarcinoma formation in Barrett’s patients.37,38
Inactivation of p16 may be associated with a poor outcome. Kratzke and colleagues and others have reported that the loss of p16 is associated with a poor prognosis,39 while Kinoshita and associates found that inactivation of p16 or RB1 was associated with an increased proliferative index in tumors lacking p53 activity.
GENOMICS AND PROTEOMICS
Genomics
As technology has evolved and the human genome has been sequenced, the ability to screen samples for expression/mutation across large numbers of genes has become both possible and affordable. These techniques involve isolating either RNA (in the case of transcriptome profiling) or DNA (in the case of single nucleotide polymorphism [SNP] arrays), fluorescently labeling them, and hybridizing them to chips to which are immobilized short DNA sequences (probes) from thousands of genes of interest. After reading and analysis, an expression value for each probe is obtained. Commercially available chips now contain all known genes in the genome of humans and almost any other organism under study.
The vast amount of information obtained from these arrays has necessitated the explosion of the field of bioinformatics in that complex, computerized statistical algorithms have been developed to identify important significant differences between samples. In the case of the Affymetrix (www.affymetrix.com) human genome U133 2.0 Plus chips, expression data for over 54,000 probes is provided for each sample.
As these fields have advanced, these approaches have been applied to human lung cancer. Several groups including ours have demonstrated that squamous cell carcinoma and adenocarcinoma have markedly different transcriptome profiles.40–43 Within these broad histologic classes, these studies have also identified subgroups that have poor prognosis. These types of studies may eventually lead to personalized treatment.
In contrast to the transcriptome array studies discussed thus far, sequencing of the human genome has led to the accumulation of single nucleotide polymorphism data. These polymorphisms represent single base pair differences between individuals and may either cause disease (if the change causes differences in protein expression) or be used as markers of disease (if a SNP is very close to a gene contributing to a disease phenotype such that recombination happens between the gene and the SNP only rarely). Large SNP arrays are now commercially available. The protocol for their use is similar to that of transcriptome arrays except that genomic DNA is hybridized to the chips. Because this technology is relatively new, less lung cancer-specific data has been published. However, recent studies have demonstrated increased lung cancer risk with specific polymorphisms.44–47 As the technology becomes more affordable and easier to use, more of these studies will undoubtedly be published over the next several years. Because these studies identify genetic markers in genomic DNA which can be isolated and analyzed at any time from a blood sample, a myriad of ethical considerations will inevitably arise concerning testing of healthy people for increased lung cancer susceptibility.
Proteomics
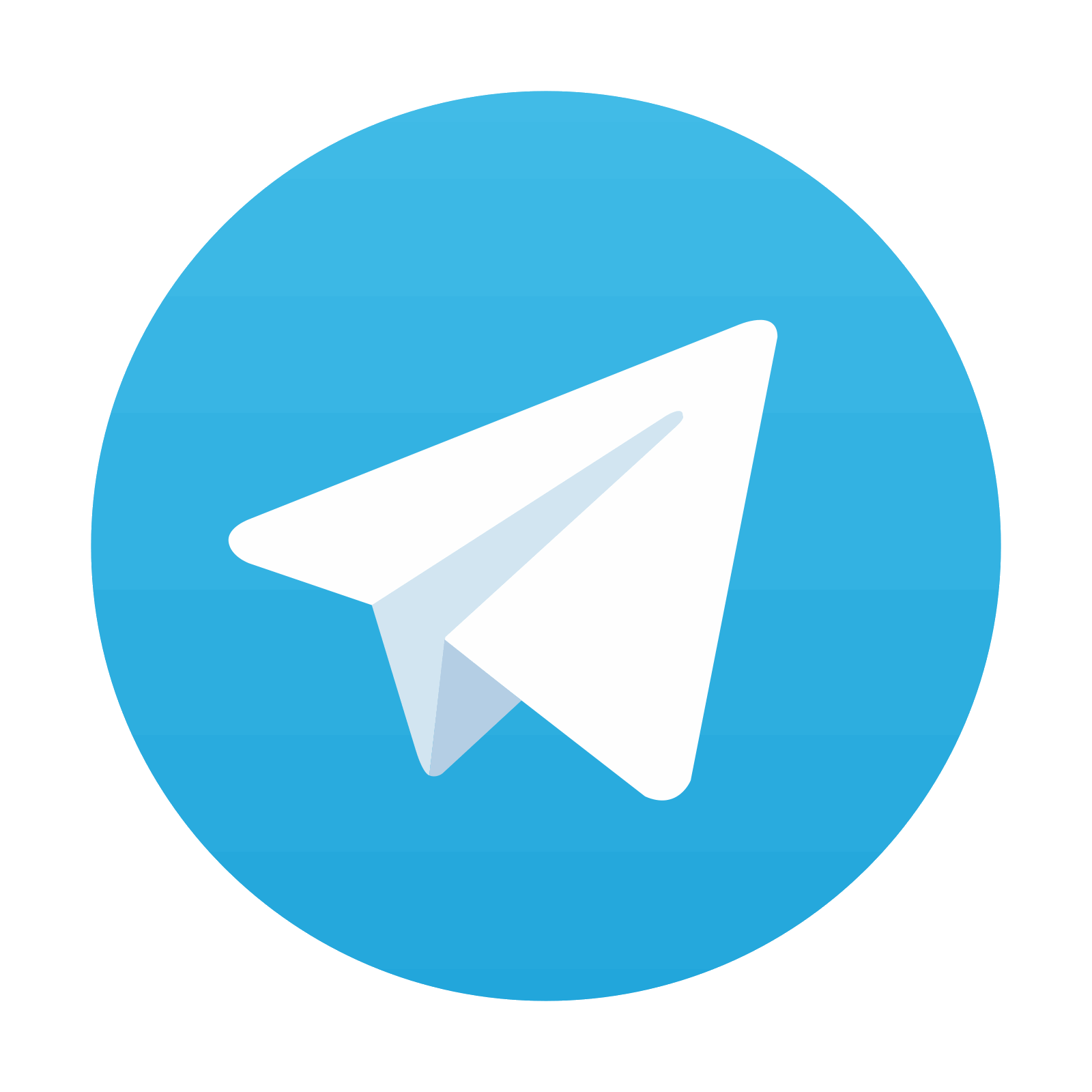
Stay updated, free articles. Join our Telegram channel
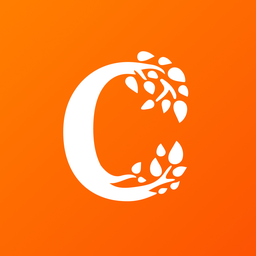
Full access? Get Clinical Tree
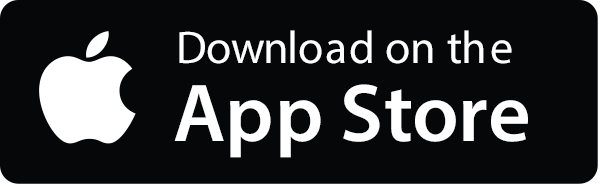
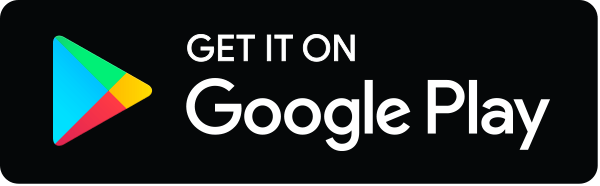