FIGURE 26.1 Zero-heat-flux cutaneous thermometer consists of a thermal insulator covered by an electric heater. The heater is servo-controlled to eliminate the heat flow through the insulator, at which point heater and skin temperatures are equal. Tissue temperature at the skin surface and just below the monitor must also be the same as subdermal temperature to avoid heat accumulation, which enables it to effectively measure tissue temperature approximately 1 to 2 cm below the skin surface. (From Eshraghi Y, Nasr V, Parra-Sanchez I, et al. An evaluation of a zero-heat-flux cutaneous thermometer in cardiac surgical patients. Anesth Analg 2014;119:543–549.)
Because the Second Law of Thermodynamics specifies that heat can only flow down a temperature gradient, there can be no flow of heat across an insulating layer which has identical temperatures on the skin and ambient surfaces. The combination of the insulating layer and servo-controlled heater thus becomes a perfect insulator. Heat flowing from the core toward the skin surface thus cannot be dissipated through the monitor’s insulating layer, and is reflected internally. After a suitable equilibration period, temperature of tissues 1 to 2 cm below the monitor resemble nearly equal temperature of deeper tissues (5). In appropriate body locations such as forehead and neck, temperature just below the skin surface is essentially core temperature (Fig. 26.1).
This device was originally manufactured in Japan with a heavy nondisposable probe that takes considerable time to reach thermal equilibrium (6). A new device is now available with a lightweight disposable probe that takes about 3 minutes to reach thermal equilibrium because of its low thermal mass (5). Forehead and neck zero-heat-flux thermometer measurements reflect core temperature in the pulmonary artery with a mean bias of −0.23° and −0.30°C, respectively. The average perioperative difference between forehead zero-heat-flux and pulmonary artery temperatures in elective cardiac surgery with CPB was −0.08° to −0.32°C, and 84% of measurements were within 0.5°C difference. It is likely that results are better in the target population of general surgical patients in whom temperature perturbations are lower and slower.
SITES OF TEMPERATURE MEASUREMENT
The core thermal compartment comprises well-perfused tissues whose temperature is uniform, and high, compared with the rest of the body. Core temperature is defined as the average temperature of the core compartment; it is not the highest temperature in the body, which may be found in the brain or liver. Core temperature can be evaluated in the pulmonary artery, distal esophagus, tympanic membrane with a contact probe, or nasopharynx with a probe inserted at least 10 cm.
The temperature equilibration rate between the blood and the organ measured is affected by various factors, such as the types of organ and the rate of temperature change. The average time lag between monitoring sites and the pulmonary artery (the gold standard) are reported as 5 minutes for the esophagus and forehead (zero-heat-flux measurement), 8 minutes for nasopharynx, 10 minutes for actual tympanic membrane, 15 minutes for rectum, and 20 minutes for bladder. Nasopharyngeal temperatures remain close to the brain temperature even during profound hypothermia, although the temperature gradients as large as 5°C have been observed between the brain and bladder and rectal temperatures (7).
The most common site of temperature measurement is probably under the tongue, at least in nonclinical environments. Unfortunately, hot and cold drinks, open-mouth versus close-mouth breathing, and tachypnea all affect oral temperature readings. However, nasogastric tubes do not interfere with oral temperature measurement (8–10). Carefully performed oral temperatures are remarkably accurate (11), while the accuracy of axillary temperature measurements is worse than at other sites, probably due to probe positioning (12). Axillary measurements are nonetheless usually suitable if care is taken to position the probe over the axillary artery and to keep the arm adducted.
Rectal temperature measurement is most accurate when the sensor is inserted more than 10 cm into the rectum; the rectal temperature correlates well with distal esophageal, bladder, and tympanic temperatures although, typically, it slightly exceeds the core value. However, this site responds slowly to rapid changes in body temperature (13). Consequently, rectal readings are often erroneous—sometimes by degrees—during heat stroke, malignant hyperthermia, and other situations where core temperature changes rapidly (14). It is thus a poor choice when, as usual, the purpose of monitoring is to detect rapid perturbations.
Tympanic membrane temperatures, obtained with a contact thermocouple or thermistor, are highly accurate core temperatures (15). By way of contrast, infrared aural canal “tympanic” temperatures do not actually evaluate the tympanic membrane and are far less accurate than actual tympanic membrane temperatures, thus possibly representing rather poorly the core temperature (16). Users must ensure that the aural canal is not obscured by cerumen and that the ear canal is straightened by manipulating the ear lobe so that the sensor can point directly at deeper sections of the canal which are nearer to core temperature (17). Even though infrared aural canal thermometers are often labeled as “tympanic,” they do not actually “see” the tympanic membrane but extrapolate tympanic or core temperature from the skin temperature in the aural canal. Earphone-type infrared tympanic thermometer may work better than conventional infrared aural canal thermometers (18).
Distal esophageal temperature is an accurate core temperature monitoring site when patients are anesthetized or sedated, and the site is resistant to artifact (19). Probes are best situated in the lower third of the esophagus near the point of maximum heart sounds (about 45 cm from the nose in adults), but temperatures remain accurate over a broad range of insertion distances. Temperatures obtained from the proximal and mid-esophagus may be cooled by the ambient air because they are near the trachea and bronchi—although the artifact is generally small. Transesophageal echocardiography (TEE) may affect the esophageal temperature because heat is emitted by the probe. In intubated patients, the esophagus is usually the preferred temperature monitoring site since it provides true core temperature, measurements are easy to obtain using inexpensive probes, and the site is highly resistant to artifact.
Nasopharyngeal temperature monitoring is often used during general anesthesia or in adequately sedated patients. It is an excellent site and provides accurate and precise measurements of core temperature so long as probes are inserted at least 10 cm past the nares in adults. The nasopharynx is an excellent alternative to esophageal temperature and is sometimes preferable since access to the esophagus is restricted by laryngeal mask airways (20).
Urinary bladder temperature is measured by placing a probe in an indwelling urinary bladder catheter (IUBC). The measurement is affected by urinary flow during cardiopulmonary bypass and does not provide an accurate temperature measurement when urine flow is low (21). Under other circumstances, though, it is a reasonably accurate site.
Pulmonary artery temperature is measured by a sensor located at the distal end of the balloon-tipped, flow-directed pulmonary artery catheter. It is generally considered the single best core temperature measurement site, although obviously available in only a tiny fraction of patients. It is worth noting that brain temperature is slightly greater than pulmonary artery temperature, because core temperature at the pulmonary artery is the average value of the deep body structures. The gradient between core and brain temperatures tends to increase with fever and active cooling.
THERMOREGULATION
Precise control of core temperature is maintained by powerful thermoregulatory systems incorporating afferent inputs, central control, and efferent defenses. Efferent defenses can be broadly divided into autonomic responses (e.g., sweating and shivering) and behavioral responses (e.g., closing a window, putting on a sweater). Autonomic responses depend largely on core temperature and are mostly mediated by the anterior hypothalamus. In contrast, behavioral responses are roughly 50% determined by skin temperature and are controlled by the posterior hypothalamus (22).
Thermoregulation is maintained by feedforward and feedback pathways (23). Signals from cutaneous thermosensors that detect changes in environmental temperature are transmitted to the thermoregulatory center in the hypothalamus. The thermoregulatory center also receives signals from deep-tissue thermosensors which sense the body temperature. Effectors receiving efferent signals from the thermoregulatory center control thermoregulatory homeostasis. Recent studies suggest that the feedforward pathway may be more important than previously appreciated (Fig. 26.2).
Afferent Input
Dual detectors—cold sensors with myelinated A-δ fibers and warm sensors with unmyelinated C fibers—exist in the subcutaneous layer of the skin. The distribution density of cold sensors is several times greater than warm sensors. Skin temperature reflects a balance between body temperature and environmental conditions, thus serving as an “early warning” system for anticipated temperature changes to deeper tissues. Thermosensors are also located in brain areas such as the hypothalamus, midbrain, and spinal cord, and in deep thoracic and abdominal tissues. These deep sensors detect core body temperature.
TRP Channels as Putative Molecular Thermosensor
The transient receptor potential (TRP) channel superfamily in humans consists of 27 members that are subclassified into 6 subfamilies based on their sequence homology: TRPC (canonical), TRPV (vanilloid), TRPM (melastatin), TRPML (mucolipin), TRPP (polycystin), and TRPA (ankyrin). The TRP channel is a nonselective cation channel with six transmembrane domains, a pore-forming loop between the fifth and the sixth segments, and intracellular C-terminus and N-terminus (24).
Since finding the first temperature sensitive receptor, TRPV1 in 1997, 10 TRP channels (TRPV1, TRPV2, TRPV3, TRPV4, TRPM2, TRPM3, TRPM4, TRPM5, TRPM8, and TRPA1) have been reported to be highly temperature-sensitive. Furthermore, five of them—TRPV1, TRPV2, TRPM3, TRPM8, and TRPA1—are expressed on human sensory neurons (Fig. 26.3). Although each thermo-TRP channel is activated within a narrow temperature range; these channels cumulatively cover a broad temperature range, from noxious cold (TRPA1 < 17°C) to noxious heat (TRPV2 > 52°C). This feature strongly suggests that at least some thermo-TRP channels are the prime or sole molecular thermosensors responsible for the reception of peripheral temperature (25,26).

FIGURE 26.2 Thermoregulation. Signals from the cutaneous thermosensors detecting changes in environmental temperature are transmitted to the thermoregulatory center. The effectors receiving efferent signals control thermoregulatory homeostasis (feedforward). Also, the deep thermosensors detecting changes in body temperature transmit the afferent signals to the central controller (feedback).

FIGURE 26.3 Temperature thresholds of five thermo-TRP channels on human sensory nerve. Although each thermo-TRP channel is activated within a narrow temperature range, these channels cumulatively cover a broad temperature range, from noxious cold (TRPA1 < 17°C) to noxious heat (TRPV2 > 52°C).
TRPV1 is expressed in A-δ and C fibers and is best characterized among TRP channels as the major receptor for capsaicin, one of the vanilloids, the spicy ingredient in hot chili peppers. As might be expected, TRPV1 antagonists cause hyperthermia as well as elevating the heat pain threshold in human patients (27).
In cultured neurons from TRPV1-deficient mice, no responses to heat (43° to 55°C) or capsaicin were observed, though heat responses at higher temperatures remained intact. Furthermore, these mice showed a longer latency to noxious heat, but normal latencies to pain stimuli (28). Subsequent studies revealed that TRP channels are polymodal nociception receptors that respond to various nociceptive stimuli such as membrane stretch, protons, and chemical ligands, as well as noxious heat. Hypothalamic TRPV1 channels are not activated at physiologically normal temperatures, as their response thresholds exceed 43°C (29).
Recent studies of TRP channels have indicated that additional TRP-independent mechanisms might play a major role in the transduction of physiologic thermal signals. Although TRP channels as nociceptors detect noxious heat, the involvement of TRPV1 as thermosensors in response to temperature within a physiologic range remains debatable (30,31).
TRP receptors also mediate nonthermal pain, but efforts to develop antagonists as analgesics have so far been limited because the drugs simultaneously produce hyperthermia—presumably, by blocking peripheral warm input, thus fooling the thermoregulatory system into believing the body is cooler than it truly is.
Central Control
Thermal afferent signals are integrated at numerous levels within the neuraxis, including the spinal cord and brain stem. While the hypothalamus is undoubtedly the dominant and most precise controller of body temperature, warm sensors are thought to mediate many of the stimulatory and inhibitory signals to effectors, as there are few cold sensors in the hypothalamus.
Core temperature varies with a daily circadian rhythm (32). Normal temperature is altered slightly by factors such as age (0.5°C lower in the elderly), time of day (1°C higher in the afternoon), time of menstrual cycle in women (higher near ovulation), and exercise (33). Nonetheless, core temperature is normally controlled to within a few tenths of a degree centigrade virtually irrespective of the environment (34). The thresholds triggering thermoregulatory defenses are uniformly about 0.3°C greater in women than in men, even during the follicular phase (34), and are an additional approximately 0.5°C greater during the luteal phase (35). However, men and women regulate core body temperature with comparable precision (Fig. 26.4).
Nonthermoregulatory cutaneous circulatory reflexes, including cardiopulmonary and arterial baroreceptor reflexes, modulate thermoregulatory vascular tone. Cardiopulmonary baroreceptor reflex controls peripheral vascular resistance in response to the change in blood volume through the central sympathetic nervous system and the renin–angiotensin pathway. The baroreceptor reflex is modified by the right atrial transmural pressure, which is the difference between central venous pressure and the intrathoracic pressure. Baroreflex loading, by increased right atrial pressure in patients placed in the leg-up position, results in an exaggeration of anesthesia-induced hypothermia because of attenuated peripheral vasoconstriction. In contrast, positive end-expiratory pressure (PEEP) ventilation decreases right atrial transmural pressure and, as a consequence, attenuates perioperative hypothermia (Fig. 26.5) (36,37).
Efferent Responses
The balance between heat production and heat loss determines body temperature; nonevaporative heat loss depends on the difference between skin temperature and environmental temperature. Given that skin blood flow is a primary determinant of skin temperature—increased blood flow results in increased skin temperature—cutaneous vascular tone modulates heat loss. But when environmental temperature exceeds body temperature, evaporative heat loss—sweating—is the only way to lose heat.

FIGURE 26.4 The thresholds (triggering core temperatures) for the three major autonomic thermoregulatory defenses: sweating, vasoconstriction, and shivering. Temperatures between the sweating and vasoconstriction threshold define the interthreshold range, temperatures not triggering autonomic responses. The thresholds are uniformly about 0.3°C greater during the follicular phase in women than in men, and are an additional ≈0.5°C greater during the luteal phase. However, men and women regulate core body temperature with comparable precision. Results are presented as means ±SD. (From Lopez M, Sessler DI, Walter K, et al. Rate and gender dependence of the sweating, vasoconstriction, and shivering thresholds in humans. Anesthesiology 1994;80:780–788.)

FIGURE 26.5 The esophageal temperature (Tes) after induction of anesthesia. Positive end-expiratory pressure (PEEP: 10 cm H2O, P) or the leg-up position (L) was applied 10 minutes after induction of anesthesia. Baroreflex loading due to leg-up position exaggerates anesthesia-induced hypothermia because peripheral vasoconstriction is attenuated. Meanwhile, PEEP attenuated perioperative hypothermia because of stimulated vasoconstriction through baroreceptor unloading. Values are shown as mean ± SE. Significant difference compared with the control group (C). (From Nakajima Y, Mizobe T, Takamata A, Tanaka Y. Baroreflex modulation of peripheral vasoconstriction during progressive hypothermia in anesthetized humans. Am J Physiol Regul Integr Comp Physiol 2000;279:R1430–1436.)
Sweating is mediated by postganglionic cholinergic nerves that terminate on sweat follicles (38); these follicles apparently have no purpose other than thermoregulation. In this regard, they differ from most other thermoregulatory effectors that appear to have been co-opted by the thermoregulatory system but continue to play other important roles, such as vasomotion in blood pressure control and skeletal muscles in postural maintenance. Heat exposure can increase cutaneous water loss from trivial amounts to 500 mL/hr. Sweat loss in trained athletes can even exceed 1 L/hr. The process is remarkably effective, dissipating 0.58 kcal/g of evaporated sweat. In a dry, convective environment, sweating can thus dissipate enormous amounts of heat—perhaps up to 10 times the basal metabolic rate.
Cutaneous vasoconstriction is the first autonomic response to cold; metabolic heat is lost primarily via convection and radiation from the skin surface, and vasoconstriction reduces this loss. Active arteriovenous shunt vasoconstriction is adrenergically mediated. The shunts are vessels 100 µm in diameter and thus convey 10,000 times as much blood as a comparable length of 10-µm capillaries (laminar flow increases by the fourth power of radius) (39). Anatomically, they are restricted to the fingers, toes, nose, and nipples. Despite this restriction, shunt vasoconstriction is among the most commonly used and important thermoregulatory defenses. Roughly 10% of cardiac output (CO) traverses dilated arteriovenous shunts; consequently, shunt vasoconstriction increases mean arterial pressure by approximately 15 mmHg.
A major purpose of thermoregulatory vasoconstriction is to isolate core tissues from the environment and thus restrict peripheral-to-core heat transfer. The normal threshold (triggering core temperature) for vasoconstriction is approximately 36.5°C (34). Thermoregulatory vasoconstriction is more effective than might be imagined, but when environmental temperature is very low, heat production must increase if thermal homeostasis is to be maintained because maximal cutaneous vasoconstriction cannot compensate for the amount of heat loss. The thermoneutral zone can be defined as the range of environmental temperature over which cutaneous vasomotion alone can maintain body temperature (Fig. 26.6). The environmental temperature at which heat production increases, the lower critical temperature is about 19°C; while the temperature at which evaporative heat loss starts, the upper critical temperature is about 31°C.

FIGURE 26.6 The thermoneutral zone is defined as the environmental temperature zone in which skin vascular tonus alone can maintain body temperature. The environmental temperature at which heat production increases, the lower critical temperature is 29°C; while the temperature at which evaporative heat loss starts, the upper critical temperature is 31°C.
In humans, shivering is the final autonomic response to be activated by cold exposure and is generally only observed when behavioral responses and vasoconstriction fail to maintain an adequate core temperature. The shivering threshold is approximately 35.5°C (34), which is about 1°C less than the vasoconstriction threshold. Shivering begins with the pectoralis muscles, but most shivering thermogenesis occurs in the extremities where the largest muscles are located. Typically, vigorous shivering doubles the metabolic rate (40,41), although greater increases can be sustained briefly (42,43).
An obvious consequence of shivering is the increased metabolic rate. This increase, which is analogous to exercise, provokes a substantial adrenergic response. For example, a reduction in core temperature of only 0.7°C increases norepinephrine (NE) concentration by 400% and oxygen consumption by 30%. When core temperature decreases to 1.3°C, NE concentration increases 700% and oxygen consumption doubles. As might be expected, shivering is associated with peripheral vasoconstriction and hypertension—although heart rate remains unchanged, as do plasma epinephrine and cortisol concentrations (44).
The interaction between thermal input, central control, and effector responses is shown in Figure 26.7, which also shows the normal values for the major autonomic response thresholds. In the elderly, the vasoconstriction threshold is reduced (45). Similarly, the shivering threshold is significantly reduced in the elderly (46). Interestingly, abnormally reduced thresholds were not apparent in subjects less than 80 years of age, and even then in only a fraction of the population (Fig. 26.8).

FIGURE 26.7 Thermoregulation and energy metabolism. In the sympathetic nervous system, norepinephrine (NE) is a primary neurotransmitter to activate beta-adrenergic receptor on the targeted organs (beta-2 receptors on the viscera, skeletal muscle and white adipose tissue (WAT); and beta-3 receptors on brown adipose tissue (BAT). The sympathetic nervous system also induces insulin secretion from pancreas which results in activated protein synthesis in skeletal muscles. Thyroid hormone stimulates oxidative phosphorylation and ATP synthesis in the mitochondria, which results in the activated energy metabolism in the targeted tissues. Also, thyroid hormone promotes thermogenesis by uncoupling oxidative phosphorylation via uncoupling protein (UCP). UCP1, expressed only in the mitochondria of BAT, facilitates the reflux of protons across the mitochondria down the proton gradient, bypasses ATP synthesis, and generates heat. Expression of UCP1 is required for BAT thermogenesis, and free fatty acids released from WAT serve as the main fuel for thermogenesis in BAT. Thermogenic capacity of BAT is reported about 344 W/kg whereas that of nonspecific tissue is about 4.1 W/kg.
The most powerful inhibitors of thermoregulation are general anesthetics. These drugs have been used to facilitate induction of hypothermia for cardiac surgery and during neurosurgery. In sufficient doses, especially when combined with neuromuscular blockers, anesthetics can essentially obliterate defenses against hypothermia.
Volatile anesthetics, such as desflurane and sevoflurane, have relatively little effect on sweating: even full anesthetic doses increase the sweating threshold by only about 0.5°C. In contrast, anesthetic gases markedly reduce the vasoconstriction and shivering thresholds. Interestingly, the threshold for each major cold defense is reduced synchronously, as if they are similarly controlled (Fig. 26.9) (47).

FIGURE 26.8 The effect of aging on the shivering threshold. Fifteen patients less than 80 years old (58 ± 10 year) (mean ± SD) shivered at 36.1 ± 0.6°C; in contrast, 10 patients aged 80 years or older (89 ± 7 year) shivered at a significantly lower mean temperature, 35.2 ± 0.8°C (P < 0.001). The shivering thresholds in 7 of the 10 patients 80 years or older was less than 35.5°C, whereas the threshold equaled or exceeded this value in all the younger patients. (From Vassilieff N, Rosencher N, Sessler DI, et al. The shivering threshold during spinal anesthesia is reduced in the elderly. Anesthesiology 1995;83:1162–1166.)
Neuromuscular blockers are not believed to have any effect on central control of thermoregulatory responses. But they obviously cause paralysis and thus prevent shivering. It is equally obvious though that muscle relaxants can only be given in the context of general anesthesia or substantial sedation and that paralyzed patients require mechanical ventilation. Muscle relaxants can be used as a treatment for shivering in intensive care units (48), but are not a substitute for adequate sedation and appropriate thermal management.
Thermogenesis and Energy Metabolism
All tissues generate heat which helps maintain body core temperature, especially the liver, brain, skeletal muscle, and brown adipose tissue (BAT). Most heat generation is simply a byproduct of organs doing their intended work. But to a modest extent, thermogenesis is regulated by the thermoregulatory center in the hypothalamus through both the sympathetic nervous and endocrine hypothalamic pituitary systems (Fig. 26.7). The two systems are known to regulate various metabolic processes in a synergistic and complementary fashion (49).
In the sympathetic nervous system, NE is the primary neurotransmitter to activate the beta-adrenergic receptor on target organs (beta-2 receptors on the viscera, skeletal muscle, and white adipose tissue (WAT); and beta-3 receptors on BAT). But the sympathetic nervous system also induces insulin secretion from pancreas which results in activated protein synthesis in skeletal muscles. Thyroid hormone, controlled primary by thyrotropin-releasing hormone (TRH) from the hypothalamus and secondary by thyroid-stimulating hormone (TSH) from the pituitary gland, binds with its intranuclear receptor in the target organs. Local activation of circulating serum thyroxine (T4) to the active form of triiodothyronine (T3), converted by the iodothyronine deiodinase type 2 (D2) with no change in the serum concentration of T3, is a main mechanism of thyroid hormone regulation.
D2, expressed in key thyroid-targeting tissue, is the primary enzyme responsible for the intracellular T3 regulation. Increased intracellular T3 stimulates oxidative phosphorylation and ATP synthesis in the mitochondria which enhances energy metabolism. T3 also promotes thermogenesis by uncoupling oxidative phosphorylation via uncoupling protein (UCP). UCP1, expressed only in the mitochondria of BAT, facilitates the reflux of protons across mitochondria membranes; pumping protons back across the membrane is an ATP-requiring process that generates heat. Expression of UCP1 is required for BAT thermogenesis, which is synergistically regulated by both NE and T3. Unlike organs and other human tissues, heat production by BAT is entirely facultative.

FIGURE 26.9 Concentration-dependent thermoregulatory inhibition by desflurane and isoflurane (halogenated volatile anesthetics), propofol (an intravenous anesthetic), and alfentanil (a µ-agonist opioid). General anesthetics are the most powerful known inhibitors of thermoregulatory control. The horizontal axis, in each case, spans a clinically relevant concentration range. The sweating (triangles), vasoconstriction (circles), and shivering (squares) thresholds are expressed in terms of core temperature at a designated mean skin temperature of 34°C. Anesthesia linearly, but slightly, increases the sweating threshold. In contrast, anesthesia produces substantial and comparable linear or non-linear decreases in the vasoconstriction and shivering thresholds. Typical anesthetic concentrations thus increase the interthreshold range (difference between the sweating and vasoconstriction thresholds) approximately 20-fold from its normal value near 0.2°C. Patients do not activate autonomic thermoregulatory defenses unless body temperature exceeds the interthreshold range; surgical patients are thus poikilothermic over a 3° to 5°C range of core temperatures. Isoflurane, 1%, and desflurane, 6%, have comparable anesthetic potency. Error bars smaller than the data markers have been deleted. (From Sessler DI. Perioperative hypothermia. N Engl J Med 1997;336:1730–1737. Copyright ©1997 Massachusetts Medical Society. Reprinted with permission from Massachusetts Medical Society.)
Humans have both subcutaneous and visceral BAT. Recent studies using CT and PET imaging reveal substantial amounts of BAT in the subscapular, supraclavicular, and chest regions in adults (50), with younger and leaner subjects having more BAT. Also, cold exposure activates BAT within minutes, which is inhibited by beta-adrenergic antagonists. Persistent cold exposure affects BAT, resulting in increased expression of UCP1, increased mitochondria, and hyperplasia and hypertrophy via the sympathetic nervous system. In this process, free fatty acids released from WAT serve as the main fuel for thermogenesis in BAT. Thermogenic capacity of BAT is reported about 344 W/kg whereas that of nonspecific tissue is about 4.1 W/kg. Nonetheless, BAT contributes only small amounts to total metabolic heat production in adults and if it is clinically important, probably contributes more to long-term homeostasis (body weight stability) than protection against cold exposure.
TARGETED TEMPERATURE MANAGEMENT
Targeted temperature management (TTM), historically referred to as therapeutic hypothermia, is a clinical concept of therapeutic hypothermia followed by induced normothermia. However, it can also refer to fever prevention. While potentially applicable to most any tissue, clinical use has focused on the brain and heart (51).
Cardiac Arrest
Postcardiac arrest syndrome includes three characteristic phases of injury, largely identified in animal studies (Fig. 26.10). The first is the intra-arrest ischemic injury phase; it is due to absent blood flow, and results in energy failure, ischemic depolarization of cell membrane, release of excitatory amino acids, and cytosolic calcium overload. With return of spontaneous circulation, early reperfusion is associated with reactive oxygen generation and mitochondria calcium overload, which triggers apoptosis pathways. The delayed reperfusion phase is characterized by subsequent neuronal calcium overload, activation of pathologic proteases, and systemic inflammation. Each phase is regarded as a potential target of TTM with different therapeutic windows.

FIGURE 26.10 Time course of neuronal injury mechanisms during and after cardiac arrest and the different phases during which injury occurs. The shapes of the individual curves schematically depict the severity and duration of injury during each phase. ROSC, return of spontaneous circulation. (From Perman SM, Goyal M, Neumar RW, et al. Clinical applications of targeted temperature management. Chest 2014;145:386–393. Copyright ©2014; from Elsevier.)
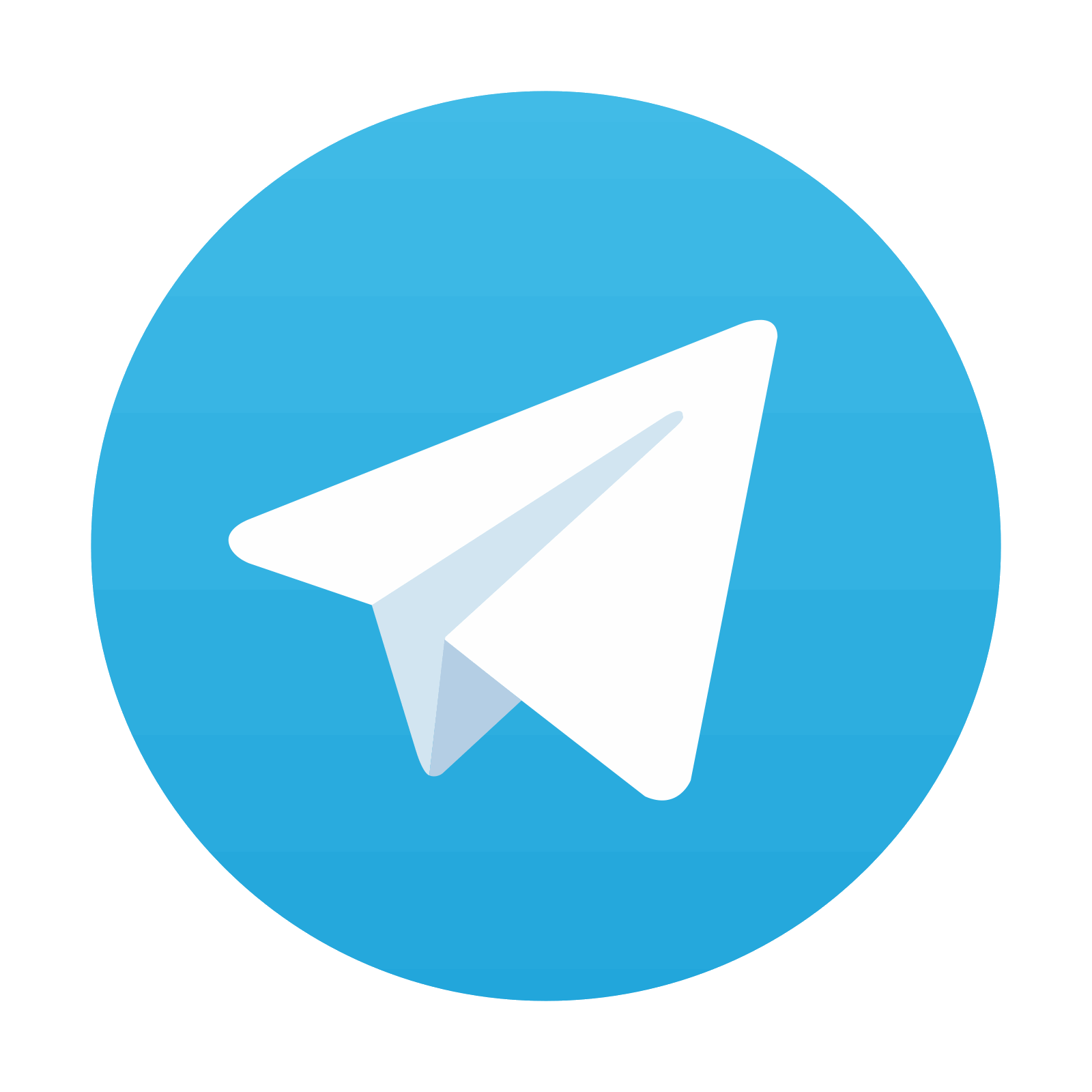
Stay updated, free articles. Join our Telegram channel
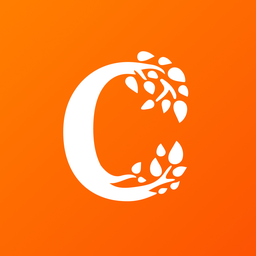
Full access? Get Clinical Tree
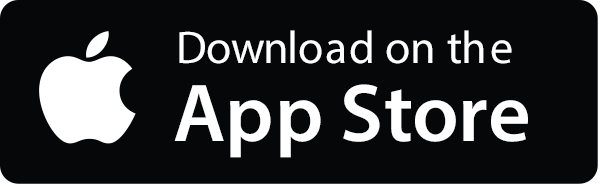
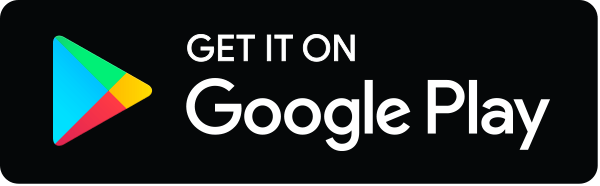