Shock: An Overview
Michael L. Cheatham
Ernest F.J. Block
Howard G. Smith
Matthew W. Lube
John T. Promes
Shock is one of the most complex conditions encountered in the critically ill patient. The term “shock” encompasses a broad range of pathologic processes that may require diametrically opposed methods of treatment. The underlying cause may be quite evident, as in traumatic hemorrhage, or occult, as in severe sepsis due to infection. Delayed shock resuscitation is associated with significant morbidity and mortality. Therapy must commonly be initiated before all clinical information and diagnostic studies are available. As a result, the intensivist must possess a solid understanding of the common shock states, their clinical presentation, and the necessary therapeutic interventions. Although mortality remains high, increasing application of early goal-directed resuscitation to achieve defined physiologic endpoints has significantly improved patient outcome from shock [1,2,3].
Over the centuries, shock has been defined in various ways. In 1534, Ambrose Pare wrote that shock was caused by “toxins in the blood” and recommended phlebotomy as the treatment, a practice that persisted until the early 1800s. By that time, shock-associated hypotension was well recognized as was the detrimental impact of bloodletting on systemic perfusion [4]. Although subsequent early definitions of shock lack scientific terminology, they compensate for this in their simplicity. John Collins Warren described shock as “a momentary pause in the act of death,” whereas Samuel David Gross defined shock as “a rude unhinging of the machinery of life” [5]. In the 1930s, Alfred Blalock published his classic series of investigations into shock confirming that hypotension was due to loss of blood and plasma into the tissues (so called “third-space losses” due to increased capillary permeability) [6]. Blalock found that the hypotension and high mortality of shock were reversible through the infusion of crystalloid solutions to replace lost intravascular and interstitial fluid, and that simple reinfusion of lost blood was not sufficient. Shock was thus identified as a systemic disorder caused by increased vascular permeability, interstitial edema, and intravascular volume depletion with the classic signs of hypotension, decreased urinary output, and multiple organ failure.
The importance of regional end-organ perfusion, rather than simply systemic blood flow alone, is the singular concept for recognizing and improving patient outcome from shock. Perfusion may be decreased either systemically (as in hemorrhagic or cardiogenic shock) or only regionally (as in septic shock) with global perfusion being normal or even elevated. Regardless of cause or severity, all forms of shock have the commonality of perfusion inadequate to meet metabolic demands at the cellular level. Decreased organ perfusion leads to tissue hypoxia, anaerobic metabolism, activation of the inflammatory cascade, and eventually organ dysfunction. The ultimate consequences of shock depend on the degree and duration of hypoperfusion, the number of organs affected, and the presence of prior organ dysfunction. The challenges to the intensivist are identifying the hypoperfused state, diagnosing its cause, and rapidly restoring cellular perfusion.
Physiology
Significant progress has been made in elucidating the cellular basis for shock. Although low blood pressure and other vital sign derangements were previously thought to be sufficient to cause shock, they are now recognized as being signs of a complex physiologic cascade of events. The delivery and consumption of oxygen at the mitochondrial level, as well as the adequate removal of cellular waste products, is of paramount importance to survival. Cellular hypoxia leads to local vasoconstriction, thrombosis, anaerobic glycolysis, release of superoxide radicals, accumulation of pyruvate and lactate, and intracellular acidosis. The severity of a patient’s acidemia, demonstrated by elevated base deficit or lactate levels, correlates with the lethality of shock [7].
In patients who experience such an anaerobic insult, injured tissues and damaged cells release a variety of intracellular mediators which initiate the proinflammatory cascade. Cytokines are small polypeptides and glycoproteins produced by a variety of immunologic cells that are responsible for many of the sequelae seen during shock. Tumor necrosis factor alpha (TNF-α) is one of the earliest cytokines released and is a product of monocytes, macrophages, and T-cells. TNF-α levels rise after a variety of cellular insults and cause hypotension, procoagulant activity, muscle breakdown, catabolism and cachexia. TNF-α levels have been seen to correlate with mortality in animal models of hemorrhagic shock [8]. Produced by macrophages and endothelial cells, interleukin-1 (IL-1) has similar effects, producing fever and anorexia. Activated T-cells produce interleukin-2 which augments cell mediated immunity. Interleukin-6, together with IL-1, mediates the acute phase response to injury and may have a role in the development of acute lung injury. Interleukin-8 is chemotactic for neutrophils and interleukin-12 has a role in cell-mediated immunity by promoting the differentiation of T-helper 1 cells. A variety of “anti-inflammatory” cytokines such as growth hormone interleukin-4, interleukin-10, interleukin-13, soluble TNF receptors (sTNFR), and IL-1 receptor antagonists (IL-1ra) are simultaneously released in an attempt to counterbalance the proinflammatory cascade.
These proinflammatory and counter-regulatory substances may lead to processes that may not be in the best interest of the patient in shock. The body’s (mal)adaptive response to the primary injury or inciting event may cause secondary injury to previously unaffected cells and organs leading to impaired perfusion, cellular death, and organ dysfunction. This systemic inflammatory response syndrome, if left unabated, may result in the multiple organ dysfunction syndrome, a common cause of shock-related morbidity and mortality.
IL-1 also activates the patient’s hypothalamopituitary axis (HPA) as well as the neuroendocrine response to critical illness. HPA activation releases adrenocorticotrophic hormone (ACTH) that acts on the adrenal gland to stimulate
glucocorticoid (cortisol) production. Appropriate adrenocortical response to shock is essential for patient survival. Relative adrenal insufficiency during critical illness is a commonly underappreciated reason for a patient’s failure to respond to resuscitative interventions [9]. Vasopressin (antidiuretic hormone [ADH]) is cosecreted from the posterior pituitary and potentiates the effect of ACTH. In addition to its primary osmoregulatory role in resorption of water from the nephron’s collecting duct, ADH is also a potent vasoconstrictor, improving systemic perfusion, and promoting gluconeogenesis and glycolysis to provide much needed metabolic substrates.
glucocorticoid (cortisol) production. Appropriate adrenocortical response to shock is essential for patient survival. Relative adrenal insufficiency during critical illness is a commonly underappreciated reason for a patient’s failure to respond to resuscitative interventions [9]. Vasopressin (antidiuretic hormone [ADH]) is cosecreted from the posterior pituitary and potentiates the effect of ACTH. In addition to its primary osmoregulatory role in resorption of water from the nephron’s collecting duct, ADH is also a potent vasoconstrictor, improving systemic perfusion, and promoting gluconeogenesis and glycolysis to provide much needed metabolic substrates.
The neuroendocrine response to shock involves many counter-regulatory substances. Epinephrine and norepinephrine are produced from the adrenal medulla and synapses of the sympathetic nervous system respectively. β-Adrenergic stimulation results in increased heart rate and contractility, and α-adrenergic stimulation increases systemic vascular resistance and blood pressure through peripheral vasoconstriction. Blood is thus shunted from less essential organs preserving flow to the heart and brain. Sympathetic stimulation also causes venoconstriction accelerating venous return to the central circulation. Through their metabolic effects, catecholamine secretion contributes to stress induced hyperglycemia, a common problem during critical illness. The renin angiotensin system is activated resulting in the release of angiotensin-II (AT-II), another potent vasoconstrictor and stimulus for aldosterone secretion. Aldosterone promotes salt and water conservation at the level of the distal renal tubule in an attempt to preserve intravascular volume. It also regulates acid-base and potassium homeostasis. Glucagon is produced by the pancreatic alpha islet cells and, unlike insulin, has a catabolic role. Release of many of these substances also leads to decreased levels of circulating insulin. The resultant catabolic state characterized by insulin resistance, hyperglycemia, lipolysis, free fatty acid formation, ketogenesis, erosion of lean body mass and negative nitrogen balance may last for weeks to months.
Classification
Shubin and Weil’s classic paper distinguished the various forms of shock with respect to cardiovascular parameters [10]. Four categories of inadequate systemic perfusion were described: (a) hypovolemic, (b) obstructive, (c) cardiogenic, and (d) distributive. Although new etiologies of shock (e.g., adrenal insufficiency of critical illness) have recently received significant attention, they are easily placed into one of these physiologic descriptions.
Table 157.1 Classification of Shocka | ||||||||||||||||||||||||||||||||||||||||||||||||||||||||||||
---|---|---|---|---|---|---|---|---|---|---|---|---|---|---|---|---|---|---|---|---|---|---|---|---|---|---|---|---|---|---|---|---|---|---|---|---|---|---|---|---|---|---|---|---|---|---|---|---|---|---|---|---|---|---|---|---|---|---|---|---|
|
Hypovolemic Shock
Hypovolemic shock is the most common form of shock. Almost all forms include some component of hypovolemia as a result of decreased intravascular volume or “preload.” The sympathetic response to reduced preload is arterial vasoconstriction, diverting blood from the splanchnic viscera, skin, and skeletal muscle. Physical findings include cold clammy skin, tachypnea, tachycardia, and low urinary output, all a result of either hypovolemia or compensatory mechanisms.
Hypovolemic shock is stratified into four classes based on the degree of circulating volume loss (Table 157.1). It is important to recognize that significant blood volume may be lost in the absence of any clinical signs. Compensatory mechanisms allow systemic blood pressure to be maintained and a well-compensated patient may display tachycardia as the only objective clinical abnormality, even with a blood volume loss of up to 30%. Hypovolemic shock may be further subclassified as either hemorrhagic or nonhemorrhagic. Hemorrhagic shock may be visibly apparent (external blood loss from traumatic injury) or occult (chronic gastrointestinal hemorrhage). Emphasis on hemorrhage control rather than simply volume replacement is an essential difference in the management of hemorrhagic shock [11,12]. Nonhemorrhagic hypovolemic shock is seen in a number of pathologic states and may be caused by absolute loss of total body fluid volume and/or migration of acellular fluid from the intravascular to the interstitial compartment (third spacing). Third spacing of fluid occurs predictably in severe illnesses such as pancreatitis, small bowel obstruction, and burns. Volume depletion may also occur as a consequence of uncompensated gastrointestinal, urinary, or evaporative losses. It is imperative that the intensivists focus on resuscitation of the patient’s intravascular volume as opposed to total body volume. Failure to do so will uniformly result in under-resuscitation and poor patient outcome.
Obstructive Shock
Obstructive forms of shock are those in which the underlying pathology is a mechanical obstruction to normal cardiac output (CO) with a resulting diminution in systemic perfusion. Cardiac tamponade is an example of obstructive shock. A small amount of fluid (usually less than 200 mL) within a noncompliant pericardium may produce significant myocardial compression [13]. Clinical signs of tamponade include jugular venous distention and a central venous pressure (CVP) waveform
demonstrating a rapid “x” descent and a blunted “y” descent due to inability of the heart to fill during diastole. Pulsus paradoxus, an exaggerated fluctuation in arterial pressure caused by changes in intrathoracic pressure during respiration, may be present. Formal echocardiography is helpful in making the diagnosis although recent advances in the use of bedside ultrasonography by noncardiologists have demonstrated excellent sensitivity and rapid performance of the examination [14].
demonstrating a rapid “x” descent and a blunted “y” descent due to inability of the heart to fill during diastole. Pulsus paradoxus, an exaggerated fluctuation in arterial pressure caused by changes in intrathoracic pressure during respiration, may be present. Formal echocardiography is helpful in making the diagnosis although recent advances in the use of bedside ultrasonography by noncardiologists have demonstrated excellent sensitivity and rapid performance of the examination [14].
Pulmonary venous thromboembolism is another example of obstructive shock and may present as profound circulatory collapse. CO is restricted either by mechanical obstruction of the pulmonary arterial tree or by pulmonary hypertension induced by release of secondary mediators. Additional findings include elevated CVP and pulmonary hypertension, but normal pulmonary artery occlusion pressure (PAOP). Through similar mechanisms, venous air embolism can completely obstruct pulmonary arterial blood flow, with ensuing cardiac arrest. Central hemodynamics mimic those of pulmonary embolism. Although numerous causes exist, of greatest concern are the placement and removal of central venous catheters and surgical procedures in which the operative site is more than 5 cm above the right atrium [15]. Venous air embolism is diagnosed by auscultation of the classic “mill wheel” heart murmur. Immediate placement of the patient in a head-down, left lateral decubitus position is advocated, as are attempts to aspirate air from the right ventricle through a central venous catheter.
Finally, tension pneumothorax may cause shock through obstruction of venous return. Elevated intrapleural pressure collapses intrathoracic veins resulting in inadequate venous filling. Tension pneumothorax should be diagnosed by physical examination and not by radiography. Needle decompression often restores venous filling sufficiently until a thoracostomy tube can be placed.
Cardiogenic Shock
In cardiogenic shock, the underlying defect is primary ventricular pump failure, the most common cause of coronary artery disease related mortality. The foundations of ventricular failure include (a) myocardial infarction with loss of myocardium, (b) reduced contractility (cardiomyopathy), (c) ventricular outflow obstruction (aortic stenosis or dissection), (d) ventricular filling anomalies (atrial myxoma, mitral stenosis), (e) acute valvular failure (aortic or mitral regurgitation), (f) cardiac dysrhythmias, and (g) ventriculoseptal defects. Most often, cardiogenic shock is a direct or indirect consequence of acute myocardial infarction.
Cardiogenic shock due to left ventricular infarction suggests that more than 40% of the left ventricle is involved [16]. On physical examination, signs of peripheral vasoconstriction are evident and oliguria is common. The typical hemodynamic profile includes systemic hypotension with decreased CO and elevated PAOP. Physical examination findings of pulmonary and peripheral edema as well as hepatomegaly may suggest volume overload, but are commonly due to third spacing of fluid due to shock with relative intravascular volume depletion being present. In such situations, hemodynamic monitoring using echocardiography or a volumetric pulmonary artery catheter may provide additional diagnostic information clarifying the patient’s true volume status.
Right ventricular dysfunction as a consequence of inferior wall myocardial infarction carries a better prognosis than left-sided failure. Diagnosis may be suggested by elevated right ventricular diastolic pressure with decreased pulmonary artery pressure [17]. Hypotension caused by right-sided heart failure must be distinguished from left-sided failure because of the significant differences in their management. Shock from right-sided failure is corrected by volume resuscitation to maintain right ventricular preload while left-sided failure is treated by volume restriction to reduce myocardial work. If inotropes are indicated, agents that do not increase pulmonary vascular resistance should be chosen [18].
Dysrhythmias are another source of cardiogenic shock. In addition to malignant dysrhythmias, such as ventricular fibrillation, atrial dysrhythmias such as atrial fibrillation or flutter as well as supraventricular tachycardia are common in the critically ill and may result in shortened diastolic filling time with a profound decrease in CO.
Distributive Shock
The classic hemodynamic profile of septic shock (high CO and systemic hypotension) has prompted some clinicians to institute antimicrobial therapy and search for an infectious source in any patient who exhibits these cardiac parameters. Such hyperdynamic patterns, however, are seen in non-infectious conditions as well including anaphylaxis, spinal cord injury, and severe liver dysfunction. The term distributive shock, rather than septic shock, is therefore used to account for these dissimilar diseases with a common hemodynamic picture.
The management of septic shock remains a major challenge to the intensivist [1,2,3]. A milieu of inflammatory cytokines, bacterial factors, and complement and coagulation activation combine to induce the complex hemodynamic pattern characteristic of septic shock. In most forms of shock, illness leads to a low CO state with elevated systemic vascular resistance (SVR) and reduced mixed venous oxygen saturation (SvO2). Early septic shock, however, is manifested by normal-to-low cardiac filling pressures, increased CO, decreased SVR, and increased SvO2 [19]. Despite elevated systemic blood flow and oxygen delivery (DO2), abnormalities exist in tissue oxygen extraction at the cellular level, perhaps through disruption of normal mitochondrial metabolic pathways [20,21]. Sepsis-induced myocardial depression may be demonstrated through decreased ejection fraction, right ventricular dysfunction, and left ventricular dilation. In the later stages of septic shock, cardiac function deteriorates with the patient’s hemodynamic status mimicking that of cardiogenic shock with decreased CO and increased SVR [22].
Anaphylaxis represents another form of distributive shock in which histamine-mediated vasodilatation occurs. The most common causes are medications, insect envenomations, blood products, radiographic contrast media, and food allergies [23]. Reactions severe enough to result in shock occur shortly after exposure to the offending agent. Physical findings include a dermatologic reaction (erythema, urticaria) and obstructive respiratory processes. Occasionally, the reaction is severe enough to produce shock through myocardial depression.
Neurogenic shock, another form of distributive shock, occurs as a result of upper thoracic spinal cord injury with hypotension, bradycardia, and warm, dry skin due to loss of sympathetic vascular tone. Although euvolemic, patients demonstrate relative hypovolemia due to vasodilatation of the intravascular space. If hypotension does not respond to volume resuscitation, it may be treated with vasopressors and any bradycardia may be corrected with atropine. In the trauma patient, hemorrhage should always be excluded before attributing shock to a neurogenic source [24].
Over the last decade, endocrine insufficiency as a result of critical illness has been recognized as an underappreciated cause of distributive shock. This relative adrenal insufficiency may worsen the impact of the various shock states as the patient is unable to respond appropriately to the stress of their critical illness [25,26]. Corticosteroid supplementation in such
patients can significantly improve systemic perfusion as well as reduce the patient’s requirement for vasopressor support.
patients can significantly improve systemic perfusion as well as reduce the patient’s requirement for vasopressor support.
Physiologic Monitoring
Vital sign derangements are typically the first indication that a shock state is present. Normalization of such parameters signifies that the patient is appropriately responding to resuscitative therapy. Physiologic monitoring is thus essential to both the diagnosis and management of shock. Such monitoring typically begins with the use of routine vital signs, but may progress to the application of invasive monitoring techniques.
Vital Signs
The diagnosis of shock was originally based on abnormalities in a patient’s vital signs. Until the late 1960s, the presence of tachycardia and hypotension was considered synonymous with shock. Over time, it became apparent that normalization of heart rate, blood pressure, temperature, and urinary output was not necessarily sufficient to reverse a patient’s shock state. Critically ill patients continued to have a high incidence of multiple organ failure and mortality despite seemingly adequate resuscitation based on restoration of vital signs to “normal.” Shock is therefore defined by the adequacy of end-organ perfusion rather than derangements in vital signs alone. Nevertheless, these physiologic parameters remain the foundation for the initial recognition that shock is present.
Heart Rate
Alterations in heart rate are common during shock. Tachycardia is most common and is usually a direct effect of intravascular volume loss in where heart rate increases to maintain adequate CO and DO2 to tissues. These increases may become pathologic if inadequate diastolic filling time results in decreased stroke volume. Tachycardia can be used to predict the presence of intravascular volume depletion and its resolution to suggest volume resuscitation adequacy [27]. Decreased heart rate, in response to a volume challenge, can be a simple and useful test for diagnosing hypovolemia.
Bradycardia is usually representative of severe physiologic derangement and impending cardiovascular collapse. Its presence in a critically ill patient demands immediate attention. Patients receiving beta-blocker therapy or with high spinal cord injuries or pacemakers may not be able to increase their heart rate and compensate for their shock. Patients with an inappropriately low heart rate and inadequate CO will benefit from increasing heart rate by withholding beta-blocker therapy, use of chronotropic medications, or reprogramming their pacemakers to a higher rate.
Blood Pressure
Hypertension is an uncommon finding in shock. Patients are typically hypotensive due to the presence of hypovolemia, decreased cardiac contractility, or systemic vasodilatation. Normotension should be restored as quickly as possible to improve tissue perfusion and oxygen delivery at the cellular level. Blood pressure may be measured either noninvasively or invasively. Both techniques are subject to certain mechanical and physiologic measurement errors, or “dynamic response artifacts,” that can result in inappropriate therapy if unrecognized by the clinician [28]. Because of these intrinsic monitoring errors, systolic blood pressure (SBP) and diastolic blood pressure (DBP) measurements may vary widely from one measurement technique to another. The mean arterial pressure (MAP), however, will remain fairly consistent regardless of the measurement method and any artifact present. As a result, MAP should be used to titrate resuscitative therapies rather than SBP or DBP. MAP is calculated as

Temperature
Patient temperature, although not indicative of either the presence or absence of shock, may help define the cause and can have significant prognostic value [29,30]. The presence of hypothermia (core body temperature less than 96.8°F or 36.0°C) suggests severe physiologic derangement and has a significant impact on patient survival [31]. Hypothermia places the patient at risk for cardiac dysrhythmias, acute renal failure, and refractory coagulopathy [32]. Although hypothermia reduces metabolic activity of the body, rewarming significantly increases global metabolic demands and oxygen consumption ([V with dot above]O2). Such demands may exceed the patient’s capacity to deliver oxygen to the cells, resulting in an oxygen transport imbalance. Care must be taken to ensure adequate DO2 and tissue perfusion during rewarming. Because of its significant morbidity and mortality, nontherapeutic hypothermia should be avoided or rapidly corrected in most critically ill patients [29,30].
Urine Output
Inadequate renal blood flow results in decreased urinary output. Oliguria is one of the earliest signs of inadequate perfusion at the tissue level. Worsening renal function is an important indicator of the presence of shock. Decreases in urine output as a result of hypovolemia are seen before changes in heart rate or blood pressure (Table 157.1). Improvements in urine volume in response to fluid loading can guide shock resuscitation as long as confounding factors are not present (e.g., diabetes insipidus, diabetic ketoacidosis, and diuretic therapy).
Pulse Oximetry
Technologic advances in the 1970s and 1980s led to the widespread introduction of pulse oximetry as the “fifth” vital sign [33]. Pulse oximetry is now routinely used in the critically ill as a noninvasive method of continuously monitoring arterial oxygen saturation. This addition to the traditional four vital signs serves two purposes. First, it provides an early warning of hypoxemia, allowing corrective interventions to be made. Second, it can be used as an endpoint in the resuscitation of patients and in the assessment of oxygen transport balance.
Hemodynamic Monitoring
In 1970, Swan and Ganz introduced the flow-directed pulmonary artery catheter, allowing clinicians to measure pulmonary artery pressures at the bedside [34]. In 1972, addition of a temperature thermistor provided the ability to calculate CO. These advancements provided clinicians with the ability to assess a variety of new hemodynamic parameters evaluating patient preload, contractility, and afterload. In the 1980s, continuous mixed venous oximetry was added as the importance of DO2, [V with dot above]O2, and oxygen transport balance in the diagnosis and management of the shock states became clear. By the early 1990s, catheters capable of calculating right ventricular volumes became available, further improving preload assessment. Current pulmonary artery catheters continuously assess hemodynamic and oxygen transport variables providing the clinician with minute-by-minute assessments of cardiopulmonary function by which to guide resuscitation. Although pulmonary artery catheterization is performed with much less frequency than in years past, it remains an important
monitoring technology for the most critically ill patients with shock and has recently been demonstrated to improve patient outcome when used in a goal-directed fashion [35,36]. A variety of other hemodynamic monitoring techniques have been developed including arterial pressure wave contour analysis, esophageal Doppler, and transesophageal echocardiography among others. Regardless of the method by which hemodynamic data is obtained, a thorough understanding of the available hemodynamic and oxygenation variables is essential if resuscitative therapy is to improve patient outcome from shock (Tables 157.2 and 157.3) [37].
monitoring technology for the most critically ill patients with shock and has recently been demonstrated to improve patient outcome when used in a goal-directed fashion [35,36]. A variety of other hemodynamic monitoring techniques have been developed including arterial pressure wave contour analysis, esophageal Doppler, and transesophageal echocardiography among others. Regardless of the method by which hemodynamic data is obtained, a thorough understanding of the available hemodynamic and oxygenation variables is essential if resuscitative therapy is to improve patient outcome from shock (Tables 157.2 and 157.3) [37].
Pressure and Pressure-Derived Variables
Pressure variables form the foundation for physiologic monitoring in shock assessment. It is important to recognize, however, that the absolute value of any single pressure variable is not as important as the trend, calculated variables, and perfusion pressures that may be identified using this pressure.
Table 157.2 Hemodynamic Variables | |||||||||||||||||||||||||||||||||||||||||||||||||||||||||||||||||||||||||||||||||||||||
---|---|---|---|---|---|---|---|---|---|---|---|---|---|---|---|---|---|---|---|---|---|---|---|---|---|---|---|---|---|---|---|---|---|---|---|---|---|---|---|---|---|---|---|---|---|---|---|---|---|---|---|---|---|---|---|---|---|---|---|---|---|---|---|---|---|---|---|---|---|---|---|---|---|---|---|---|---|---|---|---|---|---|---|---|---|---|---|
|
Table 157.3 Oxygenation Variables | ||||||||||||||||||||||||||||||||||||||||||||||||
---|---|---|---|---|---|---|---|---|---|---|---|---|---|---|---|---|---|---|---|---|---|---|---|---|---|---|---|---|---|---|---|---|---|---|---|---|---|---|---|---|---|---|---|---|---|---|---|---|
|
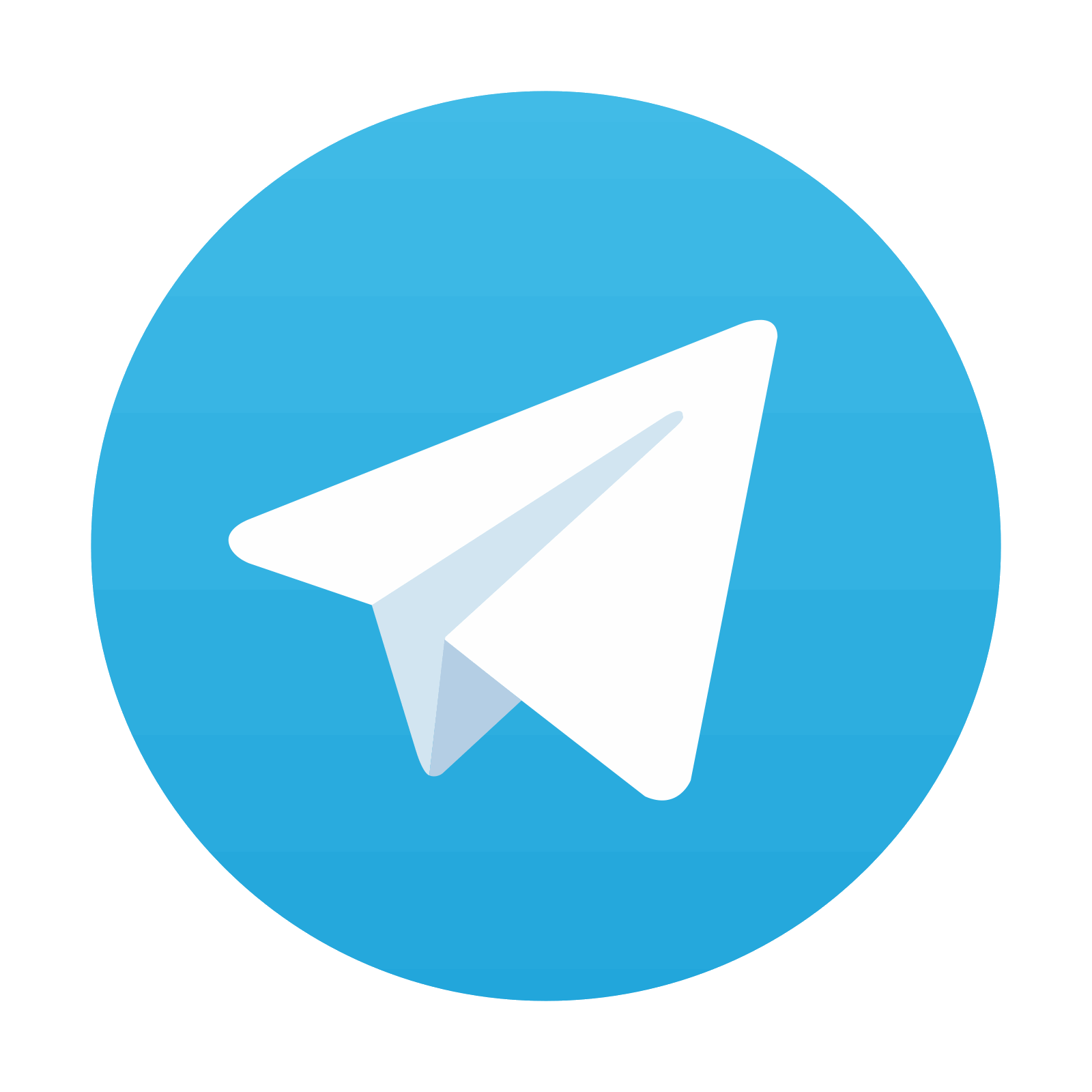
Stay updated, free articles. Join our Telegram channel
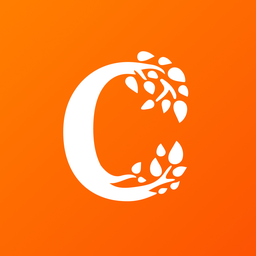
Full access? Get Clinical Tree
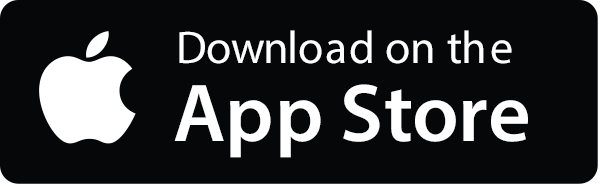
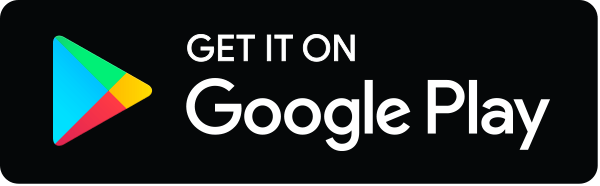
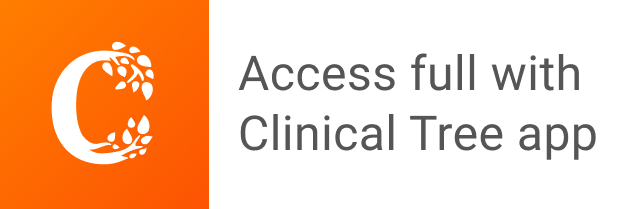