KEY POINTS
The definition of sepsis is two or more systemic inflammatory response criteria plus a known or suspected infection.
Severe sepsis is sepsis with acute organ dysfunction. Acute organ dysfunction can manifest in any organ, and frequently manifests clinically as shock, respiratory failure, acute kidney injury, hematologic or metabolic disturbances, or neurologic decline. Septic shock is a form of severe sepsis where the organ dysfunction involves the cardiovascular system.
Sepsis results in a complex set of interactions between the inciting microbes and the host immune response, which triggers the inflammatory cascade and coagulation pathway.
Management of sepsis patients involves early infection recognition, source control, fluid therapy, antibiotics, and hemodynamic supportive care. Early goal-directed therapy is the term for current early fluid resuscitation strategies that target central venous or mixed venous oxygen saturation.
The most common parameters used in monitoring septic patients are pulse oximetry, arterial blood pressure, central venous pressure, central venous or mixed venous oxygen saturation, and blood lactate. Other parameters that may guide therapy include cardiac output, systemic vascular resistance, and extravascular lung water. Each of these parameters is complementary and may assist in both the early and late management of sepsis, organ dysfunction, and shock.
Sepsis care bundles have become an integral part of the “Surviving Sepsis Campaign,” which aimed to improve survival from severe sepsis. These multifaceted interventions facilitate compliance with evidence-based guideline recommendations by creating two “bundles” that are sequentially completed at 6 and 24 hours.
DEFINITIONS AND EPIDEMIOLOGY
Sepsis has been a life-threatening medical condition since the first steps in evolution. Antimalarial compounds were prescribed for fever in China as early as 2735 bc and Hippocrates recognized the anti-infective properties of wine and vinegar around 400 bc. The basic premise of infection and immune response were recognized from the time that Marcus Terentius Varro in 100 bc noted that “small creatures invisible to the eye, fill the atmosphere, and breathed through the nose cause dangerous diseases.” These early concepts carried through the Black Death plague of the middle ages and Janssen’s invention of the microscope, to Louis Pasteur’s germ therapy, and on to Ignaz Semmelweis and Joseph Lister’s antisepsis practices. At the turn of the last century, William Osler recognized that “the patient appears to die from the body’s response to infection rather than from it.”
Despite clear advances in understanding infection and the immune response, sepsis was not recognized as a specific medical entity deserving of recognition and focused study until the 1970s. In order to facilitate the study of sepsis, in 1992 the American College of Chest Physicians (ACCP) and the Society of Critical Care Medicine (SCCM) jointly developed a set of consensus definitions for sepsis and related disorders (Table 64-1).1 In so doing, the ACCP/SCCM consensus definitions immediately created a clinically applicable definition that may be used at the bedside and can be used equally to identify patients for clinical trials and test new therapeutics. Although the ACCP/SCCM consensus definition is imperfect, suffering from both a lack of sensitivity and specificity, it has transformed our understanding of sepsis epidemiology and pathogenesis, and it has permitted the successful testing of novel therapies for this condition.
Definition of Sepsis and Related Disorders
Disease State | Definition | Mortality |
---|---|---|
Sepsis | Infection + at least two SIRS criteria | Determined by the underlying condition |
Severe sepsis | Sepsis with acute organ dysfunction | 25%-40% |
Septic shock | Sepsis with refractory hypotension despite adequate fluid loading (vasoplegia) | 40%-80% |
An underlying principle of the ACCP/SCCM definition is that clinical sepsis represents the immune response to infection. That principle is the foundation for defining sepsis as the intersection between the systemic inflammatory response syndrome (SIRS) criteria and infection (Fig. 64-1). The SIRS criteria are not specific for sepsis, and may be present in a high proportion of acutely ill and hospitalized patients. However, when at least two criteria are present and related to an infection, sepsis is diagnosed (Table 64-1). Making the diagnosis of sepsis is the foundation for understanding a variety of related processes that stem from the same host immune response. Acute organ dysfunction is the hallmark of a more lethal form of sepsis: those patients who have sepsis and acute organ dysfunction are diagnosed with severe sepsis. Acute organ dysfunction, to be discussed in detail later, may occur in any organ of the body, and frequently manifests clinically as shock, respiratory failure, acute kidney injury, or other acute conditions. The recognition of severe sepsis is important as it portends a worse prognosis and also directly influences medical therapy. The most severe form of sepsis is septic shock, defined as refractory hypotension despite fluid resuscitation. Septic shock almost invariably associates with other acute organ dysfunction and carries the highest mortality of all forms of sepsis. However, even among the critically ill patients with septic shock, prognosis is influenced by the occurrence of other acute organ dysfunction and the presence of chronic comorbid medical conditions.
While these definitions have served to permit studies of sepsis epidemiology and enrollment of subjects into clinical trials of successful new therapies, they have been criticized for being both insensitive and not specific for sepsis. These limitations led to another consensus conference with representatives from the SCCM, ACCP, the European Society of Intensive Care Medicine, the American Thoracic Society, and the Surgical Infection Society. Because a superior clinical definition was not apparent, the conference retained the original definition and developed two new and important concepts. The first concept is that the SIRS criteria are only a few signs or symptoms that may indicate sepsis, and while a new version of the SIRS criteria was not proposed, it was recognized that delaying a diagnosis of sepsis when the traditional four SIRS criteria are absent is ultimately a disservice to an acute ill patient. Additional potential criteria were proposed, including heterogeneous clinical and laboratory manifestations of systemic illness (alterations in mental status or hyperglycemia), infection (eg, elevated C-reactive protein or procalcitonin), and even sepsis-related organ dysfunction (central venous hypoxia, coagulopathy, oliguria, mottling). The second important concept put forth was the necessity to characterize the “stage” of illness for sepsis patients, as is done with cancer or heart disease. They proposed this as the PIRO model—Predisposition, Insult/Infection, Response, Organ dysfunction—which has since been validated as a tool for prognosticating outcomes with sepsis.2-4
Sepsis is classically considered a disease related to a gram-negative bacterial infection because of the original pathophysiological understanding linked to endotoxin (see the section Pathophysiology). However, recent epidemiological studies show that, when analyzing sepsis by identified organisms, gram-positive bacteria became the predominant cause of sepsis by the mid-1980s.5 This increase is multifactorial, including temporal changes in antibiotic pressure, changing patterns of health care delivery (eg, increasing use of invasive procedures) and patient populations (eg, growth of immunocompromised populations), and increasing rates of nosocomial sepsis overall. These factors are in addition to concerns that gram-positive organisms may offer differences in virulence due to cell wall constituents and exotoxins, as evidenced by toxic shock syndrome. Studies of inciting organisms with sepsis are hampered by our limited ability to convincingly identify the causative organism in more than 50% to 75% of cases, even in those with septic shock.6,7 However, although bacterial causes of sepsis predominate, fungal infections causing sepsis show the greatest rate of increase for any identified organism, far exceeding the rates of increase with any other pathogen.5 Other organisms may also elicit a sepsis response, such as parasites, Pneumocystis, and acute viral infections.
The sources of sepsis vary according to the type (severity) of sepsis. Sepsis overall is dominated by respiratory infections, accounting for approximately 40% to 50% of cases, with genitourinary (30%) and gastrointestinal (25%) infections being next most common.6 For patients with septic shock, respiratory infections still predominate (40%), but gastrointestinal (30%) and genitourinary (15%) infections switch places, in part because gastrointestinal infections are more frequently severe compared to genitourinary infections.7 The remainders of infections are identified from miscellaneous sources that vary depending on the study, but invariably include skin and soft tissue infections, bone and joint infections, central nervous system infections, and primary bacteremia. Importantly, the sources of nosocomial sepsis also differ, with a higher proportion of surgical site infections and catheter-related infections (vascular or urinary catheters most commonly), although respiratory infections remain the dominant source even in these patients.
As may be expected, certain factors may predispose to the development of sepsis. Some factors may be manipulated or controlled, whereas others, such as age, are impossible to influence directly. Age is among the most potent predictors of the risk for sepsis, with sepsis risk increasing exponentially after the age of 60 years.8-10 Many chronic comorbid medical conditions alter the risk for developing sepsis, particularly those that require frequent exposure to the health care system or are associated with altered immunity. For example, chronic immunosuppression increases the risk of both infection and sepsis, and this is evidenced by high rates of sepsis in patients with cancer, diabetes, and HIV disease.6,11,12 Remarkably, patients with cancer have among the highest population-adjusted rates for sepsis—similarly high to that for patients with HIV and exceeding estimated rates with chronic lung disease, heart disease, and diabetes.11
Sepsis incidence and mortality is also influenced by regional, seasonal, and cultural factors. Sepsis rates are lowest in the fall and highest in the winter, with the greatest increase in cases due to respiratory infection. Regional differences in sepsis incidence are also apparent, with higher rates in the Northeastern United States and the greatest seasonal changes in rates between the fall and winter seasons also seen in the Northeast.13 Both the seasonal and regional variation may relate to rates of viral infections, which closely track cases of respiratory sepsis. Infection and sepsis rates are affected by myriad factors in the developing world, including climatic conditions, and although data outside of well-developed nations are sparse, the frequency of infectious diseases makes sepsis a likely culprit for the leading cause of death worldwide.
PATHOPHYSIOLOGY
Over the years, a considerable amount has changed in the way we think about sepsis pathophysiology. Initially considered a syndrome of exaggerated inflammation, sepsis is now recognized as a complex set of interactions between the inciting microbes and the host immune response, which triggers the inflammatory cascade and coagulation pathway (Fig. 64-2).
Different microorganisms have devised their own unique method of attack. For example, gram-positive bacteria such as Staphylococcus aureus or Streptococcal pyogenes have a special exotoxin than can lead to a particular type of sepsis called toxic shock syndrome (TSS). The exotoxin is a superantigen and it bridges the T-cell antigen receptor (TCR) to bind to major histocompatibility complex (MHC) causing massive T-cell stimulation.14 Gram-negative bacteria, such as Escherichia coli, have a complex lipid called lipopolysaccharide (LPS) in its membrane barrier, which activates the innate host immune response.15 Similar to bacteria, viruses have a unique molecular pattern that is recognized and identified by various host Toll-like receptors (TLR). Additionally, an immunosuppressed host can fall prey to fungal infections such as Candida albicans, an opportunistic pathogen that develops as a consequence of an inadequate immune host response. It is able to display various morphologies from a unicellular form (eg, hyphae, pseudohyphae, or chlamydospores) and can threaten an altered immune host.16 As mentioned above, sepsis can occur with any of these microorganisms and each may initiate a host immune response resulting in a complex inflammatory and coagulation cascade.
Host Immune Response: The host’s response to an infection depends on both the innate and acquired host immune system. Certain patients are more susceptible to sepsis due to their inability to mount a normal immune response. The first line of host defense is the epithelium where the pathogen can break through and enter the host. The innate and acquired immune systems orchestrate their various roles in an attempt to eradicate the threatening pathogen.
Microbes that breach the epithelium are recognized by macrophages with “pattern recognition receptors,” which are TLRs. The TLR triggers an intracellular signaling cascade, such as nuclear factor-κB (NF-κB), which activates phagocytic macrophages.17 The inflammatory response instructs the innate immune system to provide reinforcements. The innate immune system responds rapidly and mobilizes quickly. The adaptive immune response takes days to weeks for T and B cells to identify and replicate antigen specific receptors.
Inflammation: An infectious insult can trigger a cascade of innate humoral, cytokine, and complement responses in a host, which can cause cellular dysfunction and tissue damage, potentially leading to multiorgan dysfunction and death.
Cytokines: Many cytokines serve as messengers to the host immune system, promoting an increased inflammatory response.7 Proinflammatory cytokines (eg, interleukin-1 [IL-1], IL-2, IL-6, IL-8, IL-10, interferon gamma [INF-γ], and platelet-activating factor [PAF]) conduct a myriad of biological pathways that are known as the systemic inflammatory response syndrome (SIRS).17 Chemokines are a family of cytokines that has the capacity to regulate leukocyte migration and are crucial to the organization and structure of cell distribution in the inflammatory response.17 During inflammation, neutrophils and macrophages produce large amounts of reactive oxygen species (ROS) and reactive nitrogen species (RNS), which allows the neutrophils and macrophages to kill microorganisms. ROS can also cause oxygen cell damage of the endothelium.18 The imbalance between production and adequate removal of ROS can result in leukocyte and platelet adhesion, changes in vascular tone and vascular permeability. ROS and RNS can also contribute to mitochondrial dysfunction.18 Oxygen delivery is impaired in sepsis as is the cell’s ability to utilize oxygen coining the phrase “cytopathic hypoxia.”19 Depletion of mitochondrial ATP and impaired oxidative phosphorylation has been demonstrated in animal models. Mitochondrial dysfunction in skeletal muscle and liver has been associated with poor outcomes in septic patients. Mitochondria are the main cellular oxygen consumers and their dysfunction in sepsis related to multiorgan dysfunction is an area of research interest.
The early inflammatory response is followed by an anti-inflammatory response by mediators such as IL-10, IL-1 receptor antagonist (IL-1ra), and soluble tumor necrosis factor (TNF) receptor in order to establish homeostasis.20 In addition, T-helper cells are able to change their production of type 1 proinflammatory to type 2 anti-inflammatory cytokines. Organ dysfunction and mortality often occur during this period of hypoimmunity. This hypoimmune state can also prolong the host ability to recover.
Complement: Complement activation includes three major pathways as part of the innate immune system. All three pathways lead to C3, which starts the cascade of cleavage products like C3a, C3b, C5a, C5b, and C5b-C9 lytic membrane attack complex where complement molecules create a pathway for fluid to shift from the extracellular to intracellular space resulting in cell wall lysis of the pathogen.7,21
Coagulation Imbalance: In recent years, it has been discovered that the coagulation system acts in concert with the inflammatory cascade in the pathophysiology of sepsis.22 The endothelium is the protective barrier for the blood vessel. In sepsis, their integrity is compromised. Damage to the endothelium causes hemorrhage and increases permeability,23 which is a key factor to the pathogenesis of severe sepsis.
Endothelial cells maintain systemic blood pressure and flow to organs. The human body contains about 1013 endothelial cells, an area of 4000 to 7000 m2.18 Damage to the endothelial cells cause tissue edema by increasing microvascular permeability resulting in fluid loss into the interstitial space, which can lead to hypovolemia, arterial hypoxemia, impaired gas exchange, and impaired tissue oxygen distribution.24 Endothelial damage ignites the coagulation tissue factor cascade as well.25 The protein C pathway serves as an anticoagulant system, promoting fibrinolysis by inhibiting thrombosis and inflammation.25 Thrombin binds to thrombomodulin at the endothelial protein C receptor (EPCR) on the endothelium, resulting in a complex that rapidly activates protein C, which binds to protein S, ultimately inactivating factors Va and VIIIa. Activated protein C (APC) is decreased in sepsis by impaired synthesis, consumption, and degradation.25
ORGAN DYSFUNCTION IN SEPSIS
Septic patients often have an elevated troponin level and it was unclear at first if this represented irreversible myocardial injury or reversible myocardial depression.26 However, previous studies found that coronary blood flow did not differ between septic shock and healthy patients. Additionally, coronary blood flow did not change between septic shock patients who developed myocardial depression and those who did not.26
There are multiple mechanisms contributing to myocardial dysfunction in sepsis. The proinflammatory mediators already discussed such as tumor necrosis factor-alpha (TNF-α), interleukin (IL), and nitric oxide (NO) depress cardiac myocyte contractility.27 Excess NO production by vascular endothelial cells causes myocardial depression.27 Changes in volume status, downregulated β receptors, reduced calcium from the sarcoplasmic reticulum, and downregulated signaling pathways all contribute to septic cardiac dysfunction.27 Dysfunction can be seen early in sepsis and an echocardiogram may reveal systolic, diastolic, and/or biventricular dysfunction. Myocardial depression can also exist in a hyperdynamic state. Cardiac function usually recovers between 7 and 10 days after onset.28
In contrast to cardiogenic or hypovolemic shock, septic shock is a distributive shock state resulting in vessel dilatation instead of vasoconstriction during hypotension. NO is overproduced by inducible nitric oxide synthase (iNOS) found in arterial smooth muscle cells and endothelium.29 NO is released into circulation bound to hemoglobin. Overactive substances like NO decrease vascular tone by activating potassium channels and hyperpolarizing smooth muscle plasma membranes.30 This leads to the most common presentation of a septic patient with hyperdynamic cardiac output, hypotension, and low systemic vascular resistance.
Sepsis is a major risk factor foracute respiratory distress syndrome (ARDS), which is characterized by neutrophilic inflammation and increased pulmonary vascular permeability. Development of ARDS is associated with a high rate of morbidity and mortality in the range of 30% to 50%. ARDS can progress into a more fatal form known as acute respiratory distress syndrome (ARDS), which carries an even higher rate of fatality.31 The pooled mortality rate for ALI/ARDS in several locations around the world exceeds 40%.32
There are two barriers that make up the alveolar-capillary barrier: the microvascular endothelium and the alveolar epithelium. In the acute phase, there is denudation of the basement membrane and sloughing off of the bronchial and epithelial cells. Neutrophils adhere to the injured capillary endothelium and marginate into the air space interstitium. In the air space, alveolar macrophages attack by secreting TNF-α, IL-1, IL-6, IL-8, and IL-10, which signal chemotaxin and neutrophils to attack. Neutrophils bombard the pathogen by releasing proteases, leukotrienes, and platelet-activating factor (PAF).33 Many research groups are actively seeking accurate biomarkers for both diagnosis and prognosis in patients with ARDS. Investigators have identified certain inflammatory mediators such as IL-1β and TNF-α, which have been found in the distal airways in ARDS patients.34-36 IL-8, plasminogen activator inhibitor-1, and protein C of the coagulation system have also been suggested to be predictive of clinical outcomes in this patient population.37 Markers of both endothelial lung injury, such as Von Willebrand factor, and epithelial lung injury, such as receptor for advanced glycation end products (RAGE) and surfactant protein-D (SP-D), are being studied as potential markers for disease severity.38
In sepsis, the gastrointestinal track becomes hypoperfused, which can result in gut ischemia, but in addition reperfusion of the gut can ignite proinflammatory mediators, which can cause intestinal permeability, ileus, and bacterial translocation.39 Bacterial translocation is the passage of endogenous bacterial flora endotoxins across mucosal barriers. Ileus, defined as intestinal dysmotility, causes an accumulation of bacteria of the stomach and small intestine that predisposes to bacterial translocation and aspiration pneumonia. The pathogen can also sense the alteration in the host and enhance their virulence phenotype.40 Therefore, ileus perpetuates the infectious and proinflammatory state of sepsis contributing to multiorgan failure.39
Cholestasis develops from inflammation of cytokines within hepatocytes. The proinflammatory cascade represses hepatobiliary transporter gene expression. Hepatobiliary transport system is crucial for the uptake and excretion of bile acids41 and so disruption in this process can result in sepsis-associated cholestasis.
The hypoperfusion state of sepsis with systemic vasodilatation can also cause poor perfusion to the kidney, resulting in acute kidney injury (AKI). Fifty percent of AKI in the intensive care unit (ICU) is caused by sepsis and the incidence rises with the severity of sepsis.42 Twenty-three percent of patients with sepsis have AKI and 51% of patients with septic shock develop AKI.42 Renal hypoperfusion can occur even in the absence of severe hypotension, especially in high-risk patients with baseline renal dysfunction.42 Aggressive fluid resuscitation can cause capillary leaks that lead to tissue edema in the abdomen that can further impede blood flow to the kidneys.42 Decreased renal function has been associated with a 6.5-fold increase in odds of death.43,44 Those who require renal replacement therapy (RRT) have a mortality rate of 50% to 80%.45
IL-6 and TNF-α decrease iron in the blood due to stimulation of ferritin synthesis, resulting in a decrease tissue iron release and consequential fall in soluble transferring receptors, which are needed to stimulate erythroid growth.46 Inflammatory cytokines increase hepcidin expression, which causes decreased absorption of iron from the intestine and diverts iron to storage sites like the reticuloendothelial system (RES) and the liver. This causes a decline in serum iron concentrations and transferrin saturation, which results in decreased erythroid formation and shortened survival of red cells.47,48 Given that oxygen is transported by hemoglobin, decreased red blood cell production and cell life directly impact oxygen-carrying capacity to vital organs. This has a profound effect on oxygenation and perfusion, which can lead to multiorgan failure.
Endothelial cells and megakaryocytes, which are precursors to platelets, come from the same bone marrow progenitor cells. Also, they share the same transcriptional and gene expression pathways such as von Willebrand factor.49 There is a strong interplay of communication between endothelial cells and platelets. Platelets release signaling pathways to the endothelium through cytokines like IL-1, transforming growth factor (TGF), and platelet-derived growth factor (PDGF). Conversely, endothelial cells can inhibit or promote platelet activation through NO or PAF. Miscommunication between these cells can lead to thrombocytopenia, which has an incidence of 35% to 59% in septic patients.49
Seventy percent of patients with severe sepsis develop septic encephalopathy.50 It is the most common form of encephalopathy in ICU patients, associated with increased morbidity and mortality. The symptoms vary from mild confusion, agitation, and delirium to stupor and coma.51 Originally septic encephalopathy was thought to be due to the presence of microorganisms or toxins in the blood. However, microorganisms and toxins have not been isolated from many septic patients.50 The exact mechanism in septic encephalopathy in humans is unknown, although alterations in neurotransmitters and their receptors are being investigated. Chronic LPS exposure in hippocampal cells has been found to increase the hippocampus production of IL-1bβ, and IL-1β-dependent IL-6 levels, which effects the neuronal and synaptic function that could contribute significantly to cognitive disturbances.52 Altered iNOS expression disrupts glutamatergic neurotransmission, expression, and function leading to behavioral changes in rat models.51 Septic encephalopathy likely arises from brain injury from inflammatory mediators and the brain cells’ cytotoxic response to these mediators.50
Tight junctions between endothelial cells make up the blood-brain barrier, which regulates the uptake and efflux of nutrients, toxins, and metabolites in and out of the brain. Compromise to this highly regulated security system causes entry of inflammatory cells and toxic metabolites, which leads to neuronal tissue edema, limiting diffusion and oxygenation utilization.51 Astrocytes are important in inducing the blood-brain barrier properties and their damage will cause increased permeability. Astrocytes have receptors for inflammatory mediators. In human astrocyte cultures, recombinant human gamma interferon and IL-1β induce the formation of reactive oxygen intermediates that are toxic, allowing vulnerability to free radical injury and hypoxic injury. Damaged astrocytes will impair the regulation of local blood flow and the synaptic activity of neurons.50
It is well known that acute illness and injury results in insulin resistance and consequential hyperglycemia.53 Critical illness is associated with increases in many counterregulatory hormones (glucagon, epinephrine, growth hormone) and cytokines (TNF-α, IL-1) resulting in a sustained increase in plasma glucose despite hyperinsulinemia.54,55 Resultant hyperglycemia can have significant side effects such as impaired wound healing,56 vascular and endothelial dysfunction,57 and increased proteolysis.58 Intensive insulin therapy has been shown to beneficially affect innate immunity by preventing catabolism and lactic acidosis, exerting anti-inflammatory effects59-61 and protecting endothelial62 and hepatocyte mitochondrial function.63
Thyroid hormones regulate energy expenditure and orchestrate metabolism. Early in acute stress, triiodothyronine (T3) rapidly declines. Low T3 levels remain even after thyroid-stimulating hormone (TSH) normalizes, a condition called low T3 syndrome. Low T3 decreases the pulsatile release of TSH, causing low levels of thyroxine (T4).63
Hillenbrand reported that adipokines and resistin, produced by adipose tissue and macrophages respectively, contributed to insulin resistance in septic patients.64 The hypothalamic corticotropin-releasing hormone (CRH) stimulates the pituitary for release of adrenocorticotropic hormone (ACTH) and corticotropin, which trigger the adrenal cortex to produce cortisol. Cortisol levels are usually increased in the early phase of sepsis and cause an increase in the release of CRH and ACTH.63 Elevated cortisol shifts carbohydrate, protein, and fat metabolism to allow immediate energy availability to vital organs. Both systemic and neural pathways activate the hypothalamic-pituitary adrenal axis.65 Several studies have revealed that septic patients have elevated baseline cortisol levels and a lower cortisol response to ACTH simulation test causing a relative adrenal insufficiency.65 This relative adrenal insufficiency has been associated with an increased length of ICU and hospital stay.65
DIAGNOSIS, PROGNOSIS, AND MONITORING
Patients can present in a myriad of ways with sepsis, and thus clinicians must have a high index of suspicion for infections that may cause sepsis as well as for the condition itself. The most systematic way to diagnose sepsis is to determine the SIRS criteria on all patients. Many patients may have subtle findings and various combinations of the SIRS criteria, presenting with mild leukocytosis and tachypnea, or mild tachycardia and fever or often overlooked hypothermia. During the initial evaluation of the patient, the patient should be evaluated for the SIRS criteria and then clinically assessed for any evidence or suggestion of infection. Patients can present in profound septic shock with an occult infection. Severe sepsis can be easily missed on admission. Patients can be admitted to a general hospital floor and acutely decompensate, requiring emergent ICU transfer.
The first step in both diagnosing and managing a patient with sepsis is a complete history and physical examination. The vital signs provide important information on the systemic nature of the infection and the overall condition of the patient. Clinicians are like detectives, systematically evaluating the patient from head to toe to find symptoms and signs of infection and organ dysfunction. Starting from the head, the patient’s neurological status should be assessed. Is the patient alert and oriented or confused and agitated? Is the patient hypoactive or hyperactive, either of which may be signs of encephalopathy? In patients with preexisting cerebrovascular disease or dementia, sepsis may worsen baseline neurological function. Does the patient have nuchal rigidity secondary to meningitis? Orbital and oral examinations are also important. Patients may have subtle signs of oral candidiasis often seen in immunocompromised patients. Auscultation of the lungs may reveal rhonchi or crackles (suggesting pneumonia) or dullness to percussion (suggesting pleural effusion). The abdominal examination may reveal ascites, tenderness, or other physical findings indicative of abdominal infections. Cholecystitis and acute cholangitis may cause pain in the right upper quadrant, while pancreatitis may present similarly in the epigastrium. Diverticulitis, appendicitis, and peritonitis can present with diffuse abdominal pain. Also, the skin should not be forgotten for signs of erythema, rash, or skin breakdown, which could be entry points for infectious pathogens. Cellulitis in diabetic patients can cause sepsis and may indicate a polymicrobial infection. Necrotizing fasciitis can cause rapidly progressive sepsis and organ dysfunction starting with subtle skin findings, advancing to crepitus and myonecrosis within hours.
Every attempt should be made to locate and identify the infectious pathogen. This usually involves blood, urine, and respiratory cultures. Additional directed samples from suspected sources such as cerebrospinal fluid in suspected meningitis, pleural fluid from suspected empyema, bronchial alveolar lavage or bronchial brushings from respiratory bronchi, and ascitic fluid in suspected peritonitis may be warranted (see the section Source Control).
Other diagnostic studies include a complete white blood cell count with differential, a complete metabolic profile evaluating electrolytes, kidney, and liver function as well as a coagulation profile (platelets, prothrombin time, and partial thromboplastin time). If the coagulation profile is abnormal, further evaluation with specific parameters to evaluate for disseminated intravascular coagulation (fibrinogen, fibrin split products, and D-dimer) should be ordered. For patients with respiratory dysfunction, arterial blood gases are appropriate to evaluate for pending respiratory failure, and for patients with severe sepsis, a lactate and a central venous or mixed venous blood gas is also appropriate (see the section Fluid Therapy). Septic patients commonly have multiple abnormalities on laboratory examination.
As previously discussed, the pathogenesis of sepsis can affect every organ. After a thorough history and physical examination, diagnostic imaging should be ordered targeting abnormalities noted on physical examination. Chest imaging is frequently useful, and is necessary in patients with suspected respiratory or pleural infection. A simple flat plate radiograph of the abdomen can help in diagnosing ileus or perforation, although computed tomography has superior diagnostic capability for the myriad of diseases that occur in the abdomen (eg, pancreatitis, colitis, biliary diseases, or abscess). Ultrasonography is increasingly useful in the evaluation of many sources of infection, including the chest, abdomen, genitourinary system, soft tissue, and cardiac structures.
Various biomarkers have been evaluated for diagnosis, risk stratification, and prognosis in sepsis. In the most recent sepsis consensus conference, the diagnostic approach to sepsis remained unchanged largely because no biomarker has sufficient diagnostic accuracy to reliably diagnose or exclude sepsis.66,67 However, a few biomarkers are worth discussing for either conceptual illustration or because of purported clinical value: interleukin-6 (IL-6), C-reactive protein (CRP), soluble triggering receptor expressed on myeloid cells (sTREM)-1, and procalcitonin (PCT).66
Tumor necrosis alpha (TNF-α) induces IL-6, which has a longer half-life than other inflammatory cytokines and thus can be measured reliably in the serum after the host mounts an immune response. IL-6 has been identified as an important mediator in septic shock and has shown a correlation with disease severity.66 A retrospective study of the placebo arm of the Recombinant Human Activated Protein C Worldwide Evaluation in Severe Sepsis (PROWESS) trial found that IL-6 levels correlated with AKI.45 However, IL-6 lacks specificity because it is elevated in various noninfectious inflammatory conditions as in trauma, surgery, and critical illness.68 Previous studies have revealed that the accuracy of IL-6 likely depends on the timing and frequency of measurements, with levels >1000ng/mL being highly predictive of sepsis-related death.66 IL-6 levels are not routinely available from a clinical laboratory.
C-reactive protein is an acute phase protein with both pro- and anti-inflammatory properties that is produced mostly by hepatocytes and alveolar macrophages.66 CRP, through the expression of anti-inflammatory cytokine transforming growth factor β (TGF- β), augments opsonization and phagocytosis of apoptotic cells.69 Clinically, CRP levels are often used to monitor antibiotic treatment response to various chronic infections, such as osteomyelitis. Similar to IL-6, CRP is elevated in various noninfectious states and although inexpensive and widely accessible, it is not sufficiently specific for clinical use in patients with sepsis. In addition, studies have found that CRP levels are elevated in sepsis but they do not correlate well with Sequential Organ Failure Assessment scores (see the section Severity Index Scores).70,71
Soluble triggering receptor expressed on myeloid cells (sTREM-1), part of the immunoglobulin superfamily, is stimulated in response to infection. Previous studies have investigated the use of sTREM-1 as a diagnostic biomarker for febrile neutropenic patients and found sTREM-1 sensitivity and specificity were 88% and 48%, respectively.72 When comparing serum sTREM-1 and cytokine levels between septic and nonseptic patients with ARDS, sTREM-1 could not differentiate between groups, although higher initial levels of sTREM-1 and increasing levels over 5 days predicted higher mortality.73 Other studies in adults and neonates have failed to demonstrate superiority of sTREM-1 over CRP, PCT, or other markers for the diagnosis of sepsis, although they are generally prognostically significant.74,75
Procalcitonin, a propeptide of calcitonin, is involved in the host inflammatory response. In animal models of sepsis, blocking PCT improved organ dysfunction.66 Multiple studies have been done looking at PCT as a specific diagnostic and prognostic biomarker for sepsis. Riedel et al studied the usefulness of PCT in the emergency room as a marker for blood stream infections. Serum samples of PCT were taken the same time blood cultures were obtained in 295 patients. Sensitivity and specificity for the PCT assay were 75% and 79%, respectively. The positive predictive value was 17% and the negative predictive value 98% compared with blood cultures, suggesting that PCT is a potential useful marker to evaluate for sepsis.76 PCT is studied in various other contexts as a marker of severity or a prognosticator for mortality such as postoperative sepsis, burn-related sepsis, and trauma-induced sepsis.77-80 These studies concluded that incorporating PCT into sepsis management for diagnosis and prognosis was beneficial. Karlsson et al found that although median PCT levels were not different between survivors and nonsurvivors, survivors had a greater than 50% decrease in their admission PCT levels compared to nonsurvivors, suggesting that the percent decrease of PCT levels was more important than the absolute level of PCT.81 Comparing PCT to CRP, IL-6, and lactate, PCT is consistent in detecting sepsis with a strong negative predictive value.70,82 The US Food and Drug Administration has approved the use of PCT for risk assessment for day 1 of ICU admission to determine progression of severe sepsis and septic shock, designating less than 0.5ng/mL and greater than 2ng/mL as low and high risk for illness severity respectively.66 PCT has also been studied as a marker in a pilot antibiotic stewardship program. Nobre et al stopped antibiotic therapy when there was greater 90% drop in PCT level after 3 days on antibiotics and found a 4-day reduction in antibiotic use, and a 2-day decrease in ICU length of stay, without an increase in recurrent infections or death.83 This study excluded immunosuppressed patients or patients with prolonged infections like endocarditis or osteomyelitis. PCT is the most promising sepsis biomarker to date; however, the assay availability and consensus on how the PCT absolute values should be interpreted and used for clinical judgment is still undecided.
To date, there is no single biomarker that provides sufficient diagnostic discrimination either to diagnose or to exclude sepsis. It remains to be seen whether any biomarker may improve the diagnostic or prognostic abilities to what is currently used, such as physical and laboratory examinations, and illness scoring systems (see the section Severity index scores). Given the complexity of sepsis and the common approach to integrate multiple pieces of information in decision making for these patients, the next approach may be to analyze a group of markers together in combination.66
Severity Index Scores: There are several prognostic severity illness scoring systems that have been studied and validated to risk stratify critically ill patients on the first ICU day. These include Acute Physiology and Chronic Health Evaluation (APACHE II), Simplified Acute Physiology Score (SAPS II), Sequential Organ Failure Assessment (SOFA), and Mortality Prediction Model (MPM-0). Each of these scoring systems allows clinicians to predict the likelihood of an adverse clinical outcome, such as death. Although they have differing strengths and weaknesses, they universally suffer from the same basic problem: they only accurately predict outcomes for a group of patients and not for an individual patient. However, they do permit institutional benchmarking for quality improvement, and they allow clinical researchers to compare treatment effects across patient populations controlling for illness severity or organ dysfunction. Here we discuss select severity scoring systems as they relate to sepsis (Table 64-2).
Comparison of Severity Index
APACHE IV | SAPS III | SOFA | MPM0-III |
---|---|---|---|
Age | Age | Age | |
ICU admission diagnosis | ICU admission diagnosis | ICU admission diagnosis | |
Chronic disease | Chronic disease | Chronic disease | |
Patient location prior to ICU admission | Patient location prior to ICU admission | ||
Nonelective surgery | |||
Emergency surgery | |||
Length of stay before ICU | |||
Mechanical ventilation | Mechanical ventilation within 1h of admission | ||
CPR 24h before admission | |||
Full code status | |||
Physiologic variables | |||
Temp | Temp | ||
MAP | SBP | MAP | SBP |
HR | HR | HR | |
GCS | GCS | GCS | Coma |
RR | |||
PaO2/FiO2 | FiO2 and PaO2 if ventilated | PaO2/FiO2 | |
Serum bilirubin | Serum bilirubin | Serum bilirubin | |
Serum sodium | Serum sodium | ||
Serum potassium | Serum potassium | ||
Serum creatinine | Serum creatinine or urine output | ||
WBC | WBC | ||
Platelet count | |||
BUN | BUN | ||
Urine output mL/24h | Urine output | ||
arterial pH | |||
Hematocrit | |||
Serum bicarbonate | |||
Glucose | |||
Albumin |
The first part of the APACHE scoring system is the Acute Physiology Score (APS). It calculates the probability of hospital mortality based on the main diagnosis84 and takes into account 33 physiologic measurements within the first 24 hours of patient presentation. The scoring system ranges from 0 to 4 for each of the 33 physiologic measurements. The scoring is based on the worst vital sign, common laboratory, and Glasgow Coma Score derangements in the first 24 hours. It also takes into account the patient’s chronic health, evaluating preexisting chronic medical conditions or surgeries that will predispose the patient to an acute illness. APACHE II was validated in a study of 833 consecutive ICU admissions and produced accurate estimates of death rates and prognostication in various disease states.85 APACHE continues to be updated. APACHE III takes into account the acute diagnosis, the patient’s location prior to ICU admission and lead time, while APACHE IV includes additional chemistries, whether the patient was mechanically ventilated, the ICU admission diagnosis, length of hospital stay before ICU admission, and whether emergent surgery was performed.84,86
SAPS is a severity scoring system for estimating the risk of hospital death using 17 variables: 12 physiologic variables, age, type of admission, and 3 underlying disease states (hematological malignancy, acquired immunodeficiency syndrome, and metastatic cancer). SAPS II provides estimated risk of death without a primary diagnosis. Not requiring a primary diagnosis makes this scoring system advantageous, because often patients in the ICU have multiple or initially unknown diagnoses.87 However, when this scoring system was validated in a multinational large clinical trial, the study excluded burn and cardiac patients.87 It is considered the simplest system for measuring ICU performance and comparing across years.84
The development of the SOFA score was established to categorize the degree of organ dysfunction over time and to evaluate morbidity in septic ICU patients. The SOFA score assigns 1 to 4 points for the level of dysfunction to six organ systems on a daily basis: respiratory, circulatory, renal, hematology, hepatic, and central nervous system.88 A systematic review evaluating SOFA for predicting mortality in the ICU revealed that SOFA scores at admission faired a little worse than APACHE II/III, but were comparable with SAPS II. Serial SOFA scores seem to perform similarly to other organ failure scores. The systematic review concluded that combination of the various models of SOFA with APACHE II/III and SAPS II improved prognostic performance.88
MPM-0 is a model predicting the probability of hospital death taken at 24, 48, and 72 hours. It uses chronic health status, acute diagnosis, physiologic variables, and other parameters including mechanical ventilation.84 MPM-0 was validated on 12,610 critically ill patients across Europe and the United States from 1989 to 1990.89 MPM-0 was then readjusted because observed mortality rate was lower than the predicted aging model. MPM-0 was recalibrated from 124,885 critically ill patients from 2001 to 2004. Fifteen independent variables were used in addition to elective surgical patients and “do–not-resuscitate” orders were also taken into account.89
Septic patients often require intensive care due to the severity of their illness and the monitoring that is required for optimal patient care. The combination of dehydration and vasoplegia may result in profound hypotension with circulatory shock, necessitating some form of hemodynamic monitoring. In particular, because early fluid resuscitation is crucial in the management of sepsis, accurate hemodynamic monitoring is critical to the initial approach to patient management and assessing the response to medical interventions. The most common parameters used in monitoring septic patients are pulse oximetry, central venous pressure (CVP), central venous or mixed venous oxygen saturation (ScvO2, SvO2), cardiac output (CO), systemic vascular resistance (SVR), and extravascular lung water (EVLW). Each of these parameters is complementary and may assist in both the early and later management of sepsis, organ dysfunction, and shock.
Central venous pressure can be measured by transducing the pressure from a thoracic central venous catheter placed in either the internal jugular vein or the subclavian vein with its tip resting in the right atrium. CVP is used in the algorithm to deliver early goal-directed therapy (EGDT) (see the section Fluid Therapy), primarily as a measure of volume status and cardiac preload. Although some studies have suggested that CVP may be used to predict the hemodynamic response to fluid administration (eg, increased cardiac output after fluid administration),90 CVP is notoriously inaccurate for this purpose.90 CVP cannot accurately identify patients who will respond to fluid administration, or those who will not respond to fluid administration with improved hemodynamics. In addition, CVP measures are context sensitive: for example, values <5 mm Hg may indicate hypovolemia in patients with sepsis and may be normal in healthy individuals. In addition, although the goal CVP for sepsis resuscitation is generally 8 to 12 mm Hg, for patients receiving positive pressure ventilation a higher CVP target (12-15 mm Hg) may be appropriate.91 Overall, CVP is not a good predictor of intravascular volume or fluid responsiveness and it cannot be used alone in determining fluid administration in sepsis.
Central venous oxygen saturation (ScvO2) may be determined from a thoracic central venous catheter, either by blood gas analysis or internally using a fiberoptic catheter. For patients with a pulmonary artery catheter in place, the same measures may be taken from the distal catheter tip for measures of mixed venous saturation (SvO2). Because Scv
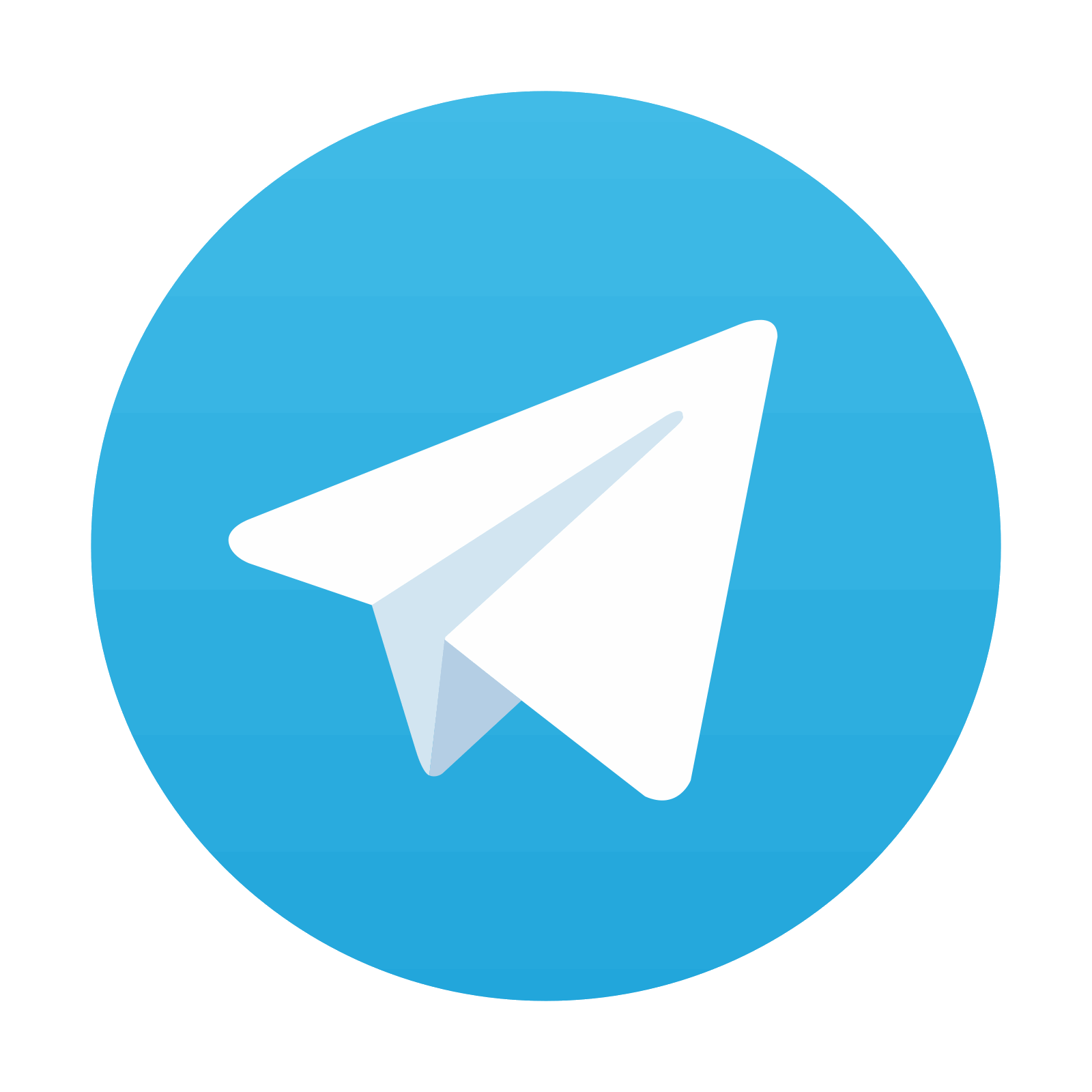
Stay updated, free articles. Join our Telegram channel
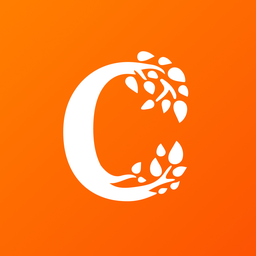
Full access? Get Clinical Tree
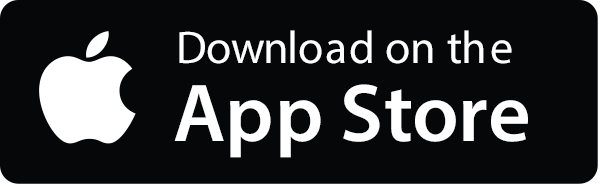
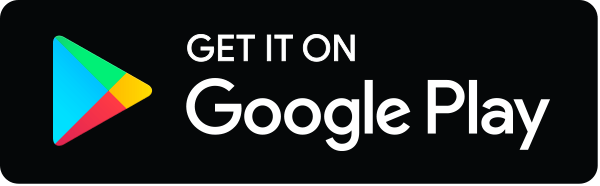
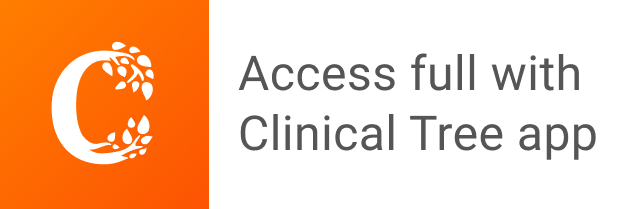