Fig. 3.1
Patient 1 (a) T1-weighted MRI scan illustrating the lesion location (white arrow) in the right ventroposterolateral (VPL) nucleus, 3 months post-stroke. Image location is 5 mm above the line adjoining the anterior and posterior commissures (AC-PC line). R right, L left. (b) Perceptual thresholds (PTs) to vibratory stimuli applied to the pad of the thumb in the absence (open bars) or presence (filled bars) of competing vibratory stimulation applied to the contralateral (CL) hand. Asterisks represent PTs exceeding the 95 % confidence interval (CI) for PTs of the unaffected hand Fig. 3.1 (continued) in the presence of contralateral vibration. (c) Median nerve SEPs from CP3 in the absence (thick line) and presence of vibration of the contralateral hand (thin line) at 2 weeks post-stroke. The arrow indicates stimulus onset. (d) N20-P27 amplitudes with contralateral vibration during recovery expressed as a percentage of the resting amplitude. The grey horizontal bar represents the 95 % CI surrounding the mean task-related N20-P27 amplitude difference in controls (n = 15). Reprinted with permission from Staines WR, Black SE, Graham SJ, McIlroy WE. Somatosensory gating and recovery from stroke involving the thalamus. Stroke, 2002, 33, 2642–2651
This was also evident using functional magnetic resonance imaging (fMRI) conducted at similar time points (Fig. 3.2). At 2 weeks, unilateral stimulation of the stroke-affected hand significantly activated voxels in the area of contralateral SI (top panel). However, during bilateral stimulation there was no significant activation in the ipsilesional SI. As recovery progressed, significant activation within the same spatial regions of the ipsilesional SI was observed during both unilateral and bilateral stimulation. We showed that efficient task-related modulation of sensory inputs is an important factor contributing to recovery from thalamic stroke. Specifically, deficits in the ability to detect contralesional tactile stimuli when presented bilaterally are reflected by deficiencies in sensory gating mechanisms measured at the level of SI. The impact that disrupted modulation has on sensorimotor control and recovery after stroke is not clear, but has the potential to be substantial (Fig. 3.2).
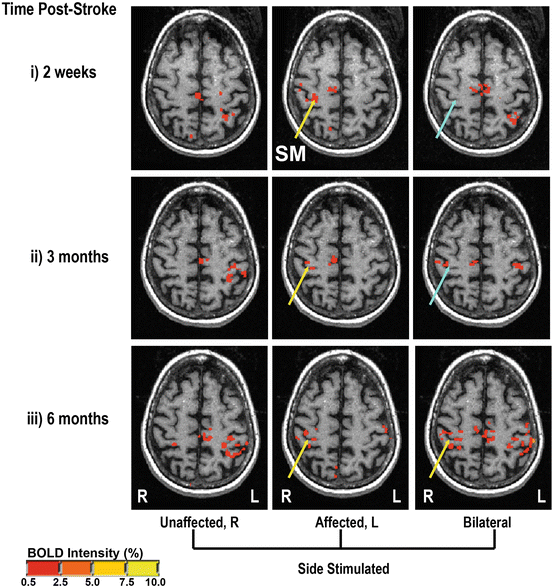
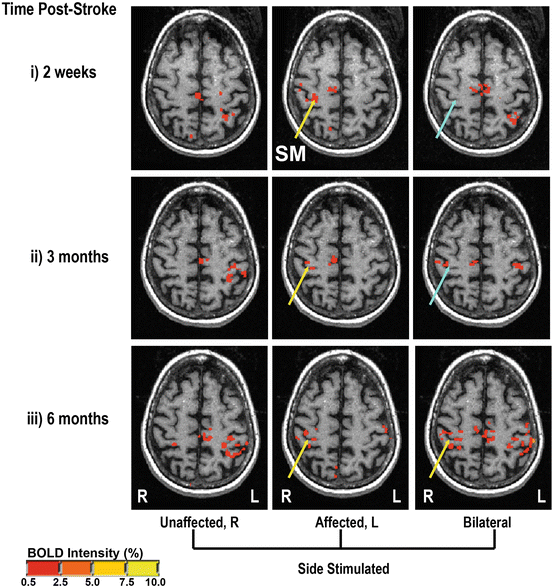
Fig. 3.2
Patient 1 fMRI scans (Talairach space, 47 mm above the AC-PC line) during tactile stimulation of the index finger on the right hand alone (Unaffected, R), the stroke-affected left hand alone (Affected, L) or both hands simultaneously (Bilateral). Each column represents repeats of the same scans at different times post-stroke: (i) 2 weeks, (ii) 3 months, and (iii) 6 months. Yellow arrows indicate the presence and blue arrows the absence of significant BOLD response in the area of SI. Reprinted with permission from Staines WR, Black SE, Graham SJ, McIlroy WE. Somatosensory gating and recovery from stroke involving the thalamus. Stroke, 2002, 33, 2642–2651
Importance of PFC
The PFC influences distributed neural networks and is critically involved in the top-down modulation of sensory signals both at the unimodal sensory processing level, and in higher order association regions [12, 18]. This modulation has been demonstrated across different sensory modalities including visual, auditory, and somatosensory processing [12]. Numerous lines of evidence, including lesion studies in humans [19, 20] and imaging work [21] have established clear links between prefrontal activity, the suppression of distracting sensory information, and subsequent cognitive performance. In patients with frontal lesions for example, the loss of normal PFC function is associated with an impaired ability to filter out irrelevant distractor sensory information, and a loss of excitatory control over sensory pathways carrying task-relevant information even at early modality-specific cortical processing stages [12]. Such clinical findings relate to the extensive anatomical connections between prefrontal regions and distributed neural networks including sensory processing sites [22]. Indeed, PFC acts to suppress irrelevant information early in the processing stream, including at the earliest cortical stages of sensory processing and even at the thalamus prior to cortical entry [23–26]. Due to this capacity to gate out irrelevant sensory information, the PFC has a critical role in providing resistance to distraction and in sparing limited cognitive resources from becoming overwhelmed by a surplus of sensory input.
In animal models, cryogenic blockade of PFC results in larger sensory evoked responses at SI revealing tonic PFC inhibition over early somatosensory processing [27]. Additionally, studies in rats [23] and non-human primates [28] indicate that PFC modulates somatosensory signals via thalamic connections. Furthermore, evidence from humans with selective frontal lesions suggests inhibitory modulation of incoming somatosensory signals through direct fronto-parietal connections [25]. Such regulatory connections make the PFC an ideal candidate for gating irrelevant and boosting relevant information across all sensory modalities, including somatic sensation.
Temporary suppression of cortical target regions can be induced experimentally using repetitive transcranial magnetic stimulation (TMS). For instance, the effect of suppressing prefrontal activity on somatosensory or other sensory cortical processing can be addressed. In one study, TMS and electroencephalography (EEG) were combined to investigate the role of the dorsolateral prefrontal cortex (DLPFC) in regulating attention-based modulation of somatosensory signal processing [13]. Following the application of continuous theta burst stimulation over the right DLPFC, it was noted that attention-based modulation for an early modality-specific component of the EEG (P100) was significantly attenuated. This suggested that the DLPFC contributes to filtering irrelevant somatosensory information at early cortical processing stages, with the P100 most likely being generated in the secondary somatosensory cortex [29]. Moreover, the source of this attenuation resulted specifically from the loss of suppression of non-attended stimuli suggesting that the DLPFC contributed to sensory gating during this task to suppress irrelevant distractor information.
The impact of prefrontal regions on suppressing irrelevant distractor inputs has been corroborated by another TMS study. Hannula and colleagues noted that single-pulse TMS applied to the middle frontal gyrus at a specific time-point in the retention phase of a tactile working memory task resulted in significantly improved performance, particularly in the midst of tactile distractor stimuli [30]. Notably, these authors observed that this single pulse was associated with a reduction in SEP amplitude from the tactile distractor stimuli, which they proposed as support for the notion that the major mechanism for performance improvement in this paradigm was the suppression of irrelevant data. Interestingly, this group investigated the specificity of this link between the middle frontal gyrus to the SI by also having the tactile working memory task with visual interference. The TMS stimulation did not improve performance in this case suggesting that the inhibitory interference control was specific to somatosensory inputs [31]. Overall, damage to the PFC can impact sensory-related activity at modality-specific cortical areas and demonstrate a behavioral consequence.
What Factors Affect Sensorimotor Recovery?
Following a cerebral insult, such as a stroke, the CNS undergoes reorganization or plasticity during the process of functional recovery. However, the degree of recovery is variable [32] and the processes subserving this recovery are incompletely understood. Neurophysiological changes associated with recovery often begin very early after the onset of stroke (within 1–2 weeks) and may plateau between 2 and 3 months later, depending on the specific neurologic deficit. Historically, the recovery over the first 3 months is believed to be the most rapid. However, functional recovery has been shown to progress even years after the stroke event, albeit at a much slower rate. Several factors influence the rate and extent of recovery, most notably the initial severity of the stroke, which is typically quantified clinically or functionally, and the size and location of the lesion. Recovery can also be influenced by individual characteristics, particularly age, comorbidities, and activity patterns of the individual, specifically their participation in formal rehabilitation training. Questions remain about the mechanisms by which such improvement is mediated, however, rehabilitation training may be critical in shaping reorganization within the CNS.
In both the healthy and injured CNS, the development and training of motor skills leads to rapid cortical adaptation characterized by shifts in central representation to meet new task demands [5, 33–38]. During sensorimotor recovery from stroke, these normal training-related cortical adaptations can interact with and influence cortical reorganization associated with spontaneous recovery. Electrophysiological and functional imaging techniques, such as TMS, event-related cortical potentials, and fMRI allow for the sensitive quantification of CNS state and provide important experimental opportunities to reveal treatment-related short-term changes as a foundation for long-term stroke recovery.
For many deficits, the key to minimizing disability following stroke is rehabilitation to retrain patients to improve capacity. The range of these approaches is largely distinguished on the basis of the specific deficit and severity, which can be quite heterogeneous. While research continues to establish specific characteristics of the most effective rehabilitation techniques, the common thread among the more successful approaches is a requirement for patients to practice “use” of the affected body part [39, 40]. In addition, the use needs to feature increased challenges that are task-related (as opposed to simple movement repetition) [41]. In the last decade, studies have shown that such task-related motor retraining may lead to improved recovery by facilitating brain repair. Such brain repair may also be augmented by specific neuropharmacological agents, the use of factors to stimulate cell growth and/or cell replacement therapies whereby endogenous stem cells are stimulated to migrate to the injury site [37, 42, 43]. These approaches are still largely at the experimental stage in animal models. One of the challenges in translating these findings to humans is to understand why and with whom to use a specific therapeutic approach in order to maximize recovery.
Neurophysiological effects occur both in the injured and uninjured hemispheres following brain injury due to stroke, illustrating the potential importance of the interaction between the two for motor recovery. Of a variety of treatment strategies, bimanual movement training (BMT) of the upper limbs has specifically shown potential to promote behavioral and functional improvement in both the subacute and chronic phases of stroke recovery [44–49]. Although there is some evidence that bimanual movement, involving both the damaged and intact hemispheres, may enhance motor-related brain activity in the stroke-hemisphere in some patients [50, 51], the neurophysiological mechanisms that contribute to the benefits of BMT are not yet clear. More specifically, few studies have reported on the cortical changes that underlie observed behavioral improvements following BMT in stroke patients [52]. The findings, however, have been consistent with reports in healthy individuals, and have shown a facilitation of primary motor cortical (M1) excitability during movements that involve activation of the contralateral homologous muscle representations [52–56].
Changes in the balance between local intracortical inhibition and excitation occur in both the affected and unaffected hemispheres following stroke [57–59]. In the subacute stage, complex changes in the function of local GABAergic interneurons (i.e., inhibitory interneurons) occur in both hemispheres. M1 lesions in animal models induce a decrease in GABAA receptor expression both in the areas immediately adjacent to the lesion and in structurally intact but connected regions, including contralesional M1 [59]. Using TMS in human stroke patients, there is evidence for both increases and decreases of cortical inhibition in the same patients [57], suggesting the presence of distinct, independent inhibitory interneuronal circuits. Importantly, the amount of cortical disinhibition appears to be correlated with the degree of hemiparesis, being more pronounced with less severe hemiparesis.
Cortical activity is enhanced in both hemispheres in damaged and healthy M1 when homologous muscles are activated together [50, 51]. It is thought that transcallosal neural activity of homologous representations in M1 act to excite and/or release inhibition from the contralateral hemisphere [60], which may contribute to M1 plasticity. Intracortical inhibition is decreased in M1 when the two upper limbs co-activate the homologous muscles simultaneously, but inhibition remains when they are not activated simultaneously [60]. Premotor cortical areas, such as the dorsal premotor cortex (PMd), have extensive reciprocal neuronal projections with M1 [61, 62] and have the potential to influence motor cortical excitability. In-phase bimanual training, in which homologous muscles are activated simultaneously in the two limbs, increases fMRI activity in the lateral premotor cortical areas, including PMd [63]. Also, short-term in-phase bimanual training, particularly involving visual cues, has been associated with increases in lateral premotor cortical activity [33, 34]. Contralesional PMd activity has been shown to influence ipsilesional sensorimotor regions, which could provide a mechanism to contribute to recovery of motor function post-stroke [64, 65]. Therefore, the liberation of inhibition due to homologous M1 extensor carpi radialis representations activated together, along with this activity engaging areas in PMd that could further facilitate muscle representations in M1, may have implications for enhancing motor function following stroke.
There now remains no debate about the importance of aerobic training after stroke. It has been shown to positively influence both stroke-related outcomes and risk factors for recurrent stroke. There exists convincing evidence of the benefits of aerobic training as reflected by improved cardiovascular function, reduced neurological impairment, enhanced sensorimotor function and endurance, and as a stimulus to promote neuroplasticity [66–72]. The evidence is so compelling that physical exercise, specifically aerobic training, is an important element of stroke management and promotes post-stroke recovery; it is part of the best-practice recommendations [73].
It is important to recognize that focused, patient-specific therapy is an important element in minimizing post-stroke disability. Determinants of the relative success of such therapy, beyond the specific techniques applied, include the intensity of therapy, patient motivation/participation in the program, and the timing of the therapy. It is becoming clear that more time directed at retraining following stroke, including patient-appropriate practice beyond the clinical setting, can enhance recovery. Within the first month after stroke, the standard discipline-specific therapy occurs on average 3 times a week for 30–60 min per session. While intensifying the routine at this stage of recovery may improve outcomes, there is growing evidence that a lack of long-term opportunities for rehabilitation services will, in many patients, result in progressive worsening of outcomes. For example, persisting disability in some patients leads to further disuse and musculoskeletal disorders that can further limit the ability of the individual to participate in activities of daily life, decrease their aerobic capacity, and compromise their physical activity. Objectively tracking functional status over time may help to document changes and screen for and establish the need for continuing therapy to offset the consequences of persisting disability. It should also be reinforced that prescribed physical activity training, both aerobic and strength training, is essential to the rehabilitation process. The difficulty in training for stroke patients can be the suitability and/or safety of the exercise activity selected. While a walking program may seem right for some people, they must be able to walk safely and rapidly enough to achieve target heart rates. For those unable to walk quickly enough or unable to walk at all, a recumbent cycle ergometer or stepper is an effective modality of training; it has been successfully used in the subacute and chronic stages after stroke and can help counter the deconditioning that occurs after a stroke [72, 74–76]. The concern for compliance can be evaluated and even motivated by monitoring activity logs using wearable activity monitors [77–79]. Cardiac rehabilitation, an established model of care for individuals with cardiac disease, may also be adapted to serve as an effective approach for those who have had a stroke [80, 81]. The next step in treatment is to couple the benefits of aerobic training with task-specific retraining in order to maximize sensorimotor recovery after stroke.
Summary
Here we have briefly discussed two classes of functional impairments that can occur following stroke: hemiparesis in the upper limbs and changes in the modulation of somatosensory information—more specifically, the inability to efficiently filter non-task relevant information. We have discussed what effect this can have on sensorimotor function, as well as some advances in knowledge on recovery and treatment following stroke. Although the etiology of these may differ, the neurophysiological substrates for recovery are similar. For example, Kautz and colleagues have shown limited benefits to the control of walking associated with an aerobic pedaling program, despite improvements in walking velocity [82]. They concluded that to achieve gains in the control of walking, “task-specific intervention may be required to improve coordination, consistent with principles of use-dependent plasticity.” In this way the substrates for recovery between the differing levels of impairment converge. The underpinning of functional recovery may be training-related adaptation in the CNS that is maximized with use of the paretic limb in challenging, skilled tasks [83]. Optimally, this could be combined with physical activity or aerobic training to achieve the cardiovascular benefits associated with decreased risk factors for recurrent stroke.
References
1.
Heart and Stroke Foundation of Ontario. Facts you should know about heart disease and stroke. Toronto, ON: Ontario Heart and Stroke Foundation; 1999.
2.
Basmajian JV, Gowland C, Brandstater ME, Swanson L, Trotter J. EMG feedback treatment of upper limb in hemiplegic stroke patients: a pilot study. Arch Phys Med Rehabil. 1982;63(12):613–6.PubMed
3.