Proapoptotic
• Isoflurane, sevoflurane, desflurane, N2O
• Ketamine, propofol, thiopental, midazolam, diazepam, MgSO4, dexamethasone, CO2
Antiapoptotic
• Lithium, melatonin, clonidine
• Preconditioning (with ketamine), NAP, TRP601
• Vitamin D3
• Environmental enrichment
Non-apoptotic
• Dexmedetomidine, opioids, ± xenon, local anesthetics
Unknown
• Muscle relaxants
Table 4.2
Functional deficits associated with specific anesthetic agents identified in animal studies
Anesthetics | Learning | Spatial memory | Social memory | Spontaneous motor activity | Attention | Behavior |
---|---|---|---|---|---|---|
Isoflurane | x | x | x | x | x | |
Sevoflurane | x | x | ||||
Nitrous oxide | x | x | x | x | x | |
Propofol | x | x | x | |||
Ketamine | x | x | x | x | x | |
Midazolam (as part of a cocktailn) | x | x | x | x | x | |
Barbiturates | x | x |
Numerous investigators have questioned the appropriateness of the model used to address this question. In most experiments, fewer than two-thirds of the animals survive until the conclusion of the study, begging the question how could such a model hold relevance to human anesthesia at all. Second, most experiments did not monitor cardiorespiratory variables throughout to ensure that they did not the harm the animals. Third, the MAC of the inhalational anesthetics is not constant over time in these animals. Finally, the care provided to these newborns after the anesthetics may not have been optimal [39].
Despite the obvious concern regarding the potential harm apoptosis may cause in humans if similarly affected, great strides have also been made in identifying strategies to block and prevent neuroapoptosis in newborn rodents. Several drugs have been identified as having antiapoptotic properties (Table 4.1). Preconditioning (with ketamine) and vitamin D3 have both been effective individually in attenuating apoptosis [40]. Additional neuroprotective strategies using NAP and TRP601, the former a derivative of the activity-dependent neuroprotective protein [41] and the latter an irreversible caspase inhibitor [42], both substantively decrease the severity of the apoptosis in newborn animal models [41, 42]. In addition, environmental enrichment, which attenuated brain injury during recovery in adult rodents, effected similar salutary effects in newborn rodents exposed to anesthesia [39, 43, 44].
The mechanisms of the neurotoxicity induced by anesthetic agents in the immature animal brain remain incompletely understood. We know that most anesthetics act by activating GABAA receptors and/or inhibiting NMDA receptors (exceptions include clonidine/dexmedetomidine and opioids). These neurotransmitters play a vital role in the normal development and maturation of the brain, particularly but not exclusively, during the vulnerable period of rapid synaptogenesis. During rapid brain growth, neurons that are superfluous and no longer required become quiescent, which triggers caspase 3 activation. This activates apoptosis and the superfluous cells involute and disappear. This remodeling process enables the brain to remove superfluous neurons and expand other parts of the brain. Anesthetics that modify GABA- and NMDA-mediated neurotransmission might disrupt this process by depressing the neurons and “putting the neurons to sleep,” triggering apoptosis even though the neurons were not scripted for removal. This “abnormal neuronal inhibition” could trigger apoptotic cell death in vulnerable neurons, or alter the normal synaptogenesis [27], causing a decrease in neuronal density and synaptic remodeling. The balance between NMDA- and GABA-mediated neuronal activity could also affect dendritic spine development and neuronal connectivity [45]. However, these theories should be regarded with caution, since the activity of GABAA receptor, for example, one of the main inhibitory systems in the mature brain, displays excitatory properties in the immature cortical neuron [46].
What is the relevance of neuroapoptosis in terms of future neurological development? Apoptosis is a normal process during brain development in mammalian species. In humans, 50–70 % of the neuronal cells will have undergone “physiological” apoptosis by the end of the cerebral maturation [47, 48]. If this process is arrested, cerebral malformations and premature death occur in a mouse model [49]. It remains to be determined if the apoptosis observed after anesthesia targets neurons that would have spontaneously died in the natural course of development or if it destroys neurons that were destined to be part of the final cerebral network. However, we do not even know if such a fixed predestination of neurons exists or if the central nervous system is able to compensate for one neuronal death by “sparing” another cell.
Many have criticized the notion of extrapolating neuronal and neurocognitive sequelae in animal studies to human neonates. These issues have been addressed in several reviews or editorials [50–54].
The corresponding period of neuronal susceptibility in humans, if one indeed exists, is unclear. It was believed, on a weight basis approach, that the brain growth spurt period in humans started in the third trimester of gestation and lasted until the third year of life [55–57]. A cell count approach points to the interval between 28 and 33 weeks of gestation as the period of maximal neurogenesis [46]. Recently, modern “neuroinformatic” methods set the peak window of vulnerability to 17–22 weeks of gestation, until the early postnatal period [58]. In this area, we still lack strong evidence defining a potential “danger” period of susceptibility to anesthetic neurotoxicity in humans.
Other questions relate to the dose and duration of anesthetic drugs used in the animal studies. Animals require comparatively greater doses of intravenous drugs than humans, on a weight basis, or with an allometric approach, to achieve the same pharmacodynamic goals [59, 60]. As a result, most of the animal studies are performed with doses that exceed the therapeutic range in humans, but that are relevant for the considered animal models [24, 61]. Anesthetic side effects, for example, at therapeutic concentrations, may be more marked in rodents than in humans, including hemodynamic and metabolic disturbances, and may confound the neurological sequelae [52, 59]. The MAC of inhalational anesthetics is believed to remain fairly constant across species. In a comparative study of isoflurane, sevoflurane, and desflurane, apoptosis was equally severe after all three anesthetics after 6 h [62]. In contrast, neuroapoptosis in newborn rats that were exposed to 1 h of isoflurane was similar to controls and significantly less than that in rats exposed to 6 h of isoflurane, although one study demonstrated neuroapoptosis after 1 h of 2 % isoflurane [25, 26]. However, recent evidence suggests that the MAC of sevoflurane in newborn rats decreases with tail-clamping over time, raising questions regarding the equivalent MAC values in neonatal animals [39]. Many studies in newborn animals have been performed with strict control of hemodynamic, respiratory, and metabolic variables and, despite the controls, still yielded substantive neuroapoptosis. Moreover, histopathologic findings describe apoptotic and not ischemic or excitotoxic mechanisms involved in neuronal cell death observed after exposure to anesthetic drugs.
Finally, all animal studies of neuroapoptosis after anesthetic drugs have been undertaken without surgical or painful stimulation, since pain and/or inflammation by themselves induce neuroapoptosis. In a surgical setting, anesthetics attenuate the potential damage of nociceptive stimulation. Two studies addressed the effects of anesthetics on neuronal function/integrity during an inflammatory insult. These studies yielded conflicting results leaving this concern unresolved [63–65].
A crucial question that is currently being investigated is the relevance of all these in vitro and animal findings in the human neonate. Does anesthesia in the young infant transiently or permanently alter his neurologic development, cognitive performance, or behavioral characteristics? Several case-controlled or cohort studies have sought evidence to answer this question. Although several studies have pointed to an association between children who received multiple general anesthetics before 3 years of age and learning disabilities, these studies have been difficult to interpret due to the presence of multiple confounding variables [66–72]. One notable study of identical twins indicated that intellectual indices did not differ between twins who were discordant for receiving anesthesia at a young age. In sum, the current clinical evidence does not establish an association between general anesthesia in young children and cognitive dysfunction. Prospective studies are required to clarify this issue [73, 74]. Nonetheless, some have taken the enormous leap of faith by assuming that the current clinical evidence heralds similar outcomes in humans and have recommended delaying elective surgery in neonates until they are older [75, 76]. However, few surgeries in neonates are totally elective. Locoregional may be preferred to general anesthesia and the use of drugs that do not trigger apoptosis, preferred to those that do, where possible. In addition, an enriched postoperative environment and strategic interventions (see above) may attenuate the risk of neurocognitive pathology. A measured response is far more appropriate than delaying all surgeries in neonates, in which parents and guardians review the risk-benefit ratio of proceeding versus delaying surgery and anesthesia, with the responsible physicians and surgeons.
A second issue that has raised concerns regarding the safe use of inhalational anesthetics in children has been epileptiform EEG activity. The immature neonatal cortex is relatively more vulnerable and sensitive to anesthetics than the mature cerebral cortex. The baseline EEG pattern of a neonate is characterized by slow oscillations, reflecting immature brain functioning. The complexity of the EEG increases with age, from neonates to adults. Evidence suggests that infants require less sevoflurane to reach a given target of cortical inhibition (BIS 50) than do older children [77]. Similarly, Rigouzzo et al. reported that subtotal cortical inhibition (defined by the occurrence of burst suppression patterns on the EEG) was observed with lower concentrations in infants than in older children. Taken together these studies suggest that infants are more sensitive to cortical inhibition produced by sevoflurane.
In terms of surgical incision, greater end-tidal concentrations of sevoflurane are required to inhibit movement as evidenced by the greater MAC values for 0–6 months compared with older children. These data suggest that in the neonate, subcortical structures, at least at the level of the spinal cord, are less sensitive to sevoflurane-induced inhibition.
The balance between cortical and subcortical processes in the neonate appears to differ from that in older children or adults. If we seek to inhibit the subcortical structures to prevent movement and negative subconscious memory formation with potential behavioral consequences, we require larger end-tidal concentrations of sevoflurane in neonates. However, if the cortex is more sensitive to sevoflurane, then these larger concentrations may introduce a risk of neuronal toxicity. So the questions are what is our goal and what level of theoretical risk can we accept?
Fortunately, opioids provide subcortical inhibition and decreased sympathetic activity, allowing reduced doses of hypnotics while reducing concerns regarding toxicity.
If all of the preceding were true, then the challenge for the anesthesiologist is to find a compromise between subcortical inhibition and potential toxicity of general anesthetics in neonates. Opioids may provide the solution with a balanced anesthetic, although judicious choice of the correct opioid and the correct dose is required to preclude delayed recovery and delayed tracheal extubation.
Optimal Risk-Benefit Ratio
To optimize the risk-benefit ratio of anesthesia in the neonate (if neurotoxicity proves to hold true in humans), the clinical approach to provide anesthesia for surgery and procedures will be to use anesthetics that are not neurotoxic, to administer adjuvant agents that prevent apoptosis (see above), and/or use regional anesthesia.
Techniques of Anesthesia
Spinal Anesthesia
Spinal anesthesia for the neonate was initially developed in the eighties for its potential benefit to preclude postoperative apnea in the premature and ex-premature infant [78, 79]. In 1998, a landmark study demonstrated a significantly small incidence of postoperative apnea in premature infants who underwent inguinal hernia repair under spinal anesthesia compared with those under general anesthesia with thiopental and halothane [80]. For the premature and ex-premature infant, the risk of apnea decreases steadily between birth and 60 weeks post-conceptional age (PCA), when it is <1 %. At 45 weeks PCA, this risk is approximately of 5 % [81, 82]. Anemia (hematocrit <30 %) [83, 84], hypothermia [85], and other comorbidities such as impaired neurologic or respiratory function increase the risk of postoperative apnea [80–82, 86–89]. It has become an increasingly common practice to consider spinal anesthesia as the preferred technique for brief surgical procedures in the inguinal and lower abdominal areas in ex-premature neonates. This practice has remained unchanged over the past two decades despite the introduction of newer, less soluble inhalational anesthetics.
One study indicated that sevoflurane might induce more cardiorespiratory complications in ex-premature neonates with a preexisting impaired respiratory function than spinal anesthesia, although spinal anesthesia was associated with a substantial failure rate [90]. In a Cochrane review on “regional (spinal, epidural, caudal) versus general anesthesia in preterm infants undergoing inguinal herniorrhaphy in early infancy” [91], the authors concluded that there is “no reliable evidence concerning the effect of spinal as compared to general anesthesia on the incidence of post-operative apnea, bradycardia, or oxygen desaturation in ex-preterm infants undergoing herniorrhaphy” based on 3 published studies that included 108 infants. However, the results were confounded by the concomitant administration of sedatives, mostly ketamine or benzodiazepine, to several infants in the spinal anesthesia arms of the studies. The authors hypothesized that if those who had received sedatives were excluded, spinal anesthesia would have likely been beneficial in terms of postoperative respiratory events.
Because of the significant risk of postoperative apnea, ex-premature infants aged less than 60 weeks PCA require 12 h of apnea-free respiratory monitoring after surgery, irrespective of the anesthetic provided. It has not yet been clearly established whether spinal anesthesia or anesthesia with sevoflurane alone or with local block can abbreviate the period of postoperative monitoring. Some have proposed that certain infants greater than 46 weeks PCA without anemia or comorbidities may be discharged earlier, if they prove to be at a reduced risk for postoperative apnea [92]. This recommendation requires prospective evaluation.
In addition to the possible benefit of avoiding general anesthesia in the neonate, spinal anesthesia has several additional advantages in the preoperative period. It allows a comfortable and safe anesthetic for the infant, who continues to breathe spontaneously; and as opposed to older children, spinal anesthesia does not induce clinically significant autonomic disturbances in the neonate. In particular, arterial blood pressure remains stable [93, 94]. Therefore, cardiorespiratory homeostasis in the neonate is usually maintained during spinal anesthesia. In addition, infants who have received spinal anesthesia are often very calm and peaceful during surgery. Some actually fall asleep during the surgery. Interestingly, the BIS has been recorded in unpremedicated ex-preterm infants undergoing surgery with spinal anesthesia. The BIS decreased during the first 25 min after the spinal, to reach a nadir of 60, that remained unchanged for the next 20 min, but finally returned to 100 during the final 20 min [95]. To explain this response, the authors posited a decrease in the ascending sensory input to the brain, permitting sleep.
Complications with spinal anesthesia are rare, even in large series [89, 96–98]. Recent evidence suggests that complications after neuraxial analgesia in neonates may be as small as 0.29 % [99]. The theoretical risk of septic or aseptic meningitis is very small and has only been reported in two children. In both instances, the role of dural puncture remained uncertain [100, 101]. A high blockade incurring the need for ventilatory assistance (0.67 % in one series) or intubation (0.33 %) [98] is unlikely, if the correct dose is administered, and the infant remains strictly horizontal. Neuraxial hematoma has not been reported in a child, although it has been described in an infant undergoing diagnostic lumbar puncture, who had undiagnosed hemophilia A [102]. The usefulness of testing for coagulation indices in children undergoing lumbar punctures under spinal anesthesia remains contentious. The results are often abnormal, particularly in the premature (<45 weeks PCA), without a clinically significant hemorrhagic tendency [103].
Based on clinical observations of transient or persistent neurological symptoms after spinal anesthesia in adults [104], a direct cytotoxicity of local anesthetics has been suspected and investigated. This neurotoxicity was first characterized in animal studies [105] and then in in vitro human neuronal cells models [106]. The mechanisms are still unclear and probably multiple. However, all local anesthetics, and particularly bupivacaine and lidocaine, have triggered dose-dependent neuronal cell dysfunction, death, or apoptosis, at in vitro concentrations similar to or less than clinical concentrations observed in the CSF after standard spinal anesthesia. Recent evidence suggested that spinal anesthesia does not trigger apoptosis in newborn rodents that received spinal anesthesia on postnatal day 7, 14, and 21 [25]. However, the period of rapid synaptogenesis may peak earlier in the dorsal horn of the spinal cord, e.g., on day 3, not day 7, as in the cortex and basal ganglia [99]. Accordingly, it remains possible that despite the current evidence to the contrary, the spinal cord might be vulnerable to apoptosis in the neonatal period.
The main concern regarding spinal anesthesia in the neonate is its failure rate. The reported failure rate of spinal anesthesia is between 3 % and 20 %, depending on the experience of the anesthesiologist with spinal blocks [89, 90, 98, 107–109].
Regarding the anesthetic potency of spinal anesthesia, in addition to interindividual variability, the duration of analgesia and motor block varies according to the type and dosage of the local anesthetic chosen, but usually lasts a minimum of 60 min [89], which sets the limit of the duration of any surgical procedure performed under spinal anesthesia.
In conclusion, spinal anesthesia displays numerous advantages over general anesthesia and is associated with few complications. Although particularly useful in the ex-preterm infant of less than 60 weeks’ PCA, it may be considered in the neonate undergoing any sub-mesocolic surgery of less than 60 min in duration. Recent concerns about potential local anesthetic neurotoxicity have been articulated in the literature. However, the evidence arises primarily from animal and experimental studies, and their clinical relevance to humans has yet to be established. When the location and duration of surgery make it appropriate, spinal anesthesia may be the optimal option for the neonate.
General Anesthesia
According to the clinical context, and in particular, the probability of full stomach, a rapid sequence induction (RSI) may be preferred.
RSI for General Anesthesia
An RSI is currently performed for every child who has a full stomach, in order to minimize the risk of regurgitation of gastric contents and pulmonary aspiration. To minimize this risk, a proper delay should be observed before induction of anesthesia in the case of non-urgent procedures. The lack of a sufficient fasting period is one of the most common circumstances leading to an RSI.
Current fasting rules were developed based on the best estimate times for gastric emptying of the food product in question. These rules are age independent, although some studies (gastric emptying of infant formula) have only been undertaken in select age groups. Clear fluids may be ingested up to 2 h before anesthesia/surgery in neonates and all older children [110–113]. A Cochrane review on pediatric fasting concluded that ad libitum clear fluid intake until 2 h before surgery improved the child’s well-being and did not increase the risk of regurgitation [114]. Breast milk may be ingested up to 4 h before anesthesia, and artificial milk (e.g., cow’s milk) may be ingested up to 6 h before anesthesia [112, 113, 115]. If it is necessary to start surgery before the stomach is considered empty, an RSI should be performed.
Alternately, some conditions associated with functional or mechanical ileus in the neonatal period require an RSI independent of the fasting interval. The most frequent pathologies are those of the digestive tract: atresia, obstruction, volvulus or perforation, necrotizing enterocolitis, omphalocele, gastroschisis, and congenital diaphragmatic hernia. Pyloric stenosis, which occurs at the end of the first month of postnatal life, is classically considered a condition with “full stomach,” although several institutions routinely perform inhalational inductions of anesthesia in these infants without substantial consequences. In the first months of life, children with hiatal hernia, untreated or persistent esophageal reflux, may also be at risk for regurgitation and considered candidates for an RSI.
Awake Versus Anesthetized Tracheal Intubation
After more than 20 years of debate, routine awake intubation in the neonate has been supplanted by premedication and anesthesia [116]. Today, there remain few reasons to perform tracheal intubation without any premedication in elective intubations [117], although many continue to recommend awake intubations during resuscitation, deteriorating critically ill neonates (and those in respiratory failure) and in those with difficult airways [118]. Recent surveys in France and in the UK [119] revealed that 80 and 90 %, respectively, of elective intubations in neonates were performed with general anesthesia. Several publications reviewed the advantages and disadvantages of premedication (e.g., sedation or general anesthesia) before intubation [120, 121]. Their conclusions consistently suggest that awake intubation in the neonate should be avoided. The physiological consequences [122] of such a procedure include:
1.
Intense pain. The short- and long-term negative consequences of pain in the neonatal period are now well recognized and documented.
2.
An increase in arterial blood pressure.
3.
4.
A decrease in heart rate due to the vagal reaction to intense pain. Tachycardia has also been observed.
5.
A decrease in blood oxygen saturation.
In the past, some clinicians feared the hemodynamic consequences of anesthetic drugs in the neonate. Bradycardia and hypotension were observed during halothane anesthesia in the neonate, although current drugs used in appropriate doses provide hemodynamic stability. In a study in which tracheal intubation was performed in 33 neonates either awake or during sevoflurane anesthesia, adverse events occurred less frequently in the anesthetized group. In particular, they noted the incidence of bradycardia decreased from 44.4 to 8.3 % in the anesthetized group, although the duration of laryngoscopy and intubation was 16 s with sevoflurane and 61 s awake [125]. Inability to perform awake intubations in ~10 s points to a serious failure in mastering the skills of awake tracheal intubation in neonates and raises serious concerns about the veracity of any of these studies [126].
Some also feared the loss of spontaneous ventilation would expose the neonates to the risk of severe hemoglobin desaturations. If the anesthesiologist is skilled to manage the airway in the neonate, mask ventilation will maintain normal oxygenation and ventilation. In fact, fewer desaturations occur when the child is anesthetized before tracheal intubation rather than awake during the procedure [125]. This may be attributed to effective preoxygenation with 100 % O2 in the absence of a child who is struggling, gasping, and crying, particularly during prolonged attempted laryngoscopies.
Premedication provides better conditions for intubation, particularly in the hands of less experienced laryngoscopists. With premedication, the neonate does not move or resist, laryngoscopy is easier to perform [125], and the vocal cords are abducted. In addition, premedication decreases the time to intubation, the number of attempts before a successful intubation, and intubation-related airway injuries [127–129].
Preoxygenation
Preoxygenation is an essential component of an RSI. The aim of preoxygenation is to provide maximal oxygen reserves to allow the greatest duration of apnea without desaturation, in order to avoid hypoxemia before the trachea is intubated. This topic has been poorly assessed in children, especially neonates. However, some of the few pediatric studies include a subgroup of infants less than 1 year of age. We can only extrapolate their results to the first month of life to appreciate the implications.
The younger the age of the child, the less the time interval between removing the facemask and the onset of hemoglobin desaturation. In healthy infants whose lungs were ventilated manually, saturation decreased from 100 to 95 % in approximately 90 s after the onset of apnea and then from 95 to 90 % rapidly (<10 s) [130, 131]. In older children (2–7 years old), 2 min of preoxygenation almost doubled the duration of apnea without incurring hypoxia [132] and that extending the duration of preoxygenation beyond 2 min did not improve the results. When this schema was tested in infants, 1 year and 3 months of age, 2 min of preoxygenation resulted in desaturation to <95 % in 110 s. Desaturation <90 % occurred only 8 s later [133].
Using an end-tidal oxygen fraction of 0.9 as the target end-point for preoxygenation, infants 0–6 months of age required 36 ± 11.4 s or ~60 s [134] for preoxygenation. These results suggested that a brief duration of preoxygenation may be effective in infants, provided the facemask is held tightly against the face during the preoxygenation period.
Cricoid Pressure
Cricoid pressure has been traditionally applied to all patients undergoing RSI to prevent the regurgitation and aspiration of gastric fluids. However, several incidents have been reported [135] in which cricoid pressure caused a clinical problem: excessive pressure [136], incorrect location, and inappropriate timing of the procedure. To be effective, but not traumatic, cricoid pressure must be applied precisely. The usual recommendation is to apply a force of 30–44 N to the cricoid ring with loss of consciousness [137], irrespective of the age of the child. However, a recent bronchoscopic study determined that only 5 N force is required to compress the lumen of the cricoid ring by 50 % in infants [138].
Several others have questioned the effectiveness of cricoid pressure [139]. In adults, an MRI investigation determined that the esophageal lumen was not obliterated by cricoid pressure, but rather it moved laterally from the spinal axis in more than 90 % of patients [140]. In contrast, another MRI study indicated that cricoid pressure obliterated the hypopharyngeal lumen, irrespective of the position and diameter of the esophagus [141]. There appears to be no technique to ensure that in any patient, cricoid pressure reliably obliterates the esophageal lumen.
Even if cricoid pressure were correctly applied, there is no evidence that RSI prevents gastric fluid aspiration. Aspiration is a very rare event with approximately 24 instances of aspiration in 63,180 pediatric general anesthetics, all of whom had cricoid pressure applied during the induction [142]. Moreover, cricoid pressure can impair view of the glottis during laryngoscopy [143–145]. This is particularly important in infants and neonates in whom the adult hand applying the cricoid pressure can limit the mouth opening. In addition, a relatively small force applied to the neck might cause subglottic obstruction in young children [138]. If tracheal intubation is impeded for any of the above reasons, cricoid pressure must be released in order to permit rapid tracheal intubation, which remains the top priority of an RSI. To conclude, cricoid pressure is no longer considered a mandatory ingredient in an RSI, provided the reasons are clearly articulated [139, 146].
Gastric Emptying
Passing an oro- or nasogastric tube before an RSI is neither required nor effective in completely emptying the stomach. However, a gastric tube may be useful in the presence of preoperative ileus or digestive tract obstruction. Since neonates are exclusively fed liquids, a gastric tube may significantly reduce the volume of the gastric fluid contents, although pulmonary aspiration may still occur. If a gastric tube is in place, it is not necessary to remove it before induction [147], and it does not impair the effectiveness of cricoid pressure [143]. The anesthesiologist may however prefer if the gastric tube is removed to ensure clear visualization of the larynx.
Mask Ventilation
Although mask ventilation with oxygen is not usually recommended during an RSI in order to avoid gastric insufflation and regurgitation, it must be performed if severe hemoglobin desaturation occurs before the trachea is intubated. Neonates should never be allowed to become hypoxic out of concern that mask ventilation might theoretically cause pulmonary aspiration. The incidence of pulmonary aspiration during and after an RSI in infants and children is rare, whereas the incidence of hypoxemia and their harmful consequences is quite frequent. Some advocate mask ventilation to provide safer intubating conditions during RSI in neonates by limiting the peak airway pressures to <10 cmH2O to avoid desaturations without inflating the stomach [148, 149]. This is particularly relevant in the neonate: without preoxygenation, severe desaturation occurs within 10 s of apnea in the healthy neonate. This is often too brief an interval to complete induction of anesthesia, neuromuscular blockade, and tracheal intubation.
Anesthetic Agents for RSI
The optimal combination of anesthetics to achieve a rapid, secure, and successful intubation in the neonate has not been determined. The RSI classically associates a hypnotic and a neuromuscular blocking agent, administered intravenously in rapid sequence in predetermined doses, with a rapid injection and effect. The objective is to minimize the period between the loss of upper airway protective reflexes and tracheal intubation, when the risk of pulmonary aspiration into the unprotected airway is greatest. Most of the drugs used in adults for RSI are considered equally safe and effective in children, even in neonates.
Regarding intravenous hypnotics, thiopental (3–5 mg/kg) or propofol (3–5 mg/kg) can be used for a rapid induction, although for propofol, there is a dearth of studies in very young children. The ED50 for thiopental in neonates (to tolerate a facemask for 30 s) is 3.4 ± 0.2 mg/kg [150]. The ED50 for propofol in neonates has not been determined.
In neonates, thiopental allows a quick and smooth induction of anesthesia and preserves hemodynamic stability [151]. In the first month of life, the dose of thiopental is 45 % less than it is in older infants 1–6 months of age, probably attributable to the reduced protein binding, more permeable blood-brain barrier, and increased brain sensitivity to thiopental [150]. However, the elimination half-life of thiopental in neonates exceeds 14 h, 2.5-fold greater than midazolam [152]. When administered with succinylcholine, thiopental increases the success rate and shortens the time interval to tracheal intubation [128]. Propofol has a time of onset similar to that of thiopental, but a much briefer duration of action, which is an advantage in the context of RSI. Propofol is also more effective than thiopental in limiting the hypertensive response to laryngoscopy in children 1–6 months and to reduce the delay before extubation [153]. Propofol confers two additional advantages: it attenuates airway reactivity and decreases the muscular tension of the jaw muscles. When associated with succinylcholine in healthy children 0–3 months undergoing pyloromyotomy, propofol caused only moderate decease in blood pressure [154]. Propofol may enhance right-to-left shunting in the immediate neonatal period (via a patent foramen ovale, ductus arteriosus), because it reduces systemic vascular resistance more than pulmonary resistance. This may explain the three reports of profound hypotension and prolonged hypoxemia in neonates [155]. When used in preterm neonates (30 weeks gestational age, <8 h postnatal age) in a dose of 1 mg/kg IV bolus, propofol decreased the mean arterial blood pressure by 33 %, from 38 (29–42) mmHg to 24 (22–40) mmHg [156]. This decrease in systemic blood pressure is similar to that associated with one MAC inhalational anesthetics in neonates [157, 158]. Administration of 10–20 ml/kg boluses of balanced salt solution before administration of propofol (and inhalational anesthetics) may attenuate these hemodynamic effects. Pain during IV injection of propofol is common in neonates, leading to a brisk withdrawal of the limb. This may lead to accidental disconnection or loss of the line, at a critical stage of induction. Several strategies have been shown to be effective to attenuate the pain during injection including administration of nitrous oxide by mask and a mini-Bier block with lidocaine [159–161]. In the absence of effective preventative treatment, the anesthesiologist should secure the limb gently while propofol is injected and until the neonate is deeply anesthetized.
In the context of neonatal RSI, the use of ketamine for induction of anesthesia has not been embraced because of its potential to increase systemic blood pressure and cerebral blood flow in premature infants. In the case of neonates who are hemodynamically unstable, intravenous fentanyl has been the preferred agent.
Succinylcholine (2 mg/kg) is the muscle relaxant of choice for RSI because of its rapid onset and brief duration of action [162]. The younger the child, the briefer the duration of the paralysis after succinylcholine [163]. All muscle relaxants, especially succinylcholine [128, 164], greatly improve the conditions for tracheal intubation [165]. However, life-threatening side effects have caused many to fear the use of succinylcholine in young children [121]. Bradycardia may occur after a single dose of IV succinylcholine in infants, but is easily prevented by pretreatment with 0.02 mg/kg IV atropine. More ominously, acute hyperkalemia and rhabdomyolysis may occur after succinylcholine when it is administered to a neonate with an undiagnosed myopathy, such as Werdnig–Hoffman disease or muscular dystrophy. Although these diseases are rare, if electrocardiographic evidence of hyperkalemia occurs after succinylcholine, IV calcium chloride (10 mg/kg), not dantrolene, should be administered intravenously. Malignant hyperthermia (MH) is an exceedingly uncommon disease in neonates, but there may be a family history of MH that will require avoiding succinylcholine and inhalational anesthetics (except nitrous oxide and xenon).
Rocuronium has emerged as the intermediate muscle relaxant of choice in infants and children when succinylcholine is contraindicated or eschewed. Although doses as large as 0.9 mg/kg IV provide excellent conditions for tracheal intubation in less than 1 min, the children in that study were greater than 1 year of age [166]. In contrast to succinylcholine, the duration of action of rocuronium is greater with increasing doses and younger age patients. The optimal dose of rocuronium for RSI in neonates has not been determined. Published studies indicate fairly rapid onset times with 0.45 and 0.6 mg/kg IV rocuronium, although these doses were studied in the presence of inhalational anesthetics [167]. The downside to using such large doses of rocuronium in neonates is the time to recover: 62 and 95 min, respectively [121, 167]. If the surgery is expected to be brief, as in a pyloric stenosis, then the use of large doses of rocuronium will markedly delay emergence and extubation. The prolonged duration of action of rocuronium, then, must be taken into consideration when choosing both the neuromuscular blocking agent and its dose.
With the increasing use of propofol in neonates and during RSI, anticholinergics may continue to play a substantive role. Propofol enhances parasympathetic activity, which can explain the bradycardia often observed after induction of anesthesia. In adults, the decrease in heart rate after propofol in patients who were also receiving a continuous infusion of remifentanil was abolished by pretreatment with 0.5 mg IV atropine [168]. Since cardiac output depends to a large extent on heart rate in neonates, a bradycardia will compromise the cardiac output and decrease tissue oxygenation. In addition, neonates are prone to developing hypoxemia during prolonged apneas such as during RSI. For both of these reasons, intravenous atropine is justified in this population and should be integrated in the standard RSI of infants when using propofol.
Induction of General Anesthesia for Elective Surgery
In the past, anesthetists feared the hemodynamic and respiratory consequences of anesthetic agents in children. Halothane in particular caused profound hypotension, bradycardia, arrhythmias, and apnea. Hypnotics, opioids, and muscle relaxants could produce severe hypotension, tachycardia, bradycardia, dysrhythmia, chest rigidity, or respiratory depression. These could lead to fatal hemodynamic or respiratory failure. To lessen the risk of such adverse events in neonates, practitioners administered the smallest possible doses of these drugs and the most limited number of different drugs. In addition, many older drugs had prolonged durations of action diverse dose-dependent side effects that led to a polypharmacy approach to minimize these effects.
With the introduction of receptor-specific and better-tolerated anesthetics, induction of general anesthesia for elective surgery even in neonate is infrequently met with the past concerns. As cardiopulmonary morbidity has substantively decreased, latent fears have emerged about potential deleterious effects of anesthetics on neurologic development. Currently, these latter fears should not deter the clinician from administering an appropriate anesthetic to a neonate to ensure their safe conduct through the surgery.
Intravenous Induction
Intravenous anesthesia is a common induction technique in neonates globally, since most neonatal surgery occurs in the peripartum period, is associated with a full stomach, and requires an RSI. These neonates arrive in the operating room from the NICU with an IV (or PICC line) in place. Thiopental or propofol is commonly used for induction of anesthesia in a similar dose and with the same limitations as in RSI.
Tracheal intubation can be performed effectively after IV propofol alone, but not so after thiopental or other IV agents [169]. This property of propofol may be attributed to its ability to profoundly depress the laryngeal reflexes and relax the oropharyngeal muscles [170]. When compared with a combination of morphine, atropine, and succinylcholine, propofol 2.5 mg/kg led to a more rapid, successful intubation, less desaturation episodes, and a more rapid recovery time in 63 premature neonates undergoing non-urgent procedures [171]. However, it should be noted that most anesthesiologists would use a larger dose of propofol if it were the only induction agent, the times to intubation were 120 versus 260 s for propofol versus the three drug regimen, times that far exceed those acceptable to anesthesiologists, and all neonates were intubated nasally. Finally, the intubations were performed by registrars, not attending faculty, again raising doubts about the external validity of the data. Similar criticisms hold true for much of the evidence emerging from the neonatology literature [126]. When inducing anesthesia with propofol, the clinician should gently hold the limb where the IV is located, in order to prevent brisk withdrawal movements that might result in loss of the line before all the drugs are injected. The IV site should also be visible at least until all the drugs have been infused. Accidental disconnections can lead to underdosage and airway or hemodynamic reactivity during laryngoscopy. The injection may be done slowly, titrating the optimal dose of hypnotic drugs to the clinical state of the neonate. Pain during injection of propofol is an issue in neonates, even if local anesthetics are added to the propofol. However, it may be attenuated by the prior injection of an opioid (see Anesthetics for RSI, above).
Inhalational Induction
An inhalational induction is a suitable technique to induce anesthesia in the neonate undergoing elective surgery. In this age group, induction is rapid, smooth, technically easy to perform, and pain free. One of its main advantages is that it does not require that IV access be established while the infant is awake, a procedure that can be particularly difficult, prolonged, painful, and stressful for the struggling infant as well as for the anesthesiologist. Sevoflurane is the inhalational agent of choice for induction of anesthesia in infants. Because of its excellent cardiovascular profile, large concentrations of sevoflurane can be administered without causing excessive circulatory depression, unlike halothane. However, theoretical concerns have been raised regarding its potential to trigger epileptiform EEG activity [172]. The probability of epileptiform EEG activity increases in the presence of large concentrations of sevoflurane [173, 174] and hyperventilation and decreases in the presence of midazolam, opioids, and nitrous oxide [173]. Rare instances of tonic–clonic activity have been reported during and after sevoflurane anesthesia (fewer than 20 in the literature), but in only one case in a neonate [175]. In fact, with the exception of a report in one adult, EEG evidence of seizures during sevoflurane anesthesia has not been proven [176]. Severe EEG epileptiform signs during sevoflurane usually occur during deep anesthesia and precede the appearance of burst suppression. Currently, the long-term morbidity of such epileptiform EEG patterns is unknown, although we do know that epileptiform EEG patterns only weakly portend seizures. Given the large number of inhalational inductions performed with sevoflurane in neonates, infants, and children globally and the dearth of reports of seizures and seizure-like activity, the risk and consequences associated with epileptiform EEG activity are probably very minor.
Tracheal intubation can be performed with sevoflurane alone in most infants and children [177]. The main factor associated with the success is to provide sufficient time from the beginning of the inhalational induction until an adequate depth of anesthesia have been achieved, one that is often associated with the loss of spontaneous ventilation [178]. Both positive end-expiratory pressure (10 cmH2O) and assisted ventilation speed the rapid onset of a deep level of anesthesia [179]. Alternately, the time to tracheal intubation may be abbreviated by supplementing the inhalational induction with IV drugs such as propofol, opioids, and muscle relaxants [180]. These strategies have been studied in children and likely may be extended to neonates, although evidence in neonates remains lacking. In neonates, the time to induce anesthesia is rapid; however, with a MAC of 3.2 % sevoflurane, the maximum MAC multiple that can be achieved within the first minute or two of anesthesia is only 1.2 (unlike halothane which can achieve a MAC multiple of 2.5 within the same time) (see Pharmacology chapter). Hence, to speed the induction of deep anesthesia, an IV dose of 2–3 mg/kg propofol may be administered [180].
The analgesic properties and adverse events of all opioids are dose dependent. But each opioid differs in its potency, onset of action, and duration of action.
Fentanyl (1–5 μg/kg) is one of the most frequently used analgesics for tracheal intubation in the NICU [119, 181]. This synthetic opioid has an analgesic potency 50–100 times that of morphine. It is quite lipid soluble and highly bound to plasma proteins. Its onset of clinical activity is approximately 1 min, with a duration of effect after a single IV dose of 30–45 min. Fentanyl’s large hepatic extraction ratio implies that its termination of the action depends on both liver blood flow and CYP450 3A4/7 activity [182]. However, when hepatic blood flow is attenuated, as in several neonatal abdominal pathologies with increased intra-abdominal pressure, the elimination rate may be dramatically reduced (approaching zero), with a correspondingly greater half-life [183, 184].
Fentanyl should be injected slowly IV since it is associated with thoracic rigidity when injected rapidly. It provides excellent conditions for rapid intubation and with few hemodynamic adverse events when it is combined with atropine and a neuromuscular blockade [121, 165, 185]. Whether administered in bolus doses or as a continuous infusion, fentanyl maintains excellent hemodynamic stability [186].
Sufentanil is a synthetic opioid that is ten times more potent than fentanyl. It is highly lipid soluble and strongly bound to alpha-1-acid glycoprotein. In the neonate, the elimination half-life of sufentanil is increased. This is attributable to an increased volume of distribution and diminished clearance [187, 188]. It has an excellent hemodynamic tolerance, even at large doses [189]. Sufentanil can attenuate the cardiovascular response to intubation. Studies performed on a neonatal population are lacking, but some data on children 2–9 years of age, 0.3 μg/kg sufentanil combined with 2.5 mg/kg of propofol, and vecuronium, effectively blunted the cardiovascular responses to tracheal intubation [190]. The ED50 for sufentanil for excellent intubating conditions decreased as the expired fraction of sevoflurane increased in children [191]. For example, at 3 % of sevoflurane, the ED50 was 0.32 μg/kg sufentanil. However, considering the significant pharmacokinetic changes that occur during the first month of life, the extrapolation of these results to neonates should be viewed cautiously.
Although fentanyl and sufentanil remain the most frequent opioids administered to neonates before laryngoscopy and tracheal intubation, even shorter-acting opioids such as alfentanil and remifentanil have been evaluated.
Alfentanil is a synthetic opioid, derived from fentanyl. It is 5–10 times less potent than fentanyl and has a shorter onset and duration of action, mainly because of its reduced volume of distribution. Alfentanil is strongly bound to albumin but also to the plasma alpha-1 acid glycoprotein, a molecule present at reduced concentrations in the plasma of neonates compared with older children or adults [192]. Thus, like sufentanil, its free fraction is increased. The metabolism of alfentanil is mainly via hepatic enzymes, using metabolic pathways that are still immature at birth. In neonates, the elimination half-life is tenfold greater than in infants and children [193], attributable primarily to its decreased clearance [194]. This limits the interest of alfentanil in neonates. In adults, a bolus of alfentanil reduces the hemodynamic response to tracheal intubation, but causes bradycardia at doses in excess of 30 μg/kg [195, 196]. In children aged 3–10 years, the optimal bolus dose for intubation after 1 min exposure to 5 % sevoflurane, in 50 % of the population, was 11.5 μg/kg, without major adverse effects [197]. In children, when combined with propofol, a bolus of 15 μg/kg of alfentanil facilitated a comfortable tracheal intubation and blunted the subsequent hemodynamic response [198, 199]. In neonates, a bolus of 9–15 μg/kg of alfentanil facilitates tracheal intubation and decreases the stress response to that procedure. However, alfentanil may induce a greater incidence of muscle rigidity than other opioids [200].
Remifentanil is the most recent synthetic opioid to become commercially available. In contrast to other opioids, remifentanil is metabolized by nonspecific plasma and tissue esterases, with activities at birth similar to those in adults. Thus, the elimination of remifentanil is rapid and independent of hepatic and renal functions. Its elimination half-life is very brief irrespective of the dose; its context-sensitive half-life is also independent of the age of the child, dose, and duration of infusion, approximately 3–5 min. Side effects associated with bolus doses of opioids may be problematic with IV remifentanil: bradycardia, chest wall rigidity, respiratory depression, nausea and vomiting, and hyperalgesia [121, 201, 202]. Remifentanil is 26- to 65-fold more potent than fentanyl, primarily binding to μ opioid receptors and secondarily to κ and σ receptors. There is no defined dosing in neonates. IV doses range from 1 to 5 μg/kg for boluses and from 0.025 to 5 μg/kg/min for infusions, depending on the concurrent medications [202]. In neonates, a single bolus of 3 μg/kg IV remifentanil for non-urgent intubation yielded less favorable intubating conditions than a combination of fentanyl and succinylcholine [203]. In children 3–10 years of age, the optimal bolus dose of IV remifentanil for successful tracheal intubation during 5 % sevoflurane was 0.56 μg/kg [204]. Remifentanil boluses have also been evaluated as adjuvants to propofol during intravenous induction of anesthesia in infants and children [205]. Although neonates have not been studied as a group separate from infants, the ED50 and ED95 for remifentanil after propofol 5 mg/kg in neonates and infants <3 months of age to provide excellent intubating conditions were 3.1 and 5.0 mg/kg [206]. A continuous infusion of remifentanil has also been recommended to complement sevoflurane during induction of anesthesia. Here too, studies in neonates are lacking. In children 3–10 years old, the ED50 sevoflurane during 0.2 μg/kg/min of remifentanil was 1.81 % [207]. The principle of providing opioid analgesia before intubation seems reasonable, but neither the opioid nor the dose and timing is known to optimize the risk-benefit ratio in the neonate. Nonetheless, it is important to administer sufficient doses of hypnotics, like sevoflurane, to attenuate the nociceptive response to laryngoscopy at a cortical and even subcortical level, even in the absence of analgesics. Thus far, the effects of large concentrations of hypnotics or the combination of hypnotics and opioids during induction of anesthesia in neonates are unknown.
Muscle Relaxants for Tracheal Intubation
All muscle relaxants improve the conditions for tracheal intubation in neonates. However, in most instances in children, relaxants are unnecessary to achieve a quick and stress-free intubation. Many authors advocate relaxant-free techniques for tracheal intubation in children, with appropriate doses of hypnotics and opioids [208]. These drugs inhibit consciousness, blunt the hemodynamic response to nociceptive stimuli, and, in sufficient amount, prevent movement (see below). Given the risks associated with the use of neuromuscular blocking agents, including anaphylaxis and difficulty to antagonize in young infants, clinicians should carefully weigh their risk-benefit ratio in neonates.
Succinylcholine should be used only for rapid sequence inductions and in emergencies (e.g., laryngospasm), because of its potential side effects. For the remainder of circumstances, a non-depolarizing agent can be used.
Infants are more sensitive to non-depolarizing neuromuscular blocking agents (ND-NMB agents) than are older children: fewer than 50 % of the receptors have to be occupied to achieve intubating conditions in young infants compared with 90 % of the receptors in adults [209]. Hence, neonates require smaller doses of succinylcholine to achieve a targeted effect, and the duration of paralysis will be greater than in children [210]. Increasing the amount of injected NMB agent will speed the time to peak effect, but prolong the duration of action of the NMB agent [211].
The advantage of rocuronium is its rapid onset of action: 0.6 mg/kg induced good intubation conditions in 60 s in children anesthetized with propofol [212]. In contrast, rocuronium has a prolonged and unpredictable duration of action in neonates [167]. For a full discussion of the dosing of rocuronium in neonates, see above.
Mivacurium and atracurium have a brief duration of action, but greater time to profound neuromuscular block (2–3 min) than other muscle relaxants. Mivacurium 200 μg/kg IV provides good intubating conditions after 90 s in children [213]. Several studies showed that fentanyl decreased the number of attempts and the incidence of desaturation during tracheal intubation in premature and full-term neonates [185, 214]. Muscle relaxation occurred by 94 s with a time to return of spontaneous movements of ~15 min. Intubating conditions were scored as excellent. However, this protocol did not include any hypnotic drug, and no study compared mivacurium-fentanyl to a hypnotic-fentanyl combination in neonates.
Mivacurium is degraded spontaneously by pseudocholinesterase, leaving no active metabolites. On the other hand, atracurium is degraded spontaneously by Hoffman degradation, a process that depends only on pH and temperature [215]. In patients with a deficiency in plasma pseudocholinesterase (estimated frequency 1/2,000), mivacurium may cause prolonged neuromuscular blockade. In neonates, the activity of these esterases is less than in older patients, approximately 50 % of the adult normal rate. The maturation profile is still unclear: there may be a rapid increase in the pseudocholinesterase in the first month of life and then a slower increase towards adult values [216]. In a neonatal case report of prolonged neuromuscular blockade after an injection of 0.2 mg/kg of mivacurium, plasma levels of cholinesterases were not instructive, and the diagnosis could only be confirmed by molecular investigation [217].
Cisatracurium is one of the ten stereoisomers that comprise the muscle relaxant, atracurium [215]. Cisatracurium induces less histamine release than atracurium. A dose of 0.15 mg/kg provides excellent intubation conditions after 120 s in infants anesthetized with nitrous oxide-thiopental-fentanyl anesthesia [215]. In the same study, the onset time was more rapid and the recovery time greater in younger patients.
Nitrous Oxide
In children, N2O speeds induction of anesthesia with sevoflurane, imparting no foul odor and limited respiratory and hemodynamic adverse effects [218]. However, nitrous oxide may not be ideal for anesthesia in the neonate for the several reasons. First, since many surgeries in neonates are emergencies that involve bowel obstruction or a risk of bowel distention, N2O is often relatively contraindicated. Second, loss of the neonatal airway during induction of anesthesia with N2O speeds the rate of desaturation as the ratio of oxygen consumption to lung oxygen reserve would be very large. Third, N2O has been shown to stimulate opioid and adrenergic centers that activate descending inhibitory neurons (DINS) in adults. These DINS transmit from supraspinal centers in the brain to the posterior horn of the spinal cord. When activated, DINS provide potent antinociception. In the neonate, the DINS are poorly developed and immature [219], thus limiting the analgesic effects of N2O [220]. Fourth, N2O potentiates the neurotoxicity of other anesthetic agents on the developing brain in neonatal animals [20], although there is no evidence that N2O is neurotoxic in humans. Fifth, when administered concomitantly with propofol or sevoflurane, N2O decreases the regional oxygen extraction fraction and creates a possible imbalance in the cerebral metabolic rate [221]. Finally, nitrous oxide impairs the metabolism of vitamin B12, folate, and methionine synthetase that may lead to myelosuppression and megaloblastic anemia [222], although this usually requires a prolonged exposure.
In conclusion, the combination of propofol, opiates, or muscle relaxants to an inhalational induction provides better conditions for tracheal intubation and permits the use of smaller concentrations of sevoflurane, although the optimal doses of these compounds have not yet been determined in neonates.
Tracheal Intubation: Oral or Nasal Route?
The decision to intubate the trachea in the neonate orally or nasally is often an institutional or clinician’s preference, based on local practice and experience.
Oral tracheal intubation is quicker [223] and easier to perform than nasal intubation, thereby reducing the period of apnea and the time interval during which the airway is unprotected. A Cochrane review of nasal versus oral tracheal intubation for the mechanical ventilation of neonates in the neonatal intensive care unit yielded only two studies, thus precluding any definitive recommendations. However, they did note from one of the studies, that hemodynamic responses were similar when hypnosis and analgesia were provided, that failed intubation occurred more frequently after nasal compared with oral intubations, and that atelectasis occurred more frequently after nasal intubation [224].
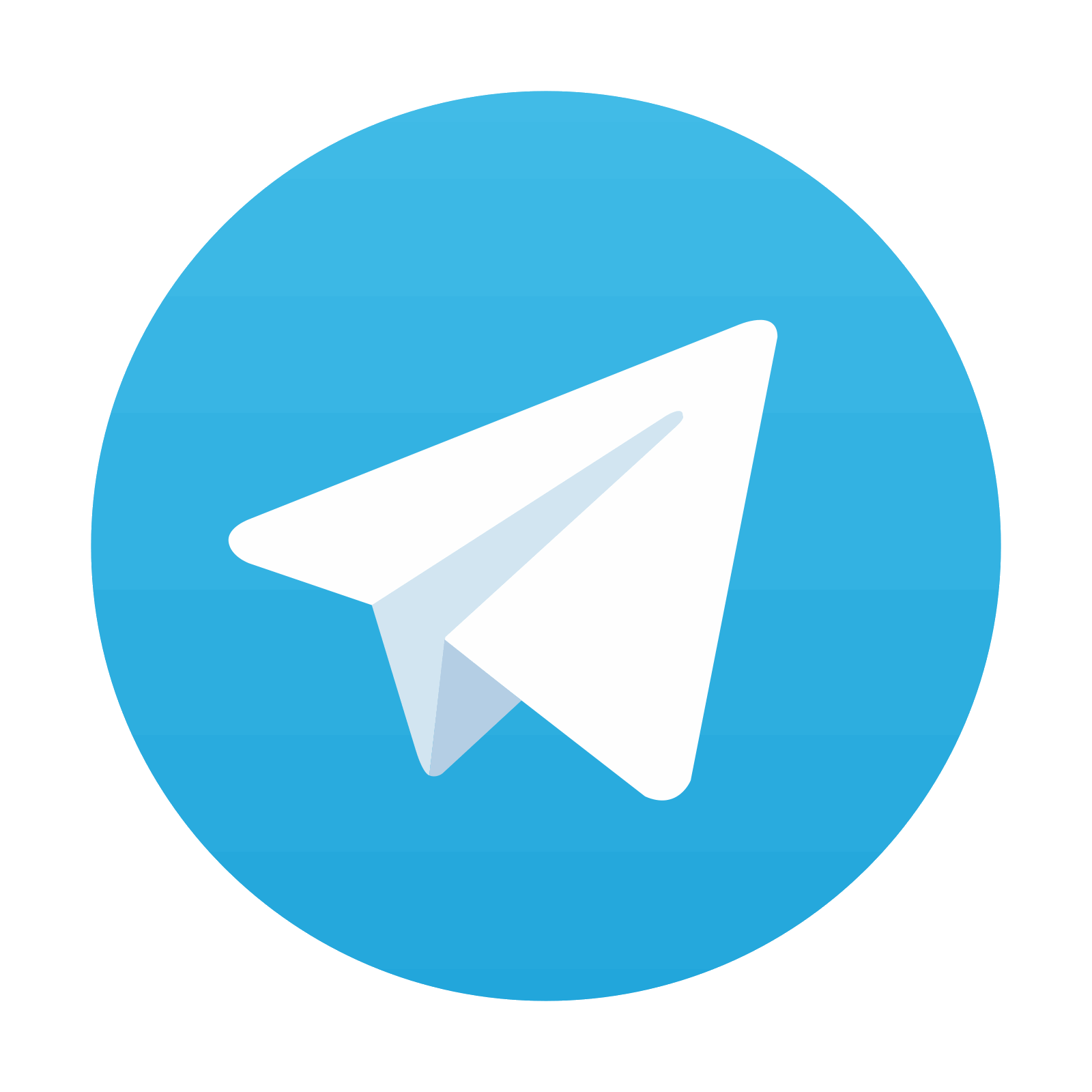
Stay updated, free articles. Join our Telegram channel
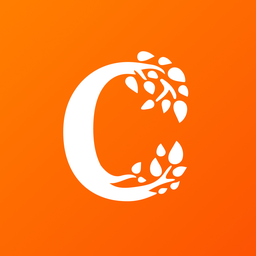
Full access? Get Clinical Tree
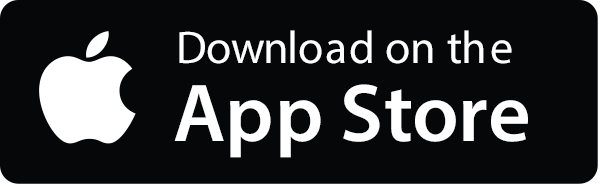
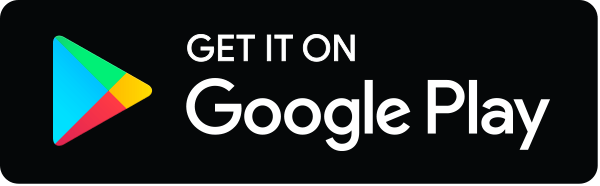
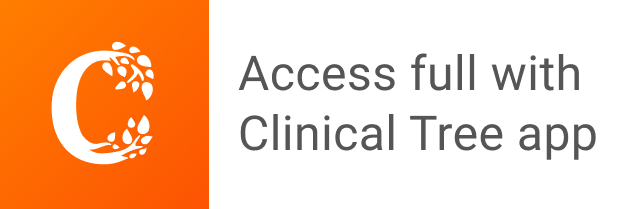