Introduction
The treatment of pain is a fundamental aspect in caring for neonates. Optimal pain management should be individualized and requires an understanding of the neurobiology of pain, developmental analgesic pharmacology, pain assessment, and techniques for providing pain relief.
Developmental Neurophysiology of Pain
There is substantial maturation of peripheral, spinal, and supraspinal neurologic pathways necessary for nociception by late in the second trimester of human gestation [1]. Cutaneous sensory terminals are present in the perioral region at 7 weeks’ gestation and spread to all body areas by 20 weeks’ gestation. Peripheral nociceptive fibers extend into the dorsal spinal cord at 8–10 weeks’ gestation [2]. At birth, A- and C-fiber territories are overlapping in the substantia gelatinosa while cortical descending inhibitory pathways such as the dorsolateral funiculus develops postnatally [3–5]. Neurobiological studies in infant rats and humans show hyper-responsiveness to noxious stimulation compared to older infants [6]. Migration of cortical neurons and establishment of cortico-thalamic connections progresses from 20 weeks’ gestation to term. Myelination extends to the spinal cord and brain stem by 22–30 weeks’ gestation and extends up to the cortex by 37 weeks or term. Research using near-infrared spectroscopy (NIRS) shows activation of the contralateral somatosensory cortex after noxious stimulation in premature infants as young as 25–28 weeks post-menstrual age (PMA) [6,7], though in the youngest of preterm infants the patterns of activation differ little between noxious and non-noxious stimulation. Studies have suggested that infants have the ability to form memory of pain and that there are potentially short- and long-term consequences of untreated pain. Neonatal males circumcised without analgesia had an enhanced pain response to immunizations at two, four, and six months [8]. Infants undergoing major surgery who received deeper anesthetic techniques had improved suppression of stress response compared to infants who received lighter anesthesia [9]. Conversely, in a prospective randomized trial, the routine administration of morphine infusions to critically ill infants receiving mechanical ventilation had no clear benefits compared to intermittent morphine boluses for painful procedures [10,11]. Infant rats exposed to repeated noxious stimuli showed increased responses to painful stimuli and had loss of nociceptive primary afferents compared to control infant and adult rats [12]. Studies of pain-conditioned behaviors in adult rats who had persistent noxious stimuli as neonates showed a decrease in pain threshold and re-organization in the dorsal horn structure [13]. A study of extremely low birth-weight-infants who experienced painful stimuli in the neonatal intensive care unit (NICU) had increased somatic complaints as toddlers compared to a control group of children who had been full-term healthy neonates [14]. In general, patterns of opioid dosing for neonates undergoing intensive care have changed considerably over the past 20 years, and it remains difficult to recommend a particular dosing regimen as better than others, both for the immediate aim of reducing pain and distress and the longer term aim of minimizing adverse effects on neurodevelopment.
Pain Assessment in Neonates
Pain assessment in neonates and other vulnerable populations is particularly challenging. Infants show predictable response pain patterns to pain with respect to stress hormone levels, behavior patterns and changes in physiologic parameters. Nevertheless, physiologic and behavioral parameters can be nonspecific indicators and may not necessarily reflect measures of pain. Studies using NIRS have attempted to evaluate whether pain assessment tools reflect cortical pain responses. A study by Slater et al. showed an overall fair correlation between the Premature Infant Pain Profile (PIPP) and cortical activity for infants undergoing heel lances; however, cortical pain responses were recorded in some infants who did not display facial expression of pain, concluding that infants with low pain scores may not necessarily be pain-free [15]. Physiologic measures of pain can be confounded by autonomic activation unrelated to pain, particularly in infants who are critically ill and receiving vasopressors. Behavioral responses are unavailable as measures during neuromuscular blockade. There are several pain assessment tools that have been studied in neonates [16–19]. Most tools are easily used at the bedside and combine behavioral parameters such as crying, facial expression, and posture with physiologic parameters such as blood pressure and heart rate. Pain assessment tools most commonly used for neonates include the PIPP [16], the Neonatal Infant Pain Scale (NIPS) [17] and Neonatal Pain Agitation and Sedation Scale (N-PASS) [18], and the CRIES scale (Crying, Requires oxygen, Increased vital signs, Expression, and Sleeplessness) [19] (see Tables 35.1–35.4). Currently used neonatal pain assessment tools may not be reliable for neonates experiencing persistent, untreated pain since neonates will often have an attenuation of their hemodynamic response and behavior in response to persistent pain [20,21].
Assessment Criteria | Sedation | Normal | Pain/agitation | ||
---|---|---|---|---|---|
–2 | –1 | 0 | 1 | 2 | |
Crying/irritability | No cry with painful stimuli | Moans or cries minimally with painful stimuli | Appropriate cryingNot irritable | Irritable or crying at intervalsConsolable | High-pitched or silent-continuous cryInconsolable |
Behavior state | No arousal to any stimuliNo spontaneous movement | Arouses minimally to stimuliLittle spontaneous movement | Appropriate for gestational age | Restless, squirmingAwakens frequently | Arching, kickingConstantly awake ORArouses minimally/no movement (not sedated) |
Facial expression | Mouth is laxNo expression | Minimal expression with stimuli | RelaxedAppropriate | Any pain expression is intermittent | Any pain expression is continual |
Extremities tone | No grasp reflexFlaccid tone | Weak grasp reflexReduced muscle tone | Relaxed hands and feetNormal tone | Intermittent clenched toes, fists or finger splayBody is not tense | Continual clenched toes, fists or finger splayBody is tense |
Vital sign: HR < RR, BP, SaO2 | No variability with stimuliHypoventilation or apnea | <10 percent variability from baseline with stimuli | With baseline or normal for gestational age | Increased 10–20 percent from baselineSaO2 increases quickly with stimulation | Increased >20 percent from baselineSaO2 < 75 percent and increases slowly with stimulationOut of sync with ventilation |
Adjustment:
-
+3 if less than 28 weeks’ gestational age
-
+2 if 28–31 weeks’ gestational age
-
+1 if 32–35 weeks’ gestation age
Indicators | Score | |||
---|---|---|---|---|
0 | 1 | 2 | 3 | |
Gestational age | 36 weeks or more | 32–35weeks + 6 days | 28–31 weeks + 6 days | Less than 28 weeks |
Behavioral state | Active, awake, eyes open, facial movements | Quiet, awake, eyes open, no facial movements | Active, awake, eyes closed, facial movements | Quiet, asleep, eyes closed, no facial movements |
Heart rate maximum | 0 bpm increase | 5–15 bpm increase | 15–24 bpm increase | 24 bpm increase |
O2 sats | 92–100 percent | 89–91 percent | 85–88 percent | 84 percent or less |
Brow bulge | None | Minimum | Moderate | Maximum |
Eye squeeze | None | Minimum | Moderate | Maximum |
Naso-labial furrow | None | Minimum | Moderate | Maximum |
Indicator | Score | ||
---|---|---|---|
0 | 1 | 2 | |
Facial expression | Relaxed – restful face, neutral expression | Grimace – tight facial muscles, furrowed brow, chin, jaw | – |
Cry (if infant is intubated, score silent cry based on facial movement) | No cry | Whimper – mild moaning, intermittent | Vigorous crying – loud screaming, shrill, continuous |
Breathing pattern | Relaxed – usual pattern for this infant | Change in breathing – irregular, faster than usual, gagging, breath holding | – |
Arms | Relaxed – no muscular rigidity, occasional random movements | Flexed/extended – tense, straight arms, rigid and/or rapid extension, flexion | – |
Legs | Relaxed – no muscular rigidity, occasional random movements | Flexed/extended – tense, straight arms, rigid and/or rapid extension, flexion | – |
State of arousal | Quiet, peaceful sleeping or awake, alert and settled | Fussy – alert, restless, and thrashing | – |
Indicator | Score | ||
---|---|---|---|
0 | 1 | 2 | |
Crying | No | High pitched | Inconsolable |
Requires oxygen | No | <30 percent | >30 percent |
Increased vital signs | Heart rate and blood pressure less than or equal to baseline | Heart rate and blood pressure increase of less than 20 percent from baseline | Heart rate and blood pressure increase more than 20 percent from baseline |
Expression | None | Grimace | Grimace/grunt |
Sleeplessness | No | Wakes at frequent intervals | Constantly awake |
Principles of Developmental Analgesic and Local Anesthetic Pharmacology
There are several pharmacokinetic and pharmacodynamic factors that produce age-related differences in responses to analgesics [22]. Neonates and young infants have immature hepatic enzyme systems involved in conjugation, glucuronidation, and sulfation of opioids and amide local anesthetics, which results in prolongation of elimination half-life and increased risk of drug toxicity. Most neonates and young infants will have maturation of hepatic enzyme systems involved in biotransformation and conjugation by the age of six months; however, enzyme maturation rates can vary. Pharmacogenomic differences can further introduce variability in hepatic metabolism of opioids. This effect is especially prominent for codeine, which acts as a pro-drug and provides analgesia only with conversion to morphine [23]. Glomerular filtration and renal tubular secretion are reduced in the first several weeks of life, resulting in slower elimination of many renally eliminated drugs and active metabolites of drugs that have undergone hepatic metabolism. Delayed elimination of glucuronides of morphine and hydromorphone can lead to sedation, analgesia, and respiratory depression. Delayed renal clearance of MEGX, a primary metabolite of lidocaine, can have neuro-excitatory effects. Neonates and young infants have decreased levels of α1-acid glycoprotein and albumin, which results in decreased plasma protein binding for many drugs including amide local anesthetics and opioids. Reduced plasma protein binding can result in increased concentrations of pharmacologically active, unbound drug, and increased risk of drug toxicity. Infants have immature ventilatory reflexes in response to hypoxia [24,25] and hypercarbia [26], increasing the risk of apnea associated with opioids. Abnormal ventilatory reflexes may persist through the first year of life in some infants with chronic lung disease and in some with abnormal brainstem development, including some infants with myelomeningocele and Arnold–Chiari malformation [27].
Management of Pain
Nonpharmacologic Approaches
Infants experience a number of routine and often daily painful procedures such as endotracheal suctioning and heel lances. In general, attempts should be made to reduce the number of painful events, but in many cases painful interventions such as endotracheal suctioning are necessary for optimal care [28]. Several studies have shown benefit of nonpharmacologic therapies such as sucrose, facilitated tucking and kangaroo care with respect to reduction of pain scores during mildly painful routine procedures in neonates [29–31]. Current American Academy of Pediatrics (AAP) guidelines recommend the use of nonpharmacologic methods to reduce pain from minor routine procedures in neonates [28]. A study of facilitated tucking during endotracheal tube (ETT) suctioning showed that the facilitated tucking group had significantly lower PIPP scores compared to a control group [32]. There is some controversy over whether sucrose’s action should be regarded as a “calming” action or an analgesic action per se [33]. A randomized controlled trial showed that sucrose, especially when combined with non-nutritive sucking, was effective in providing analgesia for heel-sticks in newborns [34]. However, a controlled trial using electroencephalography in neonates to measure pain-specific brain activity evoked by a heel lance showed that sucrose did not significantly affect activity in the neonatal brain, concluding that reductions in observational pain scores with sucrose should not necessarily be interpreted as pain relief [33]. In addition, there is controversy about the long-term effect of frequent dosing of oral sucrose in preterm infants and the developing brain [35,36].
Pharmacologic Approaches
Acetaminophen
Acetaminophen is one of the most widely used analgesics, although its actual mechanism of analgesic and antipyretic action remains uncertain. Putative targets of acetaminophen have included a range of peripheral, spinal, and supraspinal sites, including cox-isoenzymes, TRPV1, and TRPA1 receptors and cannabinoid receptors. Acetaminophen exerts little anti-inflammatory effect and is associated with significantly less gastropathy, platelet dysfunction, and renal dysfunction than NSAIDs. The majority of acetaminophen undergoes sulfation and glucuronidation to renally eliminated water-soluble products. There are limited data on hepatic enzyme profiles of acetaminophen in neonates, but preliminary studies show good hepatic tolerance [37]. A small portion of acetaminophen is metabolized by the CYP450 system to a toxic metabolite that binds to glutathione to form a non-toxic conjugate. Inadvertent overdosing errors have led to fulminant hepatic necrosis. Some initial analgesic trials with either oral or rectal acetaminophen in newborns were negative. More recently, a randomized controlled trial by Ceelie et al., showed that postoperative use of intermittent IV acetaminophen resulted in lower cumulative morphine dose in the first 48 hours in neonates and infants undergoing thoracic and abdominal surgery with no difference in pain scores or adverse effects between groups [38]. Conversely, a recent systematic review of the use of acetaminophen for the treatment of pain in neonates concluded there was lack of data for efficacy and that additional trials are needed to determine efficacy [39]. Despite the negative conclusion of this Cochrane review, we continue to prescribe acetaminophen for neonates undergoing major surgery. In our view several factors may have contributed to false-negative conclusions in some of the trials pooled in the Ohlsson systematic review: (1) overly generous rescue opioid dosing washing out between-group differences; (2) slow and inefficient absorption following rectal dosing; and (3) insensitivity of measures and the heterogeneity of behavioral and physiologic pain ratings in studies of postoperative neonates where some are receiving mechanical ventilation and others are not. There remains a possibility the target(s) of acetaminophen are not yet developed or connected in neonates, analogous to the studies by Ririe et al. [40] for NSAIDs described in the next section. Nevertheless, we believe that acetaminophen has a favorable safety profile in comparison to several other analgesics in neonates, and this factor supports its use for post-surgical pain.
Pharmacokinetic studies of intravenous acetaminophen show reduced clearance with younger gestational age infants, with clearance at 27 weeks’ PMA less than half that of a full-term newborn [41,42]. Recommended doses are found in Table 35.5. There is inadequate pharmacokinetic data for infants younger than 28 weeks’ PMA to allow for safe dosage recommendations.
Table 35.5 Acetaminophen dosing
Intravenous dosing | |
PMA 28–32 weeks | 10 mg kg–1 dose–1 every 12 hours (max. 22.5 mg kg–1 day–1) |
PMA 33–36 weeks | 10 mg kg–1 dose–1 every 8 hours (max. 40 mg kg–1 day–1) |
PMA ≥ 37 weeks | 10 mg kg–1 dose–1 every 6 hours (max. 40 mg kg–1 day–1) |
Oral dosing | |
PMA 28–32 weeks | 10–12 mg kg–1 dose–1 every 6–8 hours; max. daily dose: 40 mg kg–1 day–1 |
PMA 32–37 weeks and term neonates <10 days | 10–15 mg kg–1 dose–1 every 6 hours; max. daily dose: 60 mg kg–1 day–1 |
Term neonates ≥10 days | 10–15 mg kg–1 dose–1 every 4–6 hours; max. daily dose: 75 mg kg–1 day–1 |
Rectal dosing | |
PMA 28–32 weeks | 20 mg kg–1 dose–1 every 12 hours; max. daily dose: 40 mg kg–1 day–1 |
PMA 32–37 weeks and term neonates <10 days | Loading dose: 30 mg kg–1; then 15 mg kg–1 dose–1 every 8 hours; max. daily dose: 60 mg kg–1 day–1 |
Term neonates ≥10 days | Loading dose: 30 mg kg–1; then 20 mg kg–1 dose–1 every 6–8 hours; max. daily dose: 75 mg kg–1 day–1 |
Nonsteroidal Anti-Inflammatory Agents
Nonsteroidal anti-inflammatory agents reversibly inhibit the actions of COX enzymes, which are responsible for the conversion of arachidonic acid to prostaglandins that have diverse roles contributing to pain, inflammation, gastric mucosal integrity, vasodilation, and hypersensitivity. There are very few safety or efficacy data on the use of NSAIDs in newborns and premature infants. A clinical trial of 70 preterm infants less than 37 weeks received either high- or low-dose ibuprofen for patent ductus arteriosus (PDA) closure; there were no gastrointestinal, hematologic, or renal adverse effects reported [43]. A recent systematic review concluded that compared to indomethacin, ibuprofen was associated with reduced risk of necrotizing enterocolitis and transient renal insufficiency [44]. There are animal data showing that intraspinal and systemic administration of a COX-1 inhibitor in infant rats produced no reduction in response to incisional pain; infant rats, unlike more mature rats, had no increase in COX-1 protein in the spinal cord [40]. This raises the question of whether COX-inhibitors are effective in infants due to developmental differences in COX-enzyme expression in the spinal cord microglia [40].
Ketorolac is a commonly prescribed parenteral NSAID often used for postoperative pain in older infants and children. There are minimal efficacy data, pharmacokinetic data, and conflicting safety data on the use of ketorolac in neonates and preterm infants. In a randomized controlled trial of infants and children undergoing cardiac surgery, ketorolac did not increase the risk of surgical bleeding or gastrointestinal bleeding [45]. However, other studies have found increased risk for bleeding events in infants younger than 21 days and less than 37 weeks’ PMA who received ketorolac after surgery [46]. Small case series have not reported harm with use after surgeries in neonates and young infants [47,48], though larger prospective studies and pharmacokinetic studies are needed to better define the risks of NSAIDs in neonates and premature infants. Recommended doses are found in Table 35.6.
Table 35.6 Doses of non-steroidal anti-inflammatory drugs
Dose | |
---|---|
Ibuprofen | 5–10 mg kg–1 dose–1 every 6–8 hours; maximum dose 40 mg kg–1 day–1 |
Ketorolac | 0.5 mg kg–1 dose–1 every 6 hours for a maximum of 12 doses |
Dosing guidelines refer to infants under six months of age.
Ibuprofen and ketorolac at Q8–12 hour dosing intervals have been used in neonates and young infants but there are limited safety and efficacy data in infants under six months.
Opioid Therapy
Morphine
Opioids such as morphine are commonly used in the treatment of pain in neonates. Opioid receptors are widely distributed in the forebrain, brainstem, and spinal cord by mid-gestation; functional maturation of opioid receptors occurs prenatally and continues postnatally. Several studies have shown beneficial as well as adverse short-term effects with morphine. Long-term outcome studies among former preterm neonates have also shown mixed results [49,50]. Results from the Neurologic Outcomes and Pre-emptive Analgesia in Neonates (NEOPAIN) trial showed no differences in mortality, intraventricular hemorrhage (IVH), or periventricular leukomalacia in ventilated preterm neonates randomly assigned to receive either morphine infusions or placebo, and there was no evidence of benefit on distress measures [51]. A randomized trial by Simons et al. showed no differences in analgesia between ventilated preterm infants receiving morphine infusion vs. placebo; although the morphine group had a decreased incidence of IVH, there were no differences in poor neurologic outcomes, leading to the conclusion that routine morphine infusions are not warranted in ventilated preterm neonates [52]. Morphine appears to be effective for acute postoperative pain in preterm neonates, but there is evidence suggesting it does not provide adequate analgesia for procedural pain such as endotracheal tube suctioning or heel lances [53,54]. There is evidence that morphine may improve respiratory synchrony in ventilated neonates; however, a randomized trial showed that morphine infusions in preterm infants with respiratory distress syndrome did not improve short-term pulmonary outcomes among ventilated preterms [10,55].
In a long-term follow-up study of former ventilated preterm infants aged 5–7, those who received preemptive morphine had smaller head circumference, more social problems, and performed more poorly in memory tasks than those who had received placebo; however, there were no differences in overall IQ or academic performance [56]. A study by Valkenburg et al. found continuous morphine infusions in former preterm neonates at ages 8 or 9 years had no long-term adverse effects on quantitative sensory testing, the development of chronic pain, or overall neurologic functioning [57].
Morphine metabolism occurs in the liver through glucuronidation to morphine-3-glucuronide, which has excitatory action, and morphine-6-glucuronide, which has analgesics, sedative, and respiratory depressant actions. Both are renally eliminated and can accumulate in renal insufficiency. A randomized trial of 68 neonates receiving either intermittent doses or continuous infusions of morphine showed that younger neonates had significantly reduced postoperative morphine requirements and seemed to preferentially metabolize morphine to morphine-3-glucuronide [58]. The clearance of morphine is reduced in preterm neonates compared to term neonates [54], and is less than half that measured in adults. Dosing should be adjusted based on age, weight, and side-effects since there is considerable individual variation in pharmacokinetics. For nonventilated neonates, doses should be titrated with careful monitoring of respiratory status since neonates can develop apnea in response to hypoxia and hypercarbia. Typical starting doses for intermittent morphine bolus dosing is 0.02 mg kg–1and 0.015 mg kg–1 h–1 for continuous infusions (see Table 35.7).
Table 35.7 Typical opioid starting doses
Typical starting opioid intravenous bolus doses and intervals | Typical starting opioid intravenous infusion doses | Typical starting opioid oral doses and intervals | |
---|---|---|---|
Morphine | 0.025–0.1 mg kg–1every 2–4 h | 0.01–0.03 mg kg–1 h–1 | 0.15–0.3 mg kg–1 every 3–4 h |
Hydromorphone | 0.005–0.02 mg kg–1 every 2–4 h | 0.002–0.006 mg kg–1 h–1 | 0.02–0.04 mg kg–1 every 3–4 h |
Fentanyl | 0.25–1.0 μg kg–1 every 1–2 h | 0.5–2.0 μg kg–1 h–1 | N/A |
Effective starting doses vary considerably.
All doses are approximate and should be adjusted according to clinical circumstances with careful titration and close monitoring for side effects.
Dose reductions are recommended for premature infants and non-ventilated infants.
Fentanyl
Fentanyl undergoes metabolism in the liver primarily by CYP3A4 to inactive metabolites, and can be useful for patients with renal failure. The effect of a single dose of fentanyl is terminated by redistribution rather than metabolism, whereas the effect of continuous infusion is terminated more by metabolism and can have a prolonged duration of action. The context-sensitive half-life of fentanyl in neonates is particularly prolonged [59]. Fentanyl can induce glottic and chest wall rigidity, particularly in neonates; treatment can consist of neuromuscular blockade and controlled ventilation; naloxone is variably effective. A randomized controlled trial showed that ventilated newborns who received fentanyl infusions had lower pain scores but longer controlled ventilation time compared to a placebo group who received intermittent fentanyl doses [60]. Other opioids such as remifentanil are sometimes used for brief painful procedures, but there are few data on efficacy or safety [61].
Hydromorphone
Hydromorphone has a similar duration of action to morphine and can be used in nurse-controlled analgesia and epidural analgesia. Because of its greater potency compared to morphine, dose titration of hydromorphone is more difficult in young infants and smaller weight patients. Hydromorphone is frequently used in patients with renal insufficiency; however, there is little supportive evidence and active metabolites for both morphine and hydromorphone can be associated with neurotoxic effects. Because hydromorphone is more hydrophilic than fentanyl, we generally avoid using hydromorphone-based epidural solutions in young infants due to the risk of respiratory depression. See Table 35.7 for typical opioid starting doses.
Tolerance and Withdrawal
A consequence of prolonged opioid therapy in critically ill infants is the development of opioid tolerance and subsequent risk of withdrawal as opioids are withdrawn. Primary factors that affect the development of opioid tolerance include age and duration of opioid therapy. The function of opioid and NMDA receptors, regulation of G-protein signaling and other components of opioid signaling systems undergo age-related changes [62]. Evidence suggests that infants are more vulnerable to developing opioid tolerance than children and adults [63]. Evidence from a recent multicenter trial evaluating the efficacy of protocolized sedation for critically ill infants and children showed that adherence to a goal-directed comfort algorithm resulted in fewer days of opioid administration and exposure to fewer drug classes, and may help in preventing tolerance by allowing for proactive weaning of opioids when clinical condition permits [64]. Elements of the sedation protocol included daily team discussion of the patient’s illness trajectory and adjustment of pain and sedation medications at least every eight hours. Patients who received opioids for less than five days were able to have their opioids discontinued without weaning or signs of withdrawal. Every eight hours adjustments in pain and sedation medications were well tolerated and there were no differences in occurrence of iatrogenic withdrawal between the protocolized group and the group who received usual ICU care. Clonidine is an alpha-2-agonist that attenuates the noradrenergic effects of withdrawal, and can be useful in preventing withdrawal symptoms as opioids are weaned. A controlled trial of clonidine in ventilated newborns showed that clonidine reduced fentanyl requirements with no difference in frequency of side-effects [65].
Methods of Opioid Administration
Intermittent parenteral dosing of opioids can be useful for episodic pain or as a loading dose to achieve a rapid rise in plasma opioid concentration. However, intermittent dosing results in wide variations in plasma opioid concentrations and subsequent pain intensity and side effects. Continuous infusions are often used in neonates and infants who are mechanically ventilated with additional boluses of opioids given for endotracheal tube suctioning and painful procedures. A continuous infusion provides a steady plasma opioid concentration with near-constant level of analgesia; however, this does not take into account fluctuations in pain intensity as with chest physiotherapy or coughing. Patient-controlled and nurse-controlled analgesia takes into account both individual variations in opioid pharmacokinetics and pain intensity as well as provides ease of opioid administration. Morphine is more commonly used in NCA in young infants. Cardiorespiratory monitoring should be used for all patients receiving continuous infusions and for patients at risk for opioid-induced respiratory depression. See Table 35.8 for typical NCA starting doses.
Table 35.8 Typical starting doses for nurse-controlled analgesia
Bolus dose | Continuous rate | 1-hour limit | |
---|---|---|---|
Morphine | 0.02 mg kg–1 | 0.005–0.015 mg kg–1 h–1 | 0.1 mg kg–1 |
Hydromorphone | 0.005 mg kg–1 | 0.001–0.003 mg kg–1 h–1 | 0.02 mg kg–1 |
Fentanyl | 0.25 μg kg–1 | 0.15 μg kg–1 h–1 | 1 μg kg–1 |
Effective starting doses vary considerably.
All doses are approximate and should be adjusted according to clinical circumstances with careful titration and close monitoring for side effects.
Dose reductions are recommended for premature infants and non-ventilated infants.
Regional Analgesia
Epidural analgesia and continuous peripheral nerve blockade can provide excellent postoperative analgesia for infants undergoing a variety of surgical procedures, including thoracic, lower extremity, and abdominal procedures. Regional analgesia can reduce overall opioid use, thereby reducing opioid side-effects and the development of tolerance. A detailed discussion of regional blocks can be found in Chapter 36. To avoid direct needle placement in the thoracic region and associated risk of neurologic complications, an alternative method of epidural catheter placement is to advance catheters from the caudal and lumbar regions cephalad to thoracic dermatomes. This may be performed blindly, with guidance by fluoroscopy, ultrasound [66], and nerve stimulation [67]. Blind placement without confirmation is generally not recommended when advancing catheters cephalad since there is a 30 percent failure associated with blind placement. The clearance of amide local anesthetics is much slower in infants and lower local anesthetic infusion rates are necessary to avoid systemic toxicity. Because of the limitations of using safe local anesthetic infusion rates, it is crucial in infants to place epidural catheter tips in the correct surgical dermatome. A radio-opaque catheter can be used and visualized while advancing cephalad using fluoroscopy. Another method of radiographic confirmation is to inject a small volume of radiocontrast dye (0.5–1 ml) through the epidural catheter and observe proper tip location on a plain radiograph (epidurogram).
It is sometimes necessary to confirm proper position of an epidural catheter postoperatively for patients who have pain despite adequate dosing of epidural infusions. Due to concerns of toxicity with amide local anesthetics, one method is to confirm epidural position by administering a test dose of 2 percent chloroprocaine in incremental doses and observing for behavioral signs of pain relief such as relaxed posture and ceasing of crying as well as more objective signs such as return of blood pressure and heart rate to baseline. Alternatively, an epidurogram using radiocontrast dye can also be used to confirm epidural catheter position postoperatively.
Epidural drug selection should be individualized and varies with surgical and patient-specific factors. In most cases, we recommend infusions of local anesthetics in combination with opioids and/or clonidine due to the synergistic effect of combining local anesthetics with adjuvants. Pharmacokinetic studies measuring unbound, pharmacologically active bupivacaine in infants receiving continuous epidural bupivacaine infusions showed plasma bupivacaine levels rise and can accumulate to reach toxic levels after the first 48 hours, despite increases in serum α-1-acid glycoprotein levels [68,69]. Based on these pharmacokinetic studies, epidural bupivacaine infusion rates in neonates and infants younger than four months should be limited to 0.2 mg kg–1 h–1. In a study by Olsson, however, a substantial number of neonates had a continued rise in serum bupivacaine levels at 48 hours even with limiting the dose to 0.2 mg kg–1 h–1 [70]. Epidural bupivacaine infusion rates of 0.4 mg kg–1 h–1 appear to be safe for children over the age of six months. Pharmacokinetic data of ropivacaine in infants show that clearance is reduced in neonates and young infants compared to older children [71–73]. Ropivacaine infusion rates up to 0.2 mg kg–1 h–1 appear to be safe in neonates, although pharmacokinetic data are limited [74].
Because of the risks and limitations in dosing of amide local anesthetics in neonates and young infants, the amino ester local anesthetic chloroprocaine can be used as an alternative local anesthetic for epidural and peripheral nerve catheter infusions. Studies of continuous epidural infusions of chloroprocaine showed good surgical anesthesia [74–76].
The addition of opioids to epidural solutions can potentiate analgesia but may increase the likelihood of respiratory depression. Because of the increased risk of cephalad spread and potential for respiratory depressant effects, we rarely use hydromorphone neuraxially in nonventilated infants less than four months of age. Fentanyl is used selectively in epidural infusions in infants less than four months of age, although there remains some risk of respiratory depression. Clonidine can prolong and enhance the analgesic effect of local anesthetics. Although continuous epidural infusions containing dilute solutions of clonidine tend to be well tolerated without respiratory depression or hypotension in neonates, there have been reports of apnea associated with bolus dosing of clonidine in caudal blocks in preterm infants [77]. Due to the increased risk of toxicity in neonates and potential accumulation of amide local anesthetics during continuous infusions, our practice is to use chloroprocaine-based solutions for peripheral nerve catheter and epidural catheter infusions in neonates (see Tables 35.9 and 35.10).
Table 35.9 Recommended neonatal and infant peripheral nerve catheter infusion rates
Solution | <2 months | 2–6 months | >6 months |
---|---|---|---|
Ropivacaine 0.1% | 0.2 ml kg–1 h–1 | 0.3 ml kg–1 h–1 | 0.3–0.4 ml kg–1 h–1 |
Chloroprocaine 1.5% | 0.5–0.8 ml kg–1 h–1 |
Consider chloroprocaine-based infusions due to risk of
accumulation and toxicity of amide local anesthetics in neonates.
Little information is available on how best to adjust these rates based on degrees of prematurity.
Infusion rates and solutions should be modified according to
clinical circumstances.
Monitoring and close observation are recommended.
Table 35.10 Recommended neonatal and infant epidural infusion rates
Epidural solution | <2 months | 2–6 months | >6 months |
---|---|---|---|
Bupivacaine 0.1% ± fentanyl 0.3 μg ml–1 ± clonidine 0.05 μg ml–1 | 0.2 ml kg–1 h–1 | 0.2 ml kg–1 h–1 | |
Ropivacaine 0.1% ± fentanyl 0.3 μg ml–1 ± clonidine 0.05 μg ml–1 | 0.2 ml kg–1 h–1 | 0.3 ml kg–1 h–1 | |
Chloroprocaine 1.5% ± fentanyl 0.3 μg ml–1 ± clonidine 0.05 μg ml–1 | 0.2–0.5 ml kg–1 h–1 thoracic0.5–0.8 ml kg–1 h–1 lumbar | 0.2–0.5 ml kg–1 h–1 thoracic0.5–0.8 ml kg–1 h–1 lumbar | |
Bupivacaine 0.1% ± fentanyl 2 μg ml–1 ± clonidine 0.4 μg ml–1 | 0.2 ml kg–1 h–1 | 0.3–0.4 ml kg–1 h–1 | |
Ropivacaine 0.1% ± fentanyl 2 μg ml–1 ± clonidine 0.4 μg ml–1 | 0.3 ml kg–1 h–1 | 0.3–0.4 ml kg–1 h–1 | |
Ropivacaine 0.1% ± hydromorphone 10 μg ml–1 ± clonidine 0.4 μg ml–1 | 0.3–0.4 ml kg–1 h–1 |
Consider chloroprocaine-based infusions due to risk of accumulation and toxicity of amide local anesthetics in neonates; little information is available on how best to adjust these rates based on degrees of prematurity.
Rates shown reflect upper end of usual infusion rates.
Infusion rates and solutions should be modified according to clinical circumstances; solutions containing hydrophilic opioids may increase the risk of respiratory depression.
Cardiorespiratory monitoring and close observation are recommended for all patients receiving epidural infusions.
References
Pain management is especially challenging in neonates and, increasingly, regional anesthesia is being employed as a major pain management technique for neonates and young infants. The implementation of multimodal analgesia with the inclusion of regional anesthesia may be especially important in neonates, where the side-effects of opioids are common and frequently severe. The majority of regional anesthetic techniques provided to neonates are blocks of the neuraxis; although most of these are single-shot caudals, the use of peripheral nerve blocks is increasing in neonatal anesthesia practice [1–3]. Safety must always be a primary consideration and existing safety data are overall reassuring, with the incidence of serious complications reported in neonates at 1 percent [3,4]. While all of the complications occurred in patients having neuraxial blocks, and consisted mostly of dural punctures and total spinals with transient apnea, there were no reports of permanent sequellae. No complications occurred in neonates receiving peripheral nerve blocks, suggesting an added safety advantage of peripheral nerve blocks compared to neuraxial. These data mirror the results found in studies of older children [3].
Regional anesthesia can be performed in neonates for operative anesthesia, postoperative analgesia, or the treatment of painful nonoperative traumatic or medical conditions. Contraindications to peripheral nerve blocks in neonates are similar to those identified in older children and consist of absolute allergy to local anesthetic agents, guardian refusal, or infection at the insertion site. Although coagulopathy and treated bacteremia are not necessarily absolute contraindications, when present, the risk and benefits of the peripheral nerve block need to be carefully considered.
Most neonates need to be unconscious when the peripheral nerve block is inserted, and there is a good record of safety for performing regional anesthesia on infants while heavily sedated or under general anesthesia. Regardless of the type of sedation, all infants must have standard monitoring in place and resuscitation equipment must be immediately available when regional anesthesia is being administered. If the block is performed with the infant awake, topical or local anesthesia should be applied to the injection site(s) and a sucrose pacifier, although providing no analgesia, may give the infant comfort.
Anatomic and Developmental Differences
Myelination of peripheral nerve axons begins in utero but is not complete until 1–2 years of age. This relative lack of myelination allows the nerves to be more easily blocked with local anesthetics. Lower volumes – for example 0.1–0.2 ml kg–1– of bupivacaine and ropivacaine solutions are usually sufficient to block most peripheral nerves.
Ultrasound has become an important adjunct in regional anesthesia and the use of ultrasound for visualization of nerves and adjacent anatomy is especially advantageous in neonates due to the miniscule size of the target structures and the close proximity of adjacent structures. The ultrasound pictures of soft tissue structures are similar to those in adults and older children when corrected for size. Neonatal bone, however, is largely cartilaginous and the relative lack of ossification in neonates produces different ultrasound images of the bones. During performance of a sciatic block, the femoral head lacks the characteristic echogenicity of the bone and may be difficult to visualize. The lack of bone density of the vertebrae also creates some acoustic windows that are not normally present in older infants and children, providing unique opportunities for access to the neuraxis. During real-time imaging for a paravertebral block, the structures of the entire neuraxis may be visible due to lack of ossification in the overlying vertebra. These differences in imaging must be considered when searching for appropriate landmarks.
The integrity of the coagulation system is important when performing perineural procedures [5]. Differences exist in the neonatal coagulation system for both premature and term infants when compared to adults and older children, and result in different “normal” values when considering laboratory studies of coagulation function. Fortunately, any maturational differences are considered to be functionally balanced and do not affect overall hemostasis and thrombosis. It is, therefore, important to use normal values for gestation and age when making decisions about the performance of regional anesthesia.
The risk of local anesthetic systemic toxicity in neonates is higher compared to older children and adults for a variety of reasons. Local anesthetics are highly bound to plasma proteins, the most important of which is alpha1-acid glycoprotein. Infants have lower blood levels of alpha 1-acid glycoprotein (AAG), about 50 percent of the adult value, which results in less protein binding of local anesthetics and greater free fraction of unbound drug. Fortunately, AAG is an acute phase reactant protein and levels can rise in response to stress, providing some protection to the stressed neonate [6]. In addition, amide local anesthetic drugs are metabolized in the liver and neonates have reduced metabolism and clearance due to immaturity of the cytochrome P450 enzymes. Consequently, bolus dosing of amide local anesthetic should be reduced in neonates and young infants by at least 30 percent [7]. Pharmacokinetic data suggest that serum levels of amide local anesthetics can continue to rise with continuous infusions in neonates, even at low dosing [8]. Local anesthetic dosing recommendations are provisional and based on extrapolation of data in older children, infant animal studies, and limited pharmacokinetic data. All bolus doses should be administered incrementally with careful monitoring for toxic effects.
Ester local anesthetics are metabolized by plasma pseudocholinesterase, and although neonates have reduced levels of pseudocholinesterase, elimination of the most commonly used esters is very rapid and complete. Due to concerns about increased risk of amide local anesthetic accumulation and toxicity in neonates and young infants, infusions of 2-chloroprocaine have been advocated for use in neonates and young infants, and appear to be safe [9].
Peripheral Nerve Blocks
Indications
Almost all of the blocks performed in older pediatric populations have been used in neonates (Table 36.1).
Table 36.1 Surgical sites and their peripheral nerve blocks
Supraorbital | Frontal craniotomy, VP shunt, forehead surgery |
Infraorbital | Upper lip surgery |
Mandibular division of trigeminal | Lower lip surgery, mandibular distraction |
Greater occipital | Posterior fossa craniotomy |
Infraclavicular | Peripherally inserted central catheter (PICC), upper extremity amputation, syndactyly, polydactyly |
Digital | Finger surgery |
Axillary | Syndactyly, polydactyly, upper extremity amputation, upper extremity ischemia |
Paravertebral, intercostal | Rib fx, TEF, esophageal atresia, abdominal surgery |
Transversus abdominis plane | Ileostomy/colostomy creation and takedown, laparotomy, closure gastroschesis or omphalocele, pyloromyotomy |
Ilioinguinal | Herniorrhaphy |
Rectus sheath | Laparoscopy, periumbilical incision |
Sciatic | Lower extremity amputation, syndactyly, polydactyly |
Femoral | Lower limb amputation, muscle biopsy |
Fascia iliaca | Muscle biopsy |
Penile/pudendal | Circumcision |
Techniques
Many sources describe the technical aspects of regional anesthesia for use in infants and children. A comprehensive review of all blocks is beyond the scope of this chapter, but descriptions of the most common peripheral blocks with clinical pearls specific to neonates are included.
Transversus abdominis plane (TAP)
The abdomen is innervated by the thoracoabdominal nerves that are the continuation of the corresponding thoracic spinal nerves. These nerves travel between the deep investing fascia of internal oblique (IO) and the transversus abdominis (TA) muscles. The TAP block in neonates is used for surgery of the abdominal wall and is usually performed with ultrasound guidance. The muscle layers of the abdominal wall assume a characteristic pattern of three parallel hyperechoic muscles separated by hyperechoic fascia. In adult studies, injection at the midaxillary line results in dermatomal spread inferior to the umbilicus and is suitable for surgeries below the umbilicus, while injection more posterior, at the termination of the TA muscle, results in more extensive cephalad spread, reported as high as T7 [10]. This block has been used successfully for major and minor operations of the abdominal wall with both single injections and placement of unilateral indwelling catheters. Bilateral TAP catheters in this age group are impractical due to limitations in safe local anesthetic dosing. The TAP block is feasible in patients for whom thoracic epidurals may be contraindicated or undesirable, but no direct comparative studies are available.
Equipment
-
(1) Needle/catheter:
-
(a) Single injection: 22–24G × 40–50 mm needle
-
(b) Catheter: 18G × 50 mm Tuohy with 20G catheter; alternatively, a 20G needle with an 18G catheter over the needle system.
-
-
(2) Portable ultrasound with a high-frequency (6–13/18 MHz) linear transducer with a limited footprint (25–38 mm).
Dosing
-
(1) Single bolus: 0.2–0.50 ml kg–1 of 0.2 percent ropivacaine, 0.25 percent bupivacaine (optimal dose/dermatomal spread is not yet determined).
Technique
The ultrasound transducer is placed in a transverse orientation at the midaxillary line between the iliac crest and the 12th rib. The characteristic landmarks are identified to include the external oblique, IO, TA muscles, and the transversalis fascia and parietal peritoneum.
-
(1) Lateral TAP: Injection at the level of midaxillary line between the iliac crest and the 12th rib will result in reliable coverage of T11–L1. The needle is advanced in-plane with continuous visualization until the tip penetrates the fascia below the IO muscle. A small amount of saline is injected to confirm placement. After careful aspiration, the local anesthetic is injected and viewed for appropriate spread with real-time ultrasound.
-
(2) Posterior TAP: If more cephalad dermatomal coverage is desired, after identification of landmarks in the midaxillary line, the transducer is moved posterior until the termination of the TA muscle is identified and injection placed in the fascia at the termination of the TA muscle. If desired, a catheter can be advanced into the space and secured.
Tips
-
(1) Saline should be used to identify the fascial plane to limit dose of local anesthetic.
-
(2) For bilateral single-shot injections, local anesthetic can be diluted to 0.1 percent.
-
(3) Fascial planes are difficult to distinguish in lower weight infants.
-
(4) Echogenic needles facilitate needle visibility.
-
(5) Complications are rare but the close proximity to the abdominal cavity may predispose to injury of the bowel or liver if the needle is advanced into the peritoneum.
Ilioinguinal/Iliohypogastric
The ilioinguinal/iliohypogastric nerves arise from L1 (and sometime T12) and provide the sensory innervation to the skin of the lower abdominal wall, upper thigh, and lateral hip, making this an excellent block for analgesia following inguinal hernia repair, a frequent operation in former preterm infants. The terminal portions of the ilioinguinal/iliohypogastric nerves travel between the TA and IO just medial to the anterior superior iliac spine (ASIS) and can be identified with both ultrasound and landmarks [11,12]. The use of portable ultrasound to identify the landmarks and nerves has resulted in more reliable analgesia with fewer reported complications, but if resources are limited a landmark technique is a good option [13]. Bowel perforation and hepatic laceration have been reported with the landmark technique, while intraperitoneal trespass of the needle has been reported in the ultrasound group without negative consequences.
Equipment
-
(1) Needle: 22–24G × 40–50 mm needle
-
(2) Portable ultrasound with a high-frequency (6–13/18 MHz) linear transducer with a limited footprint (25–38 mm).
Dosing
0.2–0.40 ml kg–1 of 0.2 percent ropivacaine or 0.25 percent bupivacaine
Technique
-
(1) Ultrasound-guided technique: The transducer is placed at the edge of the ASIS and oriented toward the umbilicus. The three characteristic muscular layers of the abdominal wall, external oblique (EO), IO, and TA are identified. At this level the EO may be an aponeurotic sheath leaving just the IO and TA identifiable. In the fascial plane between the IO and the TA, the ilioinguinal nerve, when visible, will be located approximately 2 mm and the iliohypogastric will be about 4 mm away from the ASIS. The nerves appear as ellipsoid in shape with a hyperechoic sheath and hypoechoic center. Advance the needle slowly in-plane to a point midway between the two nerves and, after careful aspiration, inject immediately below the fascia beneath the IO.
-
(2) Landmark technique: Palpate and identify the ipsilateral ASIS and mark a point 3–4 mm away from the ASIS in a line connecting the ASIS to the umbilicus [14]. Following aseptic technique, the block needle is inserted perpendicular to the skin and advanced slowly to allow the operator to appreciate two “pops” associated with fascial planes between the EO and the IO and the IO and the TA. The needle must be advanced carefully as the fascial “pops” are subtle in the neonate.
Paravertebral
The thoracic spinal nerves exit the intervertebral foramen into the paravertebral space en-route to becoming the intercostal nerves. A nerve block at this level provides excellent analgesia for unilateral surgery on the thorax or abdomen. The paravertebral space is a triangular-shaped space bounded by the parietal pleura, the costotransverse ligament, and the posterolateral aspect of the vertebral bodies. This space containing the nerves is continuous between thoracic levels and injection at one level provides coverage of adjacent nerves in a dose-dependent fashion. Studies indicate that 0.2 ml kg–1 covers 5–6 dermatomes and 0.4 ml kg–1 covers 6–8 dermatomes [15]. Single injections and unilateral catheters are both used. Bilateral catheters are used in larger patients, but the small size of neonates and the limits on safe dosing of local anesthetic makes bilateral catheters impractical. Pediatric studies indicate a high success rate and no major complications, although pneumothorax, subarachnoid block, and hypotension have been reported in adults. This block was first described in neonates using a landmark technique in 1992 and has since been described using an ultrasound-guided technique.
Equipment
-
(1) Needle/catheter:
-
(a) Single injection: 22–24G × 40–50 mm needle
-
(b) Catheter: 18G × 50 mm Tuohy with 20G catheter; alternatively, a 20G needle with an 18G catheter over the needle system.
-
-
(2) Portable ultrasound with a high-frequency (6–13/18 MHz) linear transducer with a limited footprint (25–38 mm)
Dosing
0.2–0.40 ml kg–1 of 0.2 percent ropivicaine or 0.25 percent bupivacaine; dermatomal spread is dose-dependent. See Chapter 35 for peripheral nerve catheter infusions.
Ultrasound-guided Technique
The appropriate thoracic level is identified and marked. Following aseptic technique, the transducer is placed in a transverse orientation over the spinous process of the desired thoracic level. The probe is then moved lateral until the transverse process is visualized as a thumb-like hyperechoic structure above a hyperechoic sliding shimmering line representing the parietal pleura. When visible, the internal intercostal membrane (IICM) will be seen as a hyperechoic structure connecting the bottom of the transverse process to the internal intercostal muscle. The needle is advanced in-plane in a lateral to medial direction until the tip of the needle penetrates the IICM and rests in the space between the pleura and the transverse process. Proper location is confirmed by downward displacement of the pleura with injection of 1 ml of sterile saline. If desired, a catheter can be advanced into the space and secured.
Tips
-
(1) Saline should be used to identify proper needle tip placement to limit dose of local anesthetic.
-
(2) The acoustic shadow characteristically seen below the transverse process on older patients will be absent due to the lack of ossification.
-
(3) For bilateral single-shot injections, local anesthetic can be diluted to 0.1 percent.
Rectus Sheath
The terminal branches of the T8–T11 thoracoabdominal nerves exit the TAP plane (from between the IO and the TA), pierce the posterolateral border of the rectus sheath (RS) and course through the rectus abdominus (RA) muscle. These branches innervate the center of the abdominal wall between the lateral edges of the RA muscle, including the umbilicus. This block provides excellent analgesia for midline surgeries including laparoscopies, pyloromyotomies, and midline laparotomy incisions.
Equipment
-
(1) Needle: 22–24G × 40–50 mm needle
-
(2) Portable ultrasound with a high-frequency (6–13/18 MHz) linear transducer with a limited footprint (25–38 mm)
Dose
0.1 ml kg–1 of 0.2 percent ropivacaine or 0.25 percent bupivacaine.
Technique
The dermatomal level to be blocked is identified and marked. (The umbilicus is the most common level to block.) Using aseptic technique, the transducer is placed in a transverse orientation with the lateral edge of the transducer lined up with the lateral edge of the RA muscle (the linea semilunaris). The RA muscle is identified and the probe is moved slightly lateral until the medial edges of the muscles of the lateral abdominal wall are just visible. Two hyperechoic lines beneath the RA muscle represent the transversalis fascia and the parietal peritoneum.
Using an in-plane technique, the needle is inserted slowly to reach a target at the lateral-most edge of the posterior RA at a point just above the transversalis fascia. Injection into this space will cause the RA muscle to “roll up” away from the transversalis fascia.
Tips
-
(1) The epigastric vessels run within the RA muscle and Doppler can be used for identification.
-
(2) Saline should be used to identify proper needle tip placement to limit dose of local anesthetic.
-
(3) For multiple single-shot injections, local anesthetic can be diluted to 0.1 percent.
-
(4) Echogenic needles facilitate needle visibility.
Dorsal Penile
Male circumcision is commonly performed in the neonatal period, and penile nerve block has been shown to decrease the associated pain and stress response [16,17]. The penis is innervated by the pudendal nerve (S2–3), which divides into the right and left dorsal nerves of the penis and travels in the subpubic space just below the scarpas fascia. The nerves are lateral to the dorsal veins and arteries. The traditional landmark technique is described.
Equipment
-
(1) 25–30G × ¾–1¼ needle.
Medication
Bupivacaine 0.25 percent; 1 ml with total dose not to exceed 1.5 mg kg–1. Since the penile block is a fixed-needle procedure, the risk of inadvertent intravascular injection is higher.
Technique
Following aseptic preparation of the base of the penis, the penis is retracted gently in a caudal direction. At a point just below the inferior ramus of the pubic bone, two separate injections are made at the 10 o’clock and 2 o’clock positions as measured from the midline [18]. The needle is advanced slowly until first a gentle “give” (superficial fascia) is felt followed by a more definitive “pop” of the deep fascia. After aspiration, 0.5 ml of local anesthetic is injected. Injection is repeated on the contralateral side.
Femoral Nerve Block
The femoral nerve is composed of branches of the L2–L4 spinal nerves and innervates the femur, the skin of the anterior aspect of the upper leg, and the knee joint. The nerve travels with the femoral artery and can be best accessed just caudal to the inguinal ligament. The fascia surrounding the iliopsoas muscle, the fascia iliaca, runs on top of the nerve and separates the nerve from the artery. This block can be used for lower limb salvage surgery and muscle biopsies. To avoid entering the hip joint, this block is best performed using ultrasound guidance.
Equipment
-
(1) Needle/catheter:
-
(a) Single injection: 22–24G × 40–50 mm needle
-
(b) Catheter: 18G × 50 mm Tuohy with 20G catheter; alternatively, a 20G needle with an 18G catheter over the needle system.
-
-
(2) Portable ultrasound with a high-frequency (6–13/18 MHz) linear transducer with a limited footprint (25–38 mm)
Dosing
0.1 ml kg–1 of 0.2 percent ropivacaine or 0.25 percent bupivacaine.
Technique
Using aseptic technique, the transducer is placed at the level of and parallel to the inguinal crease. The landmarks of this block include the femoral artery, the iliopsoas muscle, and the fascia iliaca. The nerve lies lateral to the artery and under the fascia iliaca, and it assumes a triangular or compressed oval shape. Using an in-plane approach, the needle is advanced slowly to a target position under the fascia iliaca and lateral to the nerve. Once the needle is through the fascia iliaca, an injection of saline can be used to confirm placement beneath the fascia iliaca. After careful aspiration, local anesthetic can be injected. If desired, a catheter can be inserted and secured.
Tips
-
(1) Manual compression of the vein with the ultrasound transducer confirms lateral to medial orientation.
-
(2) Saline should be used to identify needle tip placement to limit dose of local anesthetic.
-
(3) The needle tip must be under the fascia iliaca.
Infraclavicular Brachial Plexus
The brachial plexus is composed of the ventral rami of the fifth cervical through the first thoracic spinal nerves, and supplies all of the innervation to the upper extremity. The brachial plexus can be blocked at multiple locations along its course, but the most accessible site in small infants is the infraclavicular approach that accesses the nerves as cords of the brachial plexus after they pass under the clavicle. This block can be used to facilitate peripheral insertion of central catheters (PICC lines) without the need for general anesthesia, and can facilitate limb salvage [19].
Equipment
-
(1) Needle/catheter:
-
(a) Single injection: 22–24G × 40–50 mm needle
-
(b) Catheter: 18G × 50 mm Tuohy with 20G catheter; alternatively, a 20G needle with an 18G catheter over the needle system.
-
-
(2) Portable ultrasound with a high-frequency (6–13/18 MHz) linear transducer with a limited footprint (25–38 mm).
Dosing
0.1 ml kg–1 of 0.2 percent ropivicaine or 0.25 percent bupivacaine.
Technique
Using aseptic technique, the ultrasound transducer is placed in a cephalad to caudad orientation adjacent to the medial aspect of the corocoid process with the edge of the transducer at the inferior edge of the clavicle. Slide the probe as needed to visualize the axillary artery in short axis. The two muscles overlying the artery are the pectoralis major and minor. The brachial plexus at this level consists of three cords, with the medial cord located at 9 o’clock, the posterior cord at 6 o’clock, and the lateral cord at 3 o’clock relative to the artery. If individual cords cannot be identified it will suffice to surround the artery with local anesthetic. The block needle is advanced in-plane to the bottom of the artery, initially targeting the posterior cord. Additional passes of the needle are performed to surround all of the cords and the artery with local anesthetic. If desired, a catheter can be passed through the needle and secured.
Epidural Anesthesia
Epidural anesthesia continues to occupy a major role in pediatric regional anesthesia and represents almost half of the regional anesthetics in children less than three years of age [20]. In parallel with the use of ultrasound in all regional anesthesia, the use of ultrasound for guidance of epidural anesthesia has evolved quickly. When imaging and accessing the neuraxial structures in infants and children it is important to be aware of the developmental changes in the spine and the spinal canal that affect the ultrasound images.
The location of the spinal cord and dural sac within the spinal canal changes over time. During the development of the fetus in utero, the spinal cord extends the entire length of the spinal canal. This position ascends as the fetus develops and in a newborn the spinal cord extends as low as the L3 level, with termination of the dura sac at the S4 level [21]. As the child grows, the vertebral column grows faster than the spinal cord and the termination of the spinal cord moves to a progressively higher level; L1/L2 for spinal cord and S2 for dura at one year of age [22,23]. These may be important details to keep in mind in order to avoid complications such as inadvertent dural puncture and/or spinal cord trauma [23].
The limited ossification of the pediatric vertebral column allows better visualization of the neuraxis in pediatric patients. The newborn skeleton is predominantly cartilaginous at birth and proceeds to deposit calcium and ossify progressively until puberty. This relative lack of ossification allows the easy penetration of ultrasound waves and a clear view of the neuraxial structures up until three months of age, after which ultrasound imaging becomes progressively more difficult [24]. Around nine months of age the bony mass of the vertebral arches start to impose ultrasound shadows over the underlying structures, but anatomic information remains available in the ultrasound windows between adjacent vertebrae.
Ultrasound can be used in multiple ways to help with accessing the pediatric epidural space at different levels. Pre-puncture scanning gives information about the location and depth of the epidural space and adjacent structures. Alternatively, ultrasound imaging is used for real-time scanning to guide and direct the needle and catheter insertion into the epidural space. The ultrasound imaging is also used after the catheter or needle is inserted to confirm position.
Single-Shot Caudal Approach to the Epidural Space
Background and Indications. The relative popularity of epidural analgesia can be attributed to the relative ease with which the epidural space can be accessed via the sacral hiatus in infants and young children. A single injection of local anesthetic into the caudal epidural space, the “kiddie caudal,” is appropriate for most surgeries below the umbilicus and results in low complication rates and high success rates. Inadvertent spinal anesthesia, with an incidence of dural puncture of 0.19 percent [25], and perforation of adjacent viscera from improper position of the needle, have, however, been reported. Ultrasound guidance offers a way to identify sacrum anatomy and perform caudal blocks in the patients at risk for dural puncture (neonates and very small infants), and patients with difficult anatomy (older patients and patients with lumbosacral spinal dysraphism), potentially increasing the success rate and reducing the rate of complications [26–28].
Anatomy. The sacrum is composed of five fused sacral vertebrae (Figure 36.1). Its dorsal surface is convex and has a raised interrupted median crest with four (sometimes three) spinous tubercles representing fused sacral spines. The posterior surface is formed by fused laminae. Below the fourth (or third) spinous tubercle an arched sacral hiatus is identified due to failure of the fifth pair of laminae to meet, exposing the sacral canal, a continuation of the lumbar spinal canal. This caudal opening of the canal, or sacral hiatus, is covered by the sacrococcygeal ligament, which is an extension of the ligamentum flavum. The fifth inferior articulate processes project caudally and flank the sacral hiatus as prominent boney sacral cornua. The sacral canal contains the cauda equina (including the filum terminale) and the spinal meninges, epidural venous plexus, and adipose tissue. The lowest margin of the filum terminale emerges at the sacral hiatus and transverses the dorsal surface of the fifth sacral vertebra.
Figure 36.1 The sacrum structures are visualized in both the short axis (transverse) and the midline long axis (longitudinal).
Short axis. (See Figure 36.2.) The two bony prominences of the sacral cornua appear as hyperechoic reversed U-shaped structures (Figure 36.3). Two hyperechoic band-like structures lie between the two cornua. The band-like structure on top of the sacral cornua is the sacrococcygeal ligament and the band-like structure at the bottom is the bone of the dorsal surface of the anterior sacrum. The sacral hiatus is the hypoechoic region between the two band-like structures.
Figure 36.2 Ultrasound probe position for transverse scan over sacrum.
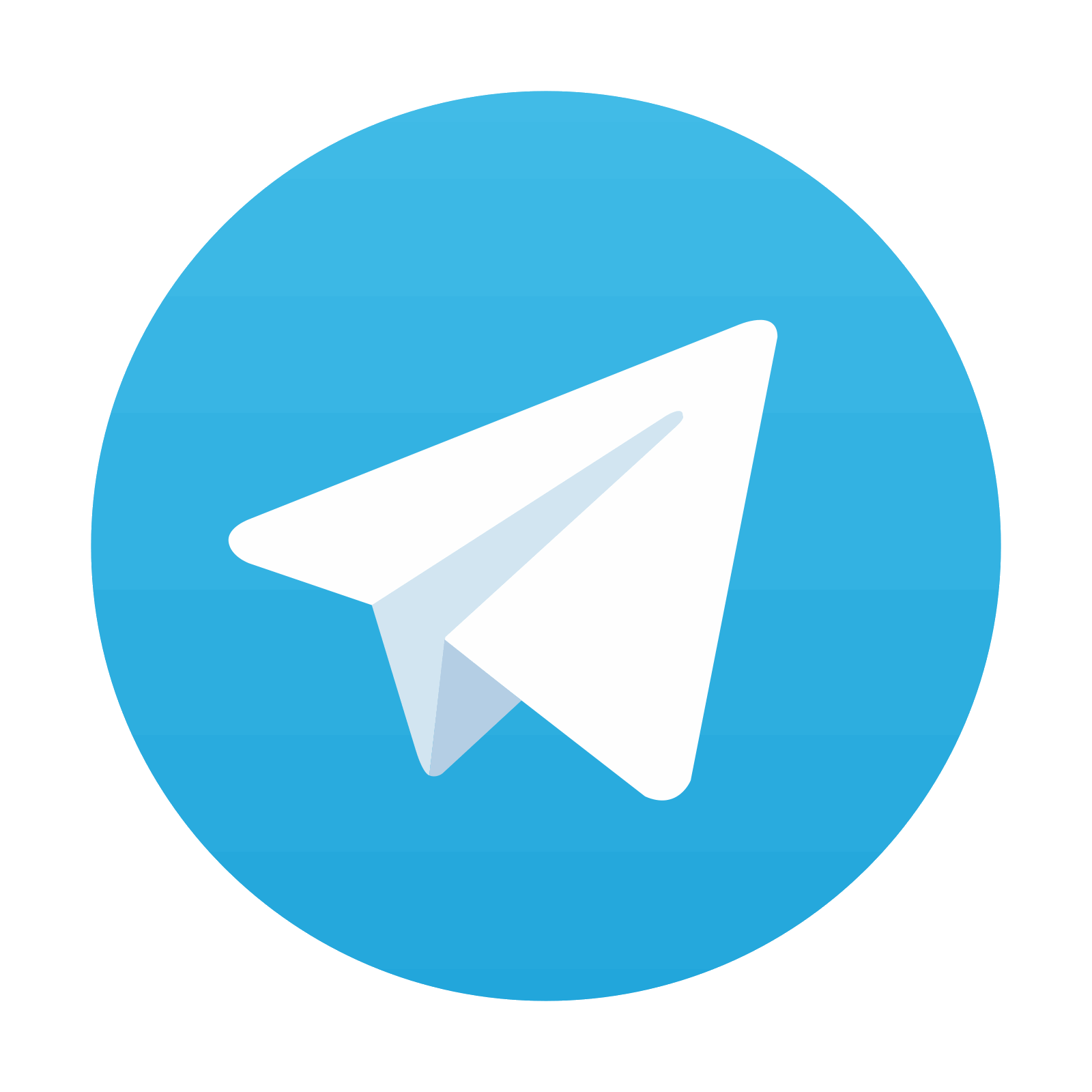
Stay updated, free articles. Join our Telegram channel
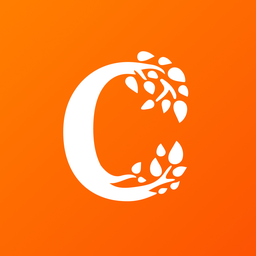
Full access? Get Clinical Tree
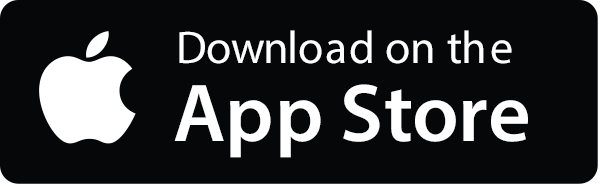
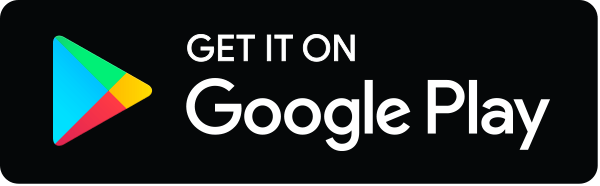