Preoperative Evaluation
The objective of the preoperative evaluation of the pediatric surgical patient is to assess current clinical status and alleviate fear and anxiety of the child and family. The process of anesthetizing an infant or child and the associated risks must be demystified during the preoperative visit since parents often have more anxiety about their child undergoing anesthesia than they do for themselves. The preoperative visit is an opportunity for the anesthesiologist to evaluate the child’s psychological status and family interactions. It has been demonstrated that parental anxiety surrounding anesthesia is highest when surgery is scheduled for infants less than one year of age and is the child’s first surgical experience [1].
Psychosocial Preparations of the Family
Anesthesiologists and other healthcare professionals who treat children face the unique challenge of caring for two patients at once. As a result of the child’s dependence, the child and parent become the patient–parent dyad. The psychological differences between children and adults are well known, and in recent years it has also been well documented that differences are seen in the emotional and cognitive development of the child as compared to that of an adult. Since reasoning skills have not yet matured in children, the understanding of and response to illness is affected. Communication skills are not highly developed either, therefore the medical practitioner is required to anticipate the child’s needs and concerns and be able to interpret nonverbal expressions and actions [2].
The anesthesiologist must offer advice, support, and reassurance on two levels and must not only be aware and sensitive to the child’s needs, but must also be able to support the parent’s need for information that will enable them to remain a strong advocate for their child throughout the medical system. For most parents, the prospect of their child’s impending hospitalization is more frightening than almost anything else. Perioperative anxiety is greatest for parents of infants between the ages of 0–6 months. Factors that influence parental anxiety include the age of the child, previous surgery in the patient or a sibling, parental gender, highest level of education obtained by the parent and preoperative discussion. Interestingly, these factors were more significant for mothers when parents were assessed by gender [3].
As infants begin to differentiate their primary caregivers from others, they develop a previously absent wariness of strangers. This change begins to occur at approximately eight months of age, whereas infants younger than this will usually separate easily from their primary caregiver. A discussion of the infant’s behavior and the presence of stranger anxiety should be included in the preoperative interview so that the anesthesiologist may obtain a sense of how easily the infant will separate from the parent and how anxious the parent is. Prior to the onset of stranger anxiety in an infant, parental presence is not required or advisable during the induction of anesthesia; therefore parents of young infants should not be invited into the operating room. An explanation of what will occur after the infant is taken from the parents is usually sufficient. If stranger anxiety is already present in an older infant, then a parent may be invited into the operating room until the child is unaware of his or her presence if the anesthesiologist feels it would be safe. An explanation of the induction process should occur, including what the parent will observe, the operating room configuration, and the personnel that will be present. They should also be instructed on when they will be asked to leave and how they will be escorted from the operating room to avoid any distress regarding leaving their child with the operating room team.
Premedication
Infants under one year of age have varying degrees of separation anxiety. Stranger anxiety develops slowly; it does not just appear suddenly. As cognitive skills in a child develop and improve, typically around 12 months of age, their stranger anxiety can become more intense. The nature of anxiety in children is dependent on many factors, including age, temperament, past experience, and family background. Parental presence, distraction, and premedication have all been used successfully, but no single strategy is effective for all children [4]. When the need for premedication arises, the standard agent of choice has been midazolam, which has been shown to be effective in infants not only for premedication but to decrease anxiety during interventional procedures performed without general anesthesia [5]. The recent introduction of dexmedetomidine as an intranasal as well as oral agent for premedication has provided an alternative choice [6]. The most recent meta-analysis demonstrated that dexmedetomidine premedication is superior to midazolam with respect to satisfactory separation from parents and mask acceptance, and has an additional clinical advantage of reducing the requirement for rescue analgesia during the postoperative period. There are, however, increased risks of decreased heart rate and blood pressure compared to midazolam when dexmedetomidine is used for premedication [7]. Ketamine has been a longstanding choice for premedication in older infants, but it should be noted that children premedicated with ketamine require close observation in a quiet environment where resuscitation equipment is readily available. Studies have shown midazolam to be a more effective and safer premedication than ketamine [8].
Informed Consent About Short- and Long-Term Morbidity
The process of informed consent for anesthesia is carried out during the preoperative discussion. An assessment of risk that is specific to each patient with regard to the planned procedure and each patient’s current state of health as compared to the usual state of health should be discussed. The overall incidence of cardiac arrest during anesthesia in children under 18 years of age has been reported to be 2.9–4.95/10 000 and 18.28/10 000 below one year of age, making cardiac arrest in infants 3–6 times more common than in older children. The greatest risk occurs in patients with an American Society of Anesthesiologists risk stratification of III and IV, as well as those undergoing emergency surgery [9].
The most common surgical procedures associated with highest risk of cardiac arrest in children include airway procedures, followed by abdominal, thoracic, and cardiovascular procedures. The vast majority of neonatal cardiac arrest occurs in patients who have congenital heart disease [10]. The most frequent noncardiac causes of anesthesia-related cardiac arrests are medication and airway related [11,12]. Any medical comorbidities that exist which increase anesthetic risk should be described in detail and the measures that the anesthesia team will take to safely care for patients with regard to these risks should be explained. An explanation of the monitoring equipment in place in each operating room and the specific physiologic parameter that it measures is useful information to parents. In addition, a description of the number of personnel in the operating room as well as those available in case of an emergency is a reassuring piece of information for parents.
Ambulatory Surgery
The greatest risk for infants undergoing ambulatory surgery is post-anesthetic apnea. There is controversy regarding the lowest age at which both full-term babies and former premature babies may be discharged home after surgery. Former preterm infants born prior to 37 weeks’ gestational age as well as full-term infants less than 28 days of life are at increased risk for respiratory depression and apnea after general anesthesia. It is therefore recommended that the period of postoperative respiratory monitoring be extended for these patients. In former preterm infants without additional comorbidities, six hours of post-anesthesia respiratory monitoring is satisfactory since the first apneic episode usually occurs within the first four hours postoperatively [13]. In the presence of additional risk factors, including chronic lung disease, anemia (as defined by hematocrit <30 percent), recurrent apnea at home, or neurologic disease, the period of observation should be extended to 12 hours [14,15]. Whenever possible, elective surgery should be postponed until a postconceptual age of 60 weeks has been achieved.
Within the pediatric population, perioperative cardiac arrest under general anesthesia is more frequent in infants than in older children and beyond the first year of life age does not noticeably affect incidence of cardiac arrest [16,17]. Most cardiac arrests in the operating room are not a direct result of the anesthetic, and intraoperative cardiac arrests occur more frequently in children with congenital cardiac disease. Although there are no data to support discouraging elective ambulatory anesthesia in children less than one year of age, many institutions do not discharge children to home after general anesthesia until they are older than six months of age.
Smoking in the House
Exposure to environmental tobacco smoke is associated with detrimental effects on pulmonary function in infants and children. There is a strong association between passive inhalation of tobacco smoke and airway complications in children receiving general anesthesia. Interestingly, studies indicate that this relationship is greatest for girls and for those whose mothers have a lower level of education. Significant physiologic effects may be documented as a result of passive tobacco smoke exposure. Cotinine is an alkaloid found in tobacco and is also a measurable metabolite of nicotine. The level of cotinine in the blood is proportionate to the amount of exposure to tobacco smoke, thus it is a valuable indicator of tobacco smoke exposure, including secondary (passive) smoke. In recent reports, perioperative airway complications occurred in 42 percent of patients with urinary concentrations of cotinine >40 ng ml–1, in 33 percent of patients with concentrations of cotinine between 10.0 and 39.9 ng ml–1, and in 24 percent of patients with concentrations of cotinine <10 ng ml–1 (the three groups) [18]. The presence of a smoking caregiver is a significant independent risk factor for upper respiratory infection during the first three years of life. An increased risk of lower respiratory infections of two-fold or more occurs in infants and children from four months of age to three years. The risk of wheezing as a result of lower respiratory infections in the presence of a smoking caregiver was more than three-fold in this patient age group [19]. The exposure of infants to passive tobacco smoke should be investigated and, if present, minimized to decrease the risk of adverse respiratory events during the perioperative period.
Fasting Requirements
It is no longer advisable or safe to restrict children to “NPO after midnight” [20]. This severe restriction routinely increases each child’s chance of undergoing the induction of anesthesia when dehydrated, hypoglycemic, and irritable, all of which lead to increased risk under anesthesia. The risk of pulmonary aspiration of gastric contents in healthy children undergoing elective surgery is only 0.04 percent [21]. The American Society of Anesthesiologists (ASA) has proposed practice guidelines that may be followed when determining the NPO restrictions in children [22]. The ASA and others recommend fasting from clear fluids for two hours prior to anesthesia. Clear liquids consist of water, non-particulate juices (apple, white grape, etc.), Pedialyte®, and Popsicles®. Fasting from breast milk for four hours and formula for six hours is recommended. The composition of human milk varies among mothers and is dependent on the mother’s diet, but in general is composed of 50 percent lipids, 40 percent carbohydrate in the form of lactose, and 10 percent protein divided into casein and whey [23]. Breast milk may cause significant pulmonary injury if aspirated due to a high fat content as determined by the maternal diet. The suggested fasting period for solid food is six hours for regular meals and eight hours for fat-containing meals; however, a large survey of pediatric institutions recommends fasting from all solids for at least eight hours in all children [24]. It is best to check with each surgical facility for specific practice guidelines.
Immunization
There is no direct evidence of any major interaction between immunization and commonly used anesthetic agents and techniques in children, but it is possible that immunosuppression caused by anesthesia and surgery may lead to decreased vaccine effectiveness. In addition, diagnostic difficulty may arise if a recently immunized child suffers from postoperative temperature elevation or malaise [25]. From a risk management perspective, a review of the available evidence suggests that it would be prudent to adopt a cautious approach where the timing of elective surgery is discretionary. It is therefore recommended that elective surgery and anesthesia should be postponed for one week after inactive vaccination and three weeks after live attenuated vaccination in children.
Anesthesia and surgery exert immunomodulatory effects and some authors argue that they may exert additive or synergistic influences on vaccine efficacy and safety. Alternatively, inflammatory responses and fever elicited by vaccines may interfere with the postoperative course. There is a lack of consensus approach among anesthesiologists regarding the theoretical risk of anesthesia and vaccination. The immunomodulatory influence of anesthesia during elective surgery is both minor and transient (around 48 hours) and the current evidence does not provide any contraindication to the immunization of healthy children scheduled for elective surgery. Respecting a minimal delay of two days for inactivated vaccines or 14–21 days for live attenuated viral vaccines between immunization and anesthesia may be useful to avoid the risk of misinterpretation of vaccine-driven adverse events as postoperative complications [26].
Prematurity
Preterm births as defined by completion of 37 weeks’ gestation or 260 postmenstrual days are estimated to occur in 12.7 percent of live births [27,28]. This group of infants has a unique subset of perioperative comorbidities that the anesthesiologist should be alert to and investigate preoperatively to avoid any unexpected untoward events related to the anesthesia or perioperative episode of care.
Thorough preoperative assessment of the former preterm infant is essential and allows time for optimization of medical conditions. These infants present for a variety of surgical procedures and some, like hernia repair, may seem minor, but the anesthetic management remains challenging. Two major areas of concern for professionals and family alike are the extent of chronic lung disease and the possibility for postoperative apnea. Concise delineation of other coexisting conditions is also extremely important to anesthesiologists.
Infants born prematurely may range from having no residual lung disease to significant bronchopulmonary dysplasia (BPD). The latter condition is extremely variable in its manifestations from mild radiographic changes in an asymptomatic patient to pulmonary fibrosis, emphysema, reactive airway disease, chronic hypoxemia and hypercarbia, tracheomalacia or bronchomalacia, and increased pulmonary vascular resistance with cor pulmonale. If pulmonary hypertension and cor pulmonale are suspected, a pre-anesthetic echocardiogram is useful not only to confirm the diagnosis, but also to guide optimization of medical therapy. Diuretics, bronchodilators, and corticosteroids are medications that many of these patients require to optimize the child’s respiratory function, and should be continued up to and including the day of surgery. Measurement of serum electrolytes to evaluate the degree of hypokalemia and compensatory metabolic alkalosis may be valuable, especially if therapy has recently been altered. Consideration of pharmacological “stress” doses of steroids should be considered in infants receiving steroid treatment.
Postoperative apnea has been reported following anesthesia in former preterm and term infants alike. The incidence of post-anesthetic apnea is inversely related to the postconceptual age and gestational age. Infants younger than 56 weeks postconceptual are at greatest risk since the risk of post-anesthetic apnea does not fall to less than 1 percent until 55 weeks postconceptual age. This risk is present in full-term infants under 28 days of life. The concurrent presence of anemia is a confounding factor that further increases the risk of post-anesthetic apnea. Hematocrit should be targeted at a minimum of 30 percent in this patient population [29–31]. There is no consensus about the patient profile of at-risk infants. Reports are not consistent in identifying the postconceptional age or gestational age of at-risk patients, the methods used to detect apnea or periodic breathing, the surgical procedure, other confounding medical conditions, or even the definition of apnea [14].
A combined analysis of eight prospective studies investigated postoperative apnea in former preterm infants following inguinal herniorrhaphy. A uniform definition of apnea was cessation of breathing or no detection of air flow for ≥15 seconds or ≤15 seconds with bradycardia defined as heart rate <80 bpm. Examination of the data revealed that hematocrit <30 percent, apnea at home, postconceptional age, and gestational age are all important risk factors for postoperative apnea [32]. This supports claims that the risk of postoperative apnea is inversely related to postconceptional age and that infants as old as 60 weeks postconceptional age may be susceptible. Also, some authors suggest that infants with a prior history of apnea and bradycardia, respiratory distress, intubation, and mechanical ventilation may be at further increased risk [33–35]. Any child considered to be at risk for postoperative apnea should have arrangements made for overnight observation and monitoring. The former preterm infant should also have a recent hematocrit available since a value of <30 percent is associated with an increased incidence of post-anesthesia apnea irrespective of postconceptional age [36,37].
Intraventricular hemorrhage is a frequent finding in premature infants and is categorized as Grade 1–4 depending on the extent of hemorrhage beyond the germinal matrix, into the ventricular system, and finally penetration into the brain parenchyma. Grade 1 hemorrhage will usually not result in significant concern for the anesthesiologist, however more extensive blood pressure control is warranted for higher grade hemorrhage.
Retinopathy of prematurity is inversely related to gestational age. It occurs in up to 50 percent of premature infants [38,39]. The presence of retinopathy of prematurity should be noted in the preoperative review since the goal of the anesthetic plan should be to maintain oxygen saturation between 90 and 95 percent with the minimal concentration inspired oxygen [40].
The incidence of hypoglycemia, defined as less than 45 mg dl–1, is three-fold higher in preterm infants as a result of decreased glycogen stores and feeding challenges. It is essential that this be identified so that a perioperative plan for glucose supplementation be made. Sepsis as a result of perinatal or postnatal acquired organisms is not an uncommon finding in the premature infant. A treatment plan for the infection as well as for hemodynamic support if the surgical procedure cannot be postponed should be identified. Because of their relatively smaller size, preterm infants are susceptible to periods of hypothermia and cold stress. Physiologic immaturity of thermoregulation, the lack of brown adipose tissue, and the lack of non-shivering thermogenesis predispose to the inability to generate heat in the same way that full-term and older infants do. A perioperative plan for temperature regulation and heat conservation is essential during the preoperative period.
Review of Systems
Cardiovascular
Effective cardiorespiratory function is represented by a respiratory rate under 60 breaths/minute, oxygen saturation above 95 percent by the first several hours of life, and the absence of respiratory distress, nasal flaring, grunting, or chest wall retractions [41]. When confronted with a neonate with respiratory abnormalities, important diagnostic considerations include: complex structural cardiac lesions, diaphragmatic hernia, persistent pulmonary hypertension, meconium aspiration syndrome, especially in infants greater than 40 weeks’ gestational age, spontaneous pneumothorax, transient tachypnea of the newborn, or pneumonia [42,43].
The etiology of a new murmur or abnormality in the cardiovascular system must be fully investigated prior to the planning and induction of general anesthesia. The timing, location, intensity, radiation, quality, and pitch are characteristics of heart murmurs that can distinguish physiologic from pathologic murmurs. Physiologic murmurs detected during the first 48 hours of life are usually attributed to flow across the ductus arteriosis as it closes or across the pulmonic valve as pulmonary vascular resistance changes. These murmurs are transient, soft ejection murmurs, usually Grade 1 or 2. Pathologic murmurs detected in the first few hours of life are caused by outflow tract obstruction as occurs in aortic or pulmonic stenosis, as well as subaortic stenosis associated with hypertrophic cardiomyopathy. These Grade 2 or 3 murmurs are characteristically crescendo–decrescendo in nature. Defects that produce left-to-right shunting appear within the first few days of life when the pulmonary vascular resistance has dropped. Pan-systolic murmurs early after birth are most commonly caused by AV value insufficiency. Shunting through a ventricular septal defect produces a pan-systolic murmur that appears after several days of life. A continuous murmur heard in an infant most often represents an extra thoracic arteriovenous communication. Referral to a cardiologist should be made during the preoperative assessment if cardiac disease is suspected.
Respiratory
The child with a recent upper respiratory infection (URI) poses a clinical dilemma for the anesthesiologist. Because most young children can have up to six URIs per year, this is a common problem for which no absolute rules exist. Most children have clear breath sounds during quiet respirations. Cough is a sign of lower respiratory involvement and should be evaluated for origin (upper or lower airway) and quality (wet or dry). Several potential risks are encountered in the perioperative period in the child who has an active cold or is recovering from a recent one.
Adverse perioperative events occur more frequently in infants with URIs. These include atelectasis, oxygen desaturation, bronchospasm, croup, and laryngospasm [44,45]. Decisions to cancel or postpone surgery should be made in conjunction with the surgeon and should be based on the type of procedure, the urgency of the procedure, and the child’s overall medical condition. Bronchial hyper-reactivity may exist for up to seven weeks after the resolution of URI symptoms, and delaying surgery for this length of time is often impractical. Most practitioners would agree that surgery may be scheduled after the acute symptoms have resolved and no sooner than two weeks after the initial evaluation.
Infants with an anterior mediastinal mass are at significant risk for airway compromise during sedation due to compression of the intrathoracic larynx. Although lymphomas constitute the largest group of masses that arise in the anterior mediastinum in infants and children, other masses that may present in this location include teratomas, cystic hygromas, thymomas, hemangiomas, sarcomas, desmoid tumors, pericardial cysts, and diaphragmatic hernias of the Morgagni type. Patients with anterior mediastinal masses may present with varied signs and symptoms referable to both the cardiovascular and respiratory systems and are directly related to the location and size of the mass, as well as the degree of compression of surrounding structures. The most commonly observed respiratory symptom is cough, especially in the supine position, which results from anterior compression of the trachea. Infants less than two years of age are more likely to experience wheezing as a sign of tracheal compression. Other respiratory symptoms include tachypnea, dyspnea, stridor, retractions, decreased breath sounds, and cyanosis on crying, all of which should alert the practitioner to some degree of airway compromise that may worsen when positive intrathoracic pressure is generated. Preoperative computerized axial tomography may be beneficial in determining the degree of compromise and anesthetic plan.
Choanal atresia may be found as part of a constellation of congenital abnormalities, including those associated with CHARGE syndrome: coloboma, heart disease, atresia choanae, retarded growth, genital abnormalities, and ear abnormalities. Infants with choanal atresia are unable to change to oral breathing during periods of nasal obstruction, which may result in cyanosis at rest, which resolves with crying or placement of an oral airway. The incidence is approximately 1 in 8000 births [46]. Respiratory distress during the newborn period may be a result of obstruction at the level of the larynx – maybe due to laryngeal web, subglottic stenosis, or vascular ring. This should be clarified prior to induction of general anesthesia to avoid airway complications.
Infants born with congenital diaphragmatic hernia may present with severe respiratory distress as a result of lung hypoplasia and inadequate pulmonary gas exchange. Tachycardia, tachypnea, and cyanosis along with a scaphoid abdomen should suggest this diagnosis, which may be confirmed by radiographic evidence of a mediastinal shift and bowel loops in the chest.
Gastric
Congenital obstruction of the gastrointestinal tract may result in vomiting, abdominal distention, aspiration with or without pneumonia, dehydration, hypovolemia, and electrolyte abnormalities. Correction of these abnormalities during the preoperative period is essential for safe preparation for general anesthesia. Associated anomalies of other organs are found in more than 50 percent of cases of children with gastroesophageal abnormalities. Often there is a combination of vertebral, anal, cardiac, tracheal, esophageal, renal, and limb anomalies (VACTERL). Cardiac anomalies are found in 15–40 percent of cases. Esophageal malformations can occur in several forms: tracheoesophageal fistula, isolated esophageal atresia with or without tracheal communication, or double fistulae. The incidence is estimated at 1 in 4000 live births. Prenatal diagnosis can be made with ultrasonography. Signs noted soon after birth include drooling due to excessive secretions, regurgitation with subsequent aspiration, choking spells, abdominal distention, and inability to pass a gastric tube beyond the atretic esophagus. Contrast radiographic studies are often conclusive. The clinical course can be complicated by the consequences of prematurity, pulmonary aspiration, and abdominal distention.
Neurologic
The incidence of myelomeningocele is 1/1000 live births and although 75 percent of lesions occur in the lumbosacral region, affected children may present with a defect anywhere along the neuraxis. Dysfunction of the skeletal system, skin, genitourinary tract, and peripheral and central nervous system may also be present, so these organ systems should be fully evaluated during the preoperative visit. These children are frequent visitors to the operating room and need careful attention to their perioperative needs to avoid trauma that would make future encounters more difficult. There is high incidence of sensitivity to latex-containing products, so an attempt to limit exposure to latex should be made. This caution should be noted during the preoperative visit and “Latex Sensitivity” should be posted clearly on the front of the patient chart.
Seizures are a frequently encountered component of many early childhood illnesses and occur in 4–6/1000 children. Seizures are a symptom of an underlying central nervous system disorder that must be fully investigated and understood. The history should provide a detailed description of the seizure, including the type, frequency, and severity of symptoms, as well as the characteristics of the postictal state so that it may easily be recognized by the OR team should it occur during the perioperative period. All anticonvulsant therapy should be recorded and serum drug levels should be checked. All anticonvulsants should be taken up to and including the morning of surgery. If the infant has seizures despite adequate therapy, this should be noted.
Infants with significant trauma as well as infants who have a variety of congenital abnormalities are at risk for cervical spine instability. Infants younger than three months are unable to adequately support their head and are at heightened risk for cervical cord injuries, which can result from excessive forces during delivery or in the first few months of life. Altered mucopolysaccharide metabolism may predispose children to deformities of the odontoid process, resulting in cervical spine instability. Atlantoaxial instability and superior migration of the odontoid process may occur in children with rheumatoid arthritis and skeletal dysplasia. Children with trisomy 21 have laxity of the transverse ligament and abnormal development of the odontoid process, which results in cervical spine instability in 15 percent of cases. Symptoms include clinical manifestations of cord compression which usually are not manifested until after five years of age. The American Academy of Pediatrics’ guidelines suggest that parents be aware of the importance of cervical spine-positioning precautions to avoid excessive extension or flexion to protect the cervical spine during any anesthetic, surgical, or radiographic procedure. In infants in which cervical abnormalities are noted, intubation of the trachea should be undertaken in a neutral head position or with somatosensory evoked potential (SSEP) monitoring of the upper extremities [47].
Physical Examination
A full physical examination of the neonate and infant is an essential component of the preoperative review. As assessment of gestation age as well as chronologic age should be made. The examination should begin with an observation of the infant in an undisturbed state, since a great deal may be learned about relevant physical findings without touching the child. The entire skin surface should be inspected for temperature, moisture, elasticity, and fragility. The color of the skin and the presence of pallor, cyanosis, rash, jaundice, unusual markings, birthmarks, and scars from previous operations should be noted. When examining a newborn, the head should be examined by inspection and palpation to determine if there is any bruising as a result of delivery, as well as the fullness of the fontanels to determine alterations in intracranial pressure. Abnormal facies might be an indication of a syndrome or constellation of congenital abnormalities. One congenital anomaly is often associated with others.
Inspection of the oral cavity may reveal normal variants such as small cysts, Epstein’s pearls, Bohn’s nodules, and dental lamina cysts. These are of no consequence and should not cause difficulty with laryngoscopy or endotracheal intubation. A gloved finger may be inserted into the oral cavity to determine the shape and integrity as well as to identify any abnormalities such as cleft palate. The neck should be observed for range of motion to detect spine abnormalities such as Klippel–Feil syndrome, congenital torticollis, and any anterior midline abnormalities such as branchial cleft or thyroglossal duct cyst. Short or webbed neck may be an indication of chromosomal abnormalities such as Down or Turner syndrome.
The shape of the chest and mechanics of respiration should be observed even before the lungs are auscultated. A normal infant is observed to be centrally acyanotic, resting comfortably, breathing easily with clear breath sounds when the lungs are auscultated. The color, viscosity, and quantity of nasal discharge should be documented. Respiratory rate may be irregular and pauses up to 20 seconds are normal in the infant. Common causes of mild respiratory distress in the newborn include transient tachypnea of the newborn due to retained fetal lung fluid, spontaneous pneumothorax, neonatal sepsis, pneumonia, meconium aspiration, or congenital heart disease. The respiratory system should be evaluated by noting the rate and quality of respirations, the presence of noisy breathing, coughing, purulent nasal discharge, stridor, and wheezing. Retractions, nasal flaring, grunting, and paradoxical respiration may be signs of increased work of breathing and respiratory distress in the infant. Noisy or labored breathing may indicate nasal or upper respiratory obstruction. If the child is coughing, the origin of the cough (upper versus lower airway) and the quality (dry or wet) can be evaluated even before auscultation of the lungs. Signs of an acute upper respiratory infection should be documented if present. The ease of mouth opening should be determined and the airway should be evaluated for ease of intubation. If the child will not open his or her mouth, a manual estimation of the thyrohyoid distance should be made. Children with micrognathia, as in Pierre Robin syndrome or Goldenhar’s syndrome, may be especially difficult to intubate.
The goal of the cardiovascular examination in the infant is two-fold; to assess the status of the circulatory system and to detect congenital heart disease. The rate, rhythm, volume, and character of the pulses in all four extremities should be evaluated. The resting heart rate of the healthy newborn averages 120–130 bpm. The precordium is examined by inspection, palpation, and auscultation. Clicks, murmurs, or other abnormal heart sounds should be sought. If a heart murmur is detected on the cardiovascular examination, there are specific concerns that must be addressed. An innocent murmur may be due to turbulent blood flow, whereas a pathological murmur is usually due to a structural abnormality; this distinction must be made. Lesions in which bacterial endocarditis prophylaxis or protection from paradoxical air embolism are required must be documented so that the anesthesia team is made aware. The child with a heart murmur or a history of a murmur warrants special consideration. The determination of an innocent versus pathologic murmur as well as the presence of hemodynamic compromise should be made. Innocent or non-pathologic heart murmurs can be identified by four characteristics: the murmur is early systolic to mid-systolic; it is softer than grade 3 of 4; the pitch is low to medium; and the sound has a musical, not harsh, quality. The infant should be evaluated for risk of paradoxical air embolus and evaluated for the need for prophylaxis for subacute bacterial endocarditis [48].
The abdomen should be observed for configuration, fullness, and movement with respiration. Major abdominal wall abnormalities such as gastroschisis, omphalocele, prune belly, or bladder extrophy will be obvious on inspection. A scaphoid abdomen may be an indication of abdominal contents that are displaced into the chest, as occurs in diaphragmatic hernia. A distended abdomen may be a sign of intestinal obstruction, ascites, or an abdominal mass. Palpation of the abdomen should include examination of the liver, and bowel sounds should be present on auscultation. Risk for aspiration of gastric contents should be determined.
The physical examination should include an evaluation of the level of consciousness, ability to swallow, intactness of the gag reflex, and an adequate cervical spine range of motion, hypotonia, spasticity, or flaccidity. General muscle tone and the presence of signs of an increase in intracranial pressure should also be noted.
Laboratory Testing
It is important to remember that phlebotomy is often traumatic for infants and their families, and an event that is not easily forgotten. For this reason, it is best to limit the number of invasive tests performed. The diagnostic studies should be selected based on the general medical health and the procedure being performed. In general, measurement of hematocrit in a healthy older infant undergoing elective surgery is unnecessary [49]. A hematocrit should be measured if significant blood loss is anticipated, if the child is less than six months of age or was born prematurely. Neither the routine measurement of the coagulation profile nor a history of “easy bruising” is reliable in predicting surgical bleeding [50]. The presence of prior hematoma, bleeding from circumcision or large bruises should prompt an investigation; however, a negative history for bruising in an otherwise healthy child would require no further testing. Routine preoperative urinalysis is not indicated in children, and serum chemistries should only be performed when an abnormality is suspected. Infants who are treated with anticonvulsants should have these medication levels checked and an electrocardiogram or chest radiograph should only be ordered if the general medical condition warrants.
References
Neonatal and Pediatric Pharmacology: The Evolution of Scaled Modeling
In 1950, Terry et al. boldly stated what practicing pediatricians had known clinically for so long – neither age nor weight were satisfactory metrics for drug calculations in children. They therefore proposed scaling drug dosages according to body surface area, and were able to support this hypothesis with empirical evidence [1]. This scaling calculation was extended to water, electrolytes, and calories, preceding Holliday and Segar’s guidance for parenteral fluid therapy according to basal metabolic rate by seven years [2]. Allometric (nonlinear, growth-based) scaling entered the mainstream of dosing concepts at that time, but was embraced by relatively few, and rather simplistic pharmacology models persisted for decades (Figure 13.1).
Figure 13.1 A simplistic – and common – concept map of dosing and elimination.
Pediatric pharmacokinetic parameters are now understood with increasing precision. Age-adjusted volume of distribution is important for the loading dose and of crucial importance in anesthetic practice because of the need to rapidly achieve a target effect. Clearance determines the maintenance dose rate. Together, clearance and volume of distribution determine the shape of the concentration–time curve [3]. Pharmacodynamic parameters, particularly for children and more so for neonates, are a far different story. Almost all neonatal organ systems are still developing; some enzymes are present, some are absent, some are increasing in activity, some are decreasing. Hematocrit affects drug delivery, as does the concentration of proteins (and the types of proteins) in the blood. Many organ systems have a relatively larger mass within a neonates body – does that mean they function as hypercellular systems, or are those systems less mature despite the increase in mass? Effective drug delivery to the receptor/effector sites depends on blood flow, tissue volume, tissue solubility coefficients, and protein binding. Babies are different in almost all of these respects than children and adults.
Effective Modeling: Why are Children Different?
Volume of Distribution
Because a child’s job is growth and development, he or she is obligated to a ravenous early life of oxygen consumption, acquisition of calories, and production of heat. In order to survive as homeotherms – without burning up – they must have a way to exquisitely balance heat generation and heat loss, which led to the proposal of the Surface Law (homeotherms maintain their temperature homeostasis in proportion to their body surface area, i.e., heat production = heat loss) [4]. This turned out to be almost right. When oxygen consumption is measured directly or indirectly, it is actually more proportional to body mass (kg)3/4 (Figure 13.2), whereas body surface area is calculated as (kg)2/3 [5]. It turns out that thermal homeostasis is proportional to the vascular branching necessary to deliver heat to the skin surface, rather than the skin surface itself. The capacitance of this branched system, influenced by the scaling concept, formed the basis for Holliday and Segar’s fluid recommendations [2].
Figure 13.2 A log-linear plot of basal metabolism of mature animals of different species, with an almost uniform slope of 0.75 – the ¾ power, if expressed exponentially. The slope of the line is the exponent of the function. When the exponent = 1, there is isometry (isometric growth), meaning there is no change in shape during growth.
Accurate anticipation of differences in the volume of distribution is of crucial importance in anesthesiology in order to rapidly achieve a target effect. With a naturally larger volume of distribution because of allometric scaling as well as a larger “effective” volume of distribution because of a decrease in plasma proteins, a larger (apparent) volume of distribution is the result. This may explain the rather ironic requirement for a larger initial drug dose in infants and small children in order to achieve target concentration parity with adults and even older children (Figure 13.3).
Figure 13.3 Enhanced clearance and a larger central compartment volume results in reduced blood concentrations compared with adults. Smaller children require higher infusion rates (weight-based) than larger children or adults to achieve equivalent blood concentrations.
Clearance
Scalar “per kilogram” models of clearance under-predict, while surface area models over-predict clearance, leading to errors in calculating clearance with decreasing weight. Scalar modeling, for example, will lead to the false conclusion that children possess an enhanced capacity to metabolize drugs due to proportionally larger livers and kidneys (more “cellularity” per kilogram of body weight) than in adults [7]. As a better alternative, allometry is a good method of scaling clearance when only size differences exist [3]; however, it will overestimate clearance in children when metabolic clearance pathways are developmentally immature (e.g., activity of the enzyme per unit volume of tissue is lower). This method should only be used alone when the patient’s clearance for age reaches similar activity (>2 years) to that of adults [8]. A maturation model is required to describe clearance in neonates and infants (<2 years) to account for changes independent of size.
Cardiac Output
One consequence of the allometrically scaled increased cardiac output and organ size in infants (i.e., the brain receives 30 percent compared to 15 percent of this proportion-adjusted cardiac output) is that more drug reaches target receptors more rapidly in vessel-rich organ groups (brain, kidney, intestinal viscera, especially the liver).
Renal Clearance
Excretion may be reduced compared with older children and adults because glomerular and tubular renal function are reduced in newborns. Using an allometrically scaled postmenstrual age (gestational age + chronological age = PMA) model, Rhodin et al. reported adult GFR as 121.2 ml min–1 per 70 kg, with half of the adult value reached at 47.7 postmenstrual weeks. At one-year postnatal age, the GFR is predicted to be 90 percent of the adult GFR [9] (Figure 13.4)
Figure 13.4 Maturation of GFR showing the predictions of the sigmoid hyperbolic function. The abscissa is expressed as weeks of postnatal age so that 0 would be a full-term infant with a postmenstrual age (PMA) of 40 weeks.
Hepatic Biotransformation
Hepatic biotransformation is unrelated to body weight and scaled growth. Some cytochrome P450 (CYP) enzymes are present at birth (CYP3A7, uridine 5′-diphospho-glucuronosyltransferase [UGT]), while others are not (CYP2E1, CYP2D6, CYP3A4, CYP2C9). Some achieve adult activity in a few days (UGT2B7), while some require months to years to mature (UGT1A6) [10] (Figure 13.5). Hepatic activity cannot be anticipated by weight, body surface area (BSA), nor allometric scaling [11].
Figure 13.5 Development and regression of cytochrome P450 (CYP) 3A4 and 3A7 as activity measured using isoform-specific probes in human liver microsomes.
The CYP system is immature at birth and does not reach adult functionality until the first or second month (Figure 13.6). This immaturity may explain the prolonged clearance or elimination of some drugs in the first few days to weeks of life, as well as the inability of the liver to convert a precursor into its active form. The CYP450 system can be induced by a variety of drugs (e.g., phenobarbital). The age from birth – not the gestational age – determines how premature or full-term infants metabolize drugs.
Figure 13.6 The activity of many cytochrome P450 (CYP) isoforms and a single glucuronosyltransferase (UGT) isoform is diminished during the first two months of life. The achievement of adult activity over time is enzyme- and isoform-specific.
Elimination may be further affected by abnormal or decreased liver blood flow as a result of illness or surgery. Certain neonatal conditions may increase intra-abdominal pressure, and also decrease liver blood flow (e.g., closure of an omphalocele or gastroschisis, reopening of a ductus venosus, positive pressure ventilation).
Binding
Opioids, amide local anesthetics, and muscle relaxants, for example, bind to albumin and alpha-1 acid glycoprotein. The unbound (free) drug is available to cross biological membranes, bind to receptors, and achieve a therapeutic concentration. Lower concentrations of albumin and alpha-1 acid glycoprotein result in more unbound drug availability to target receptors.
Organ Function
Pharmacodynamics are affected in a substantial way by anesthetic and critical care interventions. For example, positive pressure ventilation may be associated with reduced clearance. This effect may be attributable to reduced hepatic blood flow with a drug that has perfusion-limited clearance (e.g., propofol, morphine) [3].
Pharmacodynamics: Developing Organ Systems and Drug Targeting
Most investigations center on pharmacokinetics (PK), but dosing predictions as well as monitoring for therapeutic and adverse effects also requires a better understanding of pharmacodynamics. The challenge in caring for infants is that receptors themselves are in a state of development and may therefore express themselves with much greater variability quantitatively and qualitatively [7]. For example, neuroplasticity is characteristic of the developing nervous system centrally and peripherally, and there is no reason to think that it is not integral to developmental nociception. Maturation considerations for neurochemistry and neuroanatomy may alter drug efficacy in the neonate. While anatomic and neurotransmitter mechanisms are present at birth for the processing of painful stimuli, these systems evolve through the fetal and newborn period and into adult life, and it is not likely that they function in an identical manner during these lifecycle changes. Glutamine and substance P (SP) are colocalized in C fiber terminals in the dorsal horn and facilitate pain transmission in part by activating N-methyl-D-aspartate (NMDA) receptors. In the adult rat, NMDA receptor binding is restricted to the substantia gelatinosa (SG), but at postnatal day 7 (P7) in rat models it is distributed throughout the spinal cord; the more mature and discretely distributed pattern is not achieved until P28 and parallels SP and other neuropeptides (Figure 13.7). In the rat, SP receptors move from a diffuse, nonspecific distribution in the neonate to discrete and concentrated loci in the mature SG. These developmental considerations in the expression of SP as well as other neurotransmitter systems make the neonatal SG substantially different than that found in adults, notwithstanding the achievement of calculated adequate drug levels systemically or for regional anesthetics.
Figure 13.7 Mu receptor binding sites at P1, P7, P14, and P28 and at different levels of the adult rat spinal cord. In human terms, these ages are approximately 1.5 m, 19 m, 3.3 y, and 7 y.
Cardiac effects of many anesthetic drugs are not the same in neonates because the neonatal heart is different than the adult heart, or even the heart of an older child. Neonates have a cardiac output 2–3 times that of adults, about 180–240 ml kg–1 min–1. Cardiac muscle mass is smaller in newborns and infants than in older children and adults, the ventricles are less compliant, and in addition, there is a higher resting tone at end-diastole, with a lower peak pressure achieved during systole. There is also a developmental basis for differences in excitation–contraction coupling. Tension development in the myocardium is activated by the influx of Ca2+ across the sarcolemma. As the myocardium matures, intracellular Ca2+ uptake and re-release by the sarcoplasmic reticulum plays an increasingly important role in tension development [14]. Calculations for drug dosing in neonates must take into account that neonatal myocardial depression may be more than just a dosing effect, but rather an adverse effect in the context of a substantially dissimilar myocardium.
Population Pharmacokinetics and Neonatal Variability
Classical pharmacokinetic studies involve administering single or multiple doses of a drug to a small group of subjects and then obtaining multiple samples to describe the concentration and time profile of the drug. The highly charged ethical and practical issues of conducting such studies in children, especially neonates, are obvious. A preferable approach in most pediatric situations is the population pharmacokinetics (PPK) approach, combining fewer samples of individual data with blood sampling from a large target population at varying times.
Population pharmacokinetics is focused on absorption, distribution, metabolism, and excretion, using mathematical and statistical modeling, typically nonlinear mixed effects modeling (NONMEM). For example, using PPK and NONMEM modeling, Anderson et al. have shown that size explains 49.8 percent, age 18.2 percent, and renal function 14.1 percent of clearance variability of vancomycin in neonates [7].
Better Prediction Through Better Modeling?
In an attempt to incorporate the many aspects of pharmacokinetic pharmacodynamic modeling, particularly with regard to infants and small children, Simcyp® software (Simcyp Limited, Blades Enterprise Center, John Street, Sheffield S2 4SU, UK) integrates demographic, genetic, physiological, and pathological information on adults with in vitro data on human drug metabolism and transport to predict population distributions of drug clearance (CL) and the extent of metabolic drug–drug interactions (Figure 13.8). The algorithms have now been extended to pediatric populations by incorporating information on developmental physiology and the development/maturation of specific cytochrome P450s [15]. Simcyp® algorithms have been reported to provide reasonable estimates of the in vivo drug clearance of 11 drugs commonly used in neonates, infants, and children [16].
Figure 13.8 Simulated concentration–time profile for (a) midazolam alone (black line); (b) midazolam alone (black line) and in the presence of rifampicin (gray line); (c) midazolam alone (black line) and in the presence of rifampicin and fluconazole (gray line); and (d) midazolam alone (black line) and in the presence of rifampicin, fluconazole, and clarithromycin (gray line).
Inhalation Anesthetics
The effects of chloroform are more quickly produced and also subside more quickly in children than adults, owing no doubt to the quicker breathing and circulation [17].
So observed John Snow about inhaled anesthetics in children, providing early insight into fundamental differences and paving the way for pediatric anesthesiologists to be leaders in pediatric pharmacology.
Most of the PK differences are due to allometrically scaled parameters. Scaled branching and volume of the circulation is directly related to metabolic activity, since heat delivery from the core to the periphery has to be exquisitely balanced to maintain the homeothermic state. Developmental blood chemistry balance (differences in total proteins, albumin, cholesterol, and total body water) also affects tissue/blood solubility such that lower solubility is characteristic of neonates. These differences add to enhancing the speed of induction and the rapidity of therapeutic as well as adverse effects in children. These PK effects are outlined in Table 13.1.
Children | Adults | Implication | |
---|---|---|---|
Alveolar ventilation: FRC ratio | 5:1 | 1.5:1 | Enhanced wash-in |
Cardiac output | 20 dl min–1 (2 dl kg–1 min–1) | 50 dl min–1 (0.75 dl kg–1 min–1) | Shorter time to equilibrium and enhanced speed of induction |
Tissue:blood solubility | Neonates have lower levels of total protein, albumin, and cholesterol, and higher levels of total body water | ||
Total protein | 50 g L–1 | 70 g L–1 | Less protein available for binding therefore increased free fraction |
Albumin | 30 g L–1 | 37 g L–1 | Less protein available for binding therefore increased free fraction |
Cholesterol | 1.3 g L–1 | 2.09 g L–1 | Lower cholesterol, lower solubility, and increased free fraction |
Total body water | 689 g kg–1 | 605 g kg–1 | Greater dilution |
In addition to the differences in PK outlined above, anesthetic potency also influences the ability to rapidly adjust an inhaled anesthetic, whether during induction or any other phase. The minimum alveolar concentration (MAC) has been the standard used to express anesthetic potency, and in general the lower the MAC the more potent the anesthetic. Lipid solubility of the specific agent is inversely related to the MAC of that agent; in addition, MAC has long been recognized to be different for different age groups (Figure 13.9).
Figure 13.9 MACs of four commonly used inhaled anesthetics plotted vs. age. MAC is highest in infants 3–6 months of age.
Carbon monoxide (CO) can be produced in subclinical concentrations by all of the current methyl ethyl ethers (desflurane > isoflurane > halothane and sevoflurane) in the presence of desiccated soda lime or baralyme. Newer CO2 absorbents (those that contain a lithium-based catalyst) do not have the typical strong bases (KOH, NaOH) and do not result in carbon monoxide or Compound A production. Furthermore, they are not significantly exothermic. Mitigating effects in infants and children include a lower total minute CO2 production despite a higher per kilogram per minute value due to increased oxygen consumption.
Post-anesthesia delirium has been associated with all of the currently utilized inhalation agents, and there seem to be numerous cofactors that make it all the more difficult to discriminate a single etiology [19]. Again, this is a difficult assessment in the neonate and infant.
Specific adverse effects of inhalation agents more prominent in the neonatal and infant age group include:
Myocardial effects. Cardiac muscle mass is smaller in newborns and infants than in older children and adults, the ventricles are less compliant, and in addition there is a higher resting tone at end-diastole, with a lower peak pressure achieved during systole [20]. Conduction delays are almost uniformly predictable with halothane but are variable with the newer ethers.
Baroreceptor effects. Baroreception, like myocardial performance, “matures” postnatally. The sensitivity of carotid baroreceptors is reset as arterial pressure increases throughout the last third of gestation and the first postnatal month. Postnatally, baroreflex sensitivity increases from birth to six weeks of age with the most rapid changes occurring after two weeks of age [21–23]. Inhalation anesthetics may aggravate the relative insensitivity of the baroreceptor response in neonates [24,25].
Respiratory effects. All potent volatile agents depress minute ventilation and shift the CO2 response curve “up and to the left” in infants, more so than in older children and adults. Comorbidities such as the Chiara II malformation commonly associated with spina bifida worsen this finding.
Sevoflurane
Sevoflurane has become the most popular inhalation anesthetic for children because of its ease of acceptance, non-pungency, and relative forgiveness with regard to adverse cardiovascular effects in comparison with the previous generation’s gold standard, halothane [26]. After passing through soda lime or baralyme, sevoflurane is degraded into five compounds (Compounds A–E). Only Compound A accumulates significantly in the circuit when the following conditions are met: increased temperature, low water content, high concentration of sevoflurane, the use of baralyme rather than soda lime, or the use of new soda lime.
Sevoflurane has half the potency of isoflurane and one-third that of halothane. Interestingly, sevoflurane MAC does not increase with decreasing age, in contrast to the other inhaled agents. For unclear reasons, the additive effect of nitrous oxide is not as prominent with sevoflurane as it is with other agents.
There seem to be some unique electroencephalographic effects of sevoflurane, specifically epileptiform-like patterns, as well as rare facial or limb twitching during light levels that seem to disappear in deeper planes. The significance of these findings is also unclear and no adverse outcomes have been linked to these findings.
Desflurane
Desflurane is isoflurane with replacement of the chlorine with a fluorine – but what a difference pharmacologically! The blood–gas and tissue–blood solubilities are dramatically decreased and consequently the uptake and distribution as well as the washout of desflurane is the most rapid of any of the potent inhaled anesthetics. Unfortunately, the pungency and airway irritability of desflurane make it a poor clinical choice for inhalation inductions in children, but maintenance and emergence are not problematic following an intravenous induction or an inhalation induction with a less pungent (i.e., sevoflurane) anesthetic.
Emergence, as expected, is quite rapid because of the rapid washout, and therefore a plan for preemptive postoperative analgesia should be coupled with the use of desflurane as the principal anesthetic. There would appear to be little need for utilizing desflurane clinically in neonates and infants for the sake of facilitating rapid emergence or even rapid intraoperative adjustments in anesthetic depth because of their relatively small tissue stores and rapidity of equilibration with changes in the inspired agent concentration.
Isoflurane
The wash-in and wash-out of isoflurane is slower than desflurane or sevoflurane. Like desflurane, it has a pungent odor, making it less favorable for mask induction in children; maintenance and emergence do not present significant clinical issues, the pungency notwithstanding. Infants anesthetized with isoflurane do not exhibit the same degree of reduction in heart rate that results from halothane use.
Nitrous Oxide
Nitrous oxide has a long history of use in infants and small children. Its low blood–gas partition coefficient and low potency have two very appealing features for use in infants – it works rapidly and because of its lower potency may actually provide a greater margin of safety for infants. As a carrier gas it has featured prominently throughout the history of pediatric anesthesia because of the second gas effect.
Caution must be applied with regard to its tendency to occupy air-filled spaces due to its blood–gas partition coefficient being 34-fold greater than nitrogen. Inasmuch as bowel obstruction is one of the more common newborn surgical emergencies, clinicians must be circumspect about the use of nitrous oxide. More subtle considerations include its effect on the inhibition of methionine synthetase, an important consideration for infants with their developing bone marrow and nervous systems.
Intravenous Induction Agents
Barbiturates have long been the standard intravenous induction agent for all age groups. Special pediatric considerations over the years have been recognized – for example, prolongation of sleep times of newborns (presumably due to greater blood–brain barrier permeability), impaired biotransformation due to glucuronic acid conjugation immaturity in the first several weeks of life, and an increased dosing requirement in the newborn period and especially for the first six months of life.
Thiopental is a highly lipid-soluble ultra-short-acting barbiturate that results in rapid unconsciousness. Plasma protein binding is reduced in the neonate, resulting in a doubling of the plasma-free fraction in comparison to children and adults. In addition, clearance is substantially reduced in infants (less than half that of adults). Because its effect is primarily terminated by redistribution, recovery in infants nevertheless is on par with older children and adults. Thiopental has not been available in the United States since 2011.
Methohexital, a more potent barbiturate than thiopental, has not commonly been used by the intravenous route in children. It has mainly been used rectally, and a body of literature has described blood levels and reliability as an induction agent in infants and older children, but not in neonates.
Virtually all intravenous inductions, when chosen, are currently accomplished with propofol. Its short duration of action because of rapid redistribution and biotransformation permit its use by bolus as well as continuous infusion. Glucuronidation is the major route of elimination. Uridine 5-diphosphate-glucuronosyltransferases (UGTs) occur at varying times during early infancy, and some UGTs are present at birth while others develop during early infancy. A decrease in total requirements may be due to a net immaturity of neonatal enzyme systems while a decrease in induction dose requirements probably has more to do with a greater tendency toward hypotension and bradycardia in infants [27]. Propofol may also limit its own biotransformation by virtue of its effect on cardiac output and hepatic blood flow in neonates and infants, although few studies exist describing neonatal propofol pharmacology. Other features noted in patients who can self-report them – pain on injection, antiemetic effects – may occur in infants as well, but are probably more difficult to detect.
Ketamine remains a significantly utilized alternative to propofol because it preserves cardiovascular stability and rapidly results in unconsciousness. Infants typically require a much larger dose to produce lack of movement with induction than older children. In infants younger than three months, the volume of distribution is similar to older infants, but the elimination half-life is prolonged. Clearance is reduced in younger infants with reduced metabolism; slower renal excretion is the likely cause. While respiratory efforts tend to be preserved in children and adults, respiratory depression and apnea may be the result of higher doses required in infants. Muscular (extensor) spasm may also occur, as well as elevations in pulmonary artery pressure in infants with an underlying elevation in baseline pulmonary artery pressure (congenital heart disease).
Sedatives
Benzodiazepines
Benzodiazepines are commonly used in pediatric patients to provide sedation, anxiolysis, anterograde amnesia, as well as anticonvulsant therapy. Benzodiazepines bind to gamma amino butyric acid (GABA) receptors, which are distributed throughout the frontal cortex and cerebellum in varying densities during the fetal through neonatal period [28]. Metabolism occurs in the liver by cytochrome P450 enzymes with excretion in the urine. The clearance of these drugs varies depending on clearance of the parent drug and its metabolites, as well as the intrinsic physiology of neonates and their comorbidities.
Midazolam is lipid-soluble at physiological pH and therefore has a rapid onset compared to other benzodiazepines. It also has a shorter half-life compared to diazepam and lorazepam. Absorption via the gastrointestinal tract is slower in premature neonates, full-term neonates, and young infants secondary to delayed gastric emptying [12,29]. Rectally administered midazolam may have faster absorption, but the bioavailability may vary.
Midazolam is converted to the active metabolite 1-hydroxymidazolam (1-OH-midazolam) by cytochrome P450 enzymes CYP3A4, CYP3A5, and, to a lesser extent, CYP3A7 [30,31]. 1-OH-midazolam has a shorter half-life compared to other benzodiazepine metabolites, yet in neonates the half-life can be anywhere from four to six hours, compared to two hours in adults [30,31]. CYP3A4 and CYP3A5 are activated during the first week of life [29] and, therefore, the formation of 1-OH-midazolam is decreased and clearance of midazolam is slower in preterm and full-term neonates [31,32].
Diazepam is a longer-acting benzodiazepine commonly administered via oral, intravenous, or rectal routes. It is also metabolized by cytochrome P450 enzymes into desmethyldiazepam, which has a longer half-life in premature and term neonates compared to older children [28]. While highly bound to plasma proteins, the amount of unbound drug in newborns is double that of adults, so there is a potential for accumulation and a prolonged drug effect. The adult ratio of bound:unbound drug is reached by the first week of life.
Lorazepam is usually administered intravenously to the neonatal population. While some PK data exist for intramuscular and submucosal routes in the healthy adult population [33], there is still a lack of data in neonates and infants. It is a long-acting benzodiazepine, with half-life ranging from 18 to 73 hours in neonates, compared with 10–12 hours in older children and adults [34]. While also metabolized by cytochrome P450 enzymes, its metabolite is inactive. Lorazepam is about 75 percent plasma protein bound and also excreted in urine.
Any comorbidities that cause changes in hemodynamic, renal, or hepatic function or concomitant medication administration may also alter metabolism and excretion of benzodiazepines, leading to accumulation and prolonged sedation. Burtin et al. showed decreased clearance by 30 percent in neonates receiving sympathomimetic drugs, which the authors attributed to compromised cardiac output in critically ill neonates [32]. De Wildt et al. showed newborns exposed to indomethacin for treatment of patent ductus arteriosus (PDA) had higher clearance of midazolam compared to those who were not exposed; however, a decrease in renal function with the use of medications such as indomethacin and NSAIDs should also be considered in the neonate receiving benzodiazepines [31].
Interindividual variability in expression and activity of the CYP enzymes may also account for the interindividual variability in the clearance of benzodiazepines in neonates [28,29,30,32]. Exact neonatal dosing for a target concentration is difficult. Moreover, drugs like midazolam, which is considered short-acting in adults, may not have the same clinical profile in neonates. Benzodiazepines should therefore be administered and titrated to clinical effect, with the potential for accumulation in mind.
Dexmedetomidine
Dexmedetomidine is a selective α2-agonist increasingly used in pediatric anesthesia for sedation or as an adjunct [35–39]. It has an α2:α1 activity of 1620:1, compared to clonidine’s 200:1, and acts on the locus ceruleus to provide sedation and analgesia. Dexmedetomidine is metabolized in the liver by glucuronidation, hydroxylation, and N-methylation. It is about 90 percent protein-bound to albumin, and is eliminated primarily in the urine. In adults, dexmedetomidine has a half-life of about two hours, compared to clonidine’s half-life of 12–24 hours. In newborns, the half-life is about 7.6 hours in preterm neonates and 3.2 hours in term neonates [40]. Using PPK, Potts et al. found clearance in neonates in the ICU to be about one-third that of adults. Clearance reaches about 87 percent of adult values by one year of age [41]. All of these findings are attributed to immature metabolic and elimination pathways in the neonate.
The hemodynamic profile of dexmedetomidine has also been widely studied in adults and children [42–46]. Its known effects on heart rate, and blood pressure, have also been seen in neonates [39,47]. While its safety profile continues to be studied and its use generally accepted among the pediatric population, it’s PK profile in the neonate may warrant lower dosages to avoid unwanted effects [40].
Opioids
Opioids are commonly used in neonates for analgesia and sedation. Similar to benzodiazepines, several studies have shown age to be the most important variable that affects metabolite formation, morphine clearance, and plasma concentration [48,49]. In neonates and infants, the beta elimination half-life of opioids is prolonged compared to adults and older children, so respiratory depression may also be prolonged. Opioids are primarily metabolized in the liver via oxidation, except morphine which undergoes glucuronidation and remifentanil which clears via ester hydrolysis [50]. Most opioids are also protein-bound to albumin and α1-acid glycoprotein, both of which are decreased in the neonate and, therefore, cause increased concentrations of unbound drug. Excretion is mainly via the kidneys.
Morphine is metabolized into morphine-3-glucoronide (M3G), which has no analgesic properties, and morphine-6-glucoronide (M6G), the active metabolite. The plasma concentration ratio of M6G:morphine is higher after oral administration than after intravenous administration due to the first-pass mechanism [50]. Among neonates and infants, the M3G metabolite also predominates [49]. Since there is less of the active metabolite, M6G, there may be a need for a higher concentration for an analgesic effect compared to older children and adults. As a result, there may also be more undesirable effects of the opioid, such as hypotension, bradycardia, hypercarbia, and seizures. Using a PPK approach, Bouwmeester et al. found that higher morphine infusion rates were necessary for infants and young children to achieve a target plasma concentration of 10 ng mL–1, with the highest rate required at one year of age. Total body clearance of morphine reached 80 percent that of adults by age six months [49]. Once M3G and M6G metabolites are formed, they are excreted via the kidneys. Excretion then becomes a function of GFR. Because neonates have decreased glomerular and tubular function, the rate of elimination will also be slower and lead to accumulation of active metabolites.
Fentanyl is highly lipid-soluble and 50–100 times more potent than morphine, with rapid onset and shorter duration. It is also known to be relatively cardiac-stable and used widely in cardiac anesthesia as well as for sedation in the NICU. Fentanyl is metabolized in the liver by CYP3A4 enzymes via N-dealkylation and hydrozylation into inactive metabolites, and excreted in the urine. It is mostly bound to albumin, and less so to α1-acid glycoprotein. In adults, metabolism of fentanyl is not significantly altered with liver cirrhosis, but may be altered with impaired hepatic blood flow [50]. Transmucosal and transdermal routes of administration have been used and studied in older children [48,49], but their use and pharmacokinetic profile has not been delineated in neonates.
Remifentanil is a synthetic opioid with a potency similar to that of fentanyl. It is fast-acting and has a very short half-life. In adults, the beta elimination half-life is 9.5 min, while studies in neonates show it to be 3.4–5.7 min [51,52]. It is unique compared to other opioids because it undergoes ester hydrolysis in the plasma. The clearance rate in neonates is about twice as long as adults; however, liver disease, kidney disease, or the use of cardiopulmonary bypass do not appear to affect the clearance of remifentanil [51,53].
Sufentanil is also a highly lipid-soluble, synthetic opioid 5–10 times more potent than fentanyl. Like fentanyl, it has been shown to be appropriate for use in pediatric cardiac patients because it maintains relative hemodynamic stability. Sufentanil is metabolized by N-dealkylation into inactive metabolites and excreted in the urine [50]. It is mostly bound to α1-acid glycoprotein [54,55]; thus, more active, unbound drug can be found in the plasma of neonates. Moreover, protein binding is affected by plasma pH such that alkalosis would decrease and acidosis increase binding [55].
Alfentanil is another fast-acting opioid with a short half-life (5–10 min). It is one-third less potent than fentanyl and bound mainly to α1-acid glycoprotein. Unlike sufentanil, plasma pH does not affect its protein binding [50]. Alfentanil is metabolized by CYP3A4 and excreted in the urine. Like fentanyl, muscle rigidity has been found to occur in neonates receiving alfentanil infusions [56].
Acetaminophen
Acetaminophen is a commonly used over-the-counter drug to treat fever and pain. It is water-soluble and therefore has potentially a lower peak concentration in neonates, given their higher volume of distribution. This would lead to less effectiveness and the need for a higher loading dose. While maturation may account for about one-third of the variability in absorption, other factors that can contribute to slower absorption in the neonate include their slower gastric emptying, and concomitant administration of food or other medications [57]. Absorption after rectal administration is much faster compared to oral administration, but the bioavailability of the drug may also have interindividual variability, depending on where in the rectum it is delivered. Although metabolism is by glucuronidation in a mature system, acetaminophen undergoes primarily sulfate conjugation and renal excretion in the neonate. Thus, one can expect slower clearance and the potential for accumulation and toxicity. Adult clearance is usually reached by 12 years of age [58].
Muscle Relaxants
Neuromuscular blockers (NMBs) are used intraoperatively and postoperatively for endotracheal intubation, immobilization and decreasing barotrauma, oxygen consumption, and anesthetic or sedative requirements. While some data exist in neonates and infants, the only current consensus guidelines for use of NMBs in the pediatric population comes from the United Kingdom [59]. However, this consensus only addresses use in critically ill children, and does not include neonates.
Categorized into depolarizing and nondepolarizing agents, NMBs essentially bind directly or competitively to acetylcholine receptors (AChRs) on the neuromuscular junction (NMJ) and block transmission of the electrical impulse that causes muscle contraction. A higher volume of distribution generally means a higher concentration of drug needed to reach motor endplates; however, the number and type of AChRs present change throughout development. In neonates, maturation of the NMJ occurs during the first two months of life [60]. Mature AChRs are produced only in the junctional area, but an immature type of receptor is also formed during development and in cases of denervation. These immature AChRs are located in areas outside of the junctional area, have a greater affinity for succinylcholine than nondepolarizing agents, and have sodium channels that are more easily depolarized and open for a longer period of time [61]. Clinically, this may result in variability and unpredictability of results after administration of NMBs. Older children have a higher muscle mass:fat ratio compared to infants, so will have more AChRs and require a higher drug concentration for a desired effect [60].
Succinylcholine is the only nondepolarizing agent used clinically and it is well established that larger doses are required in neonates and infants because of their increased volume of distribution [62]. Recommended doses are 2–3 mg kg–1 in neonates and infants, 1 mg kg–1 in older children, and 4 mg kg–1 for intramuscular administration. Succinylcholine is hydrolyzed by plasma cholinesterases into succinic acid and choline, so conditions like liver disease and atypical plasma cholinesterase may prolong its metabolism. Acute denervation injuries, crush injuries, and burns can result in a hypersensitivity to succinylcholine and lead to hyperkalemia, hyperkalemic dysrhythmias, or cardiac arrest. Children with undiagnosed myopathies, such as Duchenne muscular dystrophy, are at risk for malignant hyperthermia after succinylcholine administration. These and other known serious side-effects have led to the black box warning that recommends limiting use of succinylcholine to rapid sequence intubation and emergent securing of an airway in children [63].
The nondepolarizing muscle relaxants can be categorized into long-, intermediate-, and short-acting groups. With the exception of cisatracurium, they are primarily metabolized by the liver, with biliary and renal excretion. The comparative effective dose to produce 95 percent depression of twitch height (ED95) for commonly used NMBs is listed in Table 13.2.
ED95 (mcg/kg) | Onset (min) | Duration (min) | Recovery (min) | |||||||||
---|---|---|---|---|---|---|---|---|---|---|---|---|
Infant | Child | Adult | Infant | Child | Adult | Infant | Child | Adult | Infant | Child | Adult | |
Pancuronium | 66 | 93 | 67 | 2–5 | 2–4 | 3–5 | – | 24 | 22 | – | 33 | 37 |
Cisatracurium | – | 41 | 48 | – | 1–3 | 2–4 | – | 25 | 25 | – | 40 | 45 |
Vecuronium | 47 | 81 | 43 | – | 1–3 | 2–3 | – | 22 | 26 | 73 | 35 | 53 |
Rocuronium | 255 | 402 | 350 | – | 0.8–1.5 | 1–2 | – | 27 | 42 | – | 42 | 69 |
Succinylcholine | 650 | 400 | 300 | 0.5 | 0.6 | 1 | 4.6 | 5.8 | 6.0 | 7.4 | 9.7 | 10 |
Pancuronium is a bisquaternary aminosteroid, long-acting, nondepolarizing muscle relaxant. It is favored in critically ill pediatric patients because of its known sympathomimetic effects resulting in tachycardia, hypertension, and increased cardiac output. There are sparse data on neonates. Some have shown the ED50 of children to be greater than that of adults overall, [64] and the ED95 to be greater in children compared to neonates, likely due to the difference in volume of distribution and protein binding.
Vecuronium is a monotertiary, monoquaternary aminosteroid derivative of pancuronium that is an intermediate-acting NMB. It does not have the same hemodynamic effects as pancuronium. Meretoja et al. found that neonates and infants less than one year had a lower ED95 compared to children aged 2–13 years, which the authors again attribute to the difference in volume of distribution and protein binding between age groups [65]. These differences also account for the prolonged effect of vecuronium in infants compared to older children [66].
Rocuronium is a monoquaternary aminosteroid, intermediate-acting NMB, that is six times less potent and has faster onset than vecuronium and pancuronium [67]. Its ED95 also appears to be age-dependent and it does not cause histamine release or hemodynamic changes [67,68].
Cisatracurium is the cis–cis isomer of atracurium, a bisquaternary ammonium benzylisoquinolinium that primarily undergoes Hoffman elimination. It is organ-independent for clearance and desirable for use in neonates and children with renal impairment. Inasmuch as Hoffman elimination depends on pH and temperature, lower pH and lower temperature will increase time to elimination. This should be considered when cisatracurium is used in patients with a metabolic acidosis. Cisatracurium is 3–4 times more potent than atracurium and does not cause significant histamine release [59]. Few studies exist for cisatracurium in infants and neonates. Some suggest that in neonates with congenital cardiac repairs, recovery from cisatracurium infusion is faster than from vecuronium infusion, but there was no difference in general patient outcomes.
References
Airway management poses a challenge due to the newborn’s small size, unique anatomy, and physiology. Thus, the approach to newborn airway management differs from that for older children and adults. This chapter provides an overview of a spectrum of topics important in newborn airway management, including airway anatomy and physiology, tips for adequate ventilation and intubation, one-lung ventilation techniques, management of mediastinal masses, difficult airway management, and airway management in infants undergoing mandibular distraction and tongue–lip adhesion.
Infant Airway Anatomy and Physiology
The infant respiratory system is not simply a miniaturized version of the adult system. In addition to anatomical differences (Table 14.1), significant differences in respiratory physiology exist. The newborn’s smaller airway radius creates greater resistance to air flow that in turn increases the work of breathing and offers added pressure on the lumen of the airway. Any amount of airway narrowing caused by edema, and congenital malformations, can have serious consequences on the overall work of breathing and respiratory function [1]. Combined with the infant’s higher chest wall compliance, the narrow airway also increases the incidence of airway obstruction [2]. The reduced outward recoil of the highly compliant chest wall produces low transpulmonary pressures and causes small peripheral airways to collapse during tidal breathing, predisposing to ventilation/perfusion mismatch (V/Q) [2,3] and oxygen desaturation.
Infant airway anatomy | Clinical significance in airway management |
---|---|
Larger occiput | Supine positioning on a flat surface causes neck flexion that may lead to airway obstruction; consider use of a shoulder roll |
Shorter and narrow hypopharynx, higher larynx | Barrier to aligning oral, laryngeal, and tracheal axes for laryngoscopy |
Relative macroglossia, shorter mandible | Reduced upper airway space to displace tongue into the submandibular space |
Vocal cords angled more cephalad rather than at 90 degrees | Vocal cords appear more anterior, potentially more traumatic tracheal tube insertion |
U-shaped, long, floppy epiglottis | Use of semi-straight laryngoscope blade is preferable |
Cartilaginous larynx and trachea (not yet calcified) | Extra compliance increases incidence of airway obstruction with positive-pressure ventilation |
Funnel-shaped airway with cricoid cartilage as the functionally narrowest point; elliptical-shaped cricoid ring with larger anterior–posterior diameter | Affects the airway seal of both cuffed and uncuffed tracheal tubes |
The lungs of newborns are very compliant, contributing to low functional residual capacity (FRC) and dynamic airway collapse [3]. Combined with their higher oxygen consumption, this may lead to rapid oxygen desaturation during apneic periods [4,5]. Infants also have increased carbon dioxide production when compared with adults. Therefore, infants require a higher respiratory rate to achieve a relatively higher minute ventilation needed to eliminate carbon dioxide [15].
Practical Tips to Adequately Ventilate and Intubate Young Infants
Bag-Mask Ventilation
Bag-mask ventilation (BMV) is a critical component of airway management as it provides oxygenation and ventilation prior to placement of a definitive airway [16,17]. Selection of an appropriate-sized mask is essential to obtain an adequate seal. The mask should fit over the bridge of the nose, seal on the sides of the nasolabial folds, and fit between the lower lip and chin.
BMV Technique
The facemask should be held tightly to the patient’s face with the thumb and forefinger of the anesthesia providers’ left hand while the other fingers lift the mandible toward the facemask. Care should be taken to avoid excessive pressure on the submandibular soft tissue as this may cause airway obstruction. The pharyngeal space can be opened via displacement of the mandible, chin lift, jaw thrust, and atlanto-occipital joint extension, leading to greater patency of the airway [18]. While the left hand seals the mask, the right hand is used to compress the reservoir bag on the anesthesia breathing circuit to generate positive pressure. Peak inspiratory pressure should be kept below 20 cmH2O to avoid gastric insufflation of air. If a one-handed approach is insufficient, additional help may be recruited to attempt a two-handed technique in which the initial provider either maintains the same position while the secondary provider performs a jaw thrust, or uses both hands to secure the facemask while the secondary provider squeezes the reservoir bag. Additionally, use of an oral or nasal airway may help overcome difficulties in mask ventilation.
Tracheal Intubation Technique
Endotracheal intubation via direct laryngoscopy is normally chosen unless specific indications require a different approach. When intubating an infant, placing a rolled towel under the supine patient’s neck or shoulders helps improve airway patency. A laryngoscope can then be inserted to the right of the tongue to facilitate visualization. Because the infant’s epiglottis is U-shaped, long, and floppy, and less in line with the trachea, a straight laryngoscope blade is preferable to a curved blade since it directly displaces the epiglottis out of view rather than using the vallecula’s ligamentous connection with the epiglottis [6]. If the vocal cords cannot be visualized, gentle downward pressure at the level of the thyroid or cricoid cartilage may be helpful.
The tracheal tube (TT) should be introduced from the right side of the patient’s mouth with the natural curve of the tube directed anteriorly. Insertion from the right is necessary to maintain visualization of the glottic opening. The TT should be advanced so that its distal end is midway between the vocal cords and carina. It is imperative to confirm position of the TT after securing it and anytime thereafter when there is a change in position because the infant’s short trachea allows the TT to easily advance into the right mainstem bronchus [19]. Diminished breath sounds in the left lung may indicate right endobronchial intubation.
Use of a straight blade via a retromolar approach may allow better glottic visualization in infants with large tongues or small mandibles. A straight blade is inserted into the right side of the mouth, while the head is rotated to the left. This technique allows the tongue to be completely bypassed. The tip of the laryngoscope blade is then used to lift the epiglottis when it comes into view.
One-Lung Ventilation, Video Assisted Thoracoscopy
Video assisted thoracoscopic surgery (VATS) is a less invasive approach to thoracoscopic surgery, making it advantageous to use in patients. Successful VATS requires proper one-lung ventilation (OLV), with efforts to promote a quiet surgical field, avoid contamination of the normal lung, prevent hypoxemia, and minimize respiratory insults inherent in the ventilation technique [20].
Physiology of OLV
One-lung ventilation decreases functional residual capacity and tidal volume, predisposing to higher incidence of V/Q mismatch and oxygen desaturations. Hypoxic pulmonary vasoconstriction (HPV) diverts blood away from atelectatic lung tissue, normally minimizing V/Q mismatch. However, inhalational anesthetic agents in conjunction with high or low fractions of inspired oxygen reduce the HPV response [21,22].
Patients are placed in lateral decubitus position during VATS to allow for optimal access of the affected lung. However, this positioning has an unfavorable effect on respiratory physiology due to infants’ compliant lungs, underdeveloped rib cage, and low functional residual capacity [3]. When the healthy lung is ventilated in the dependent position, it will have a decrease in compliance, potentially increasing the incidence of airway closure [23]. In adults, the lateral decubitus position increases the hydrostatic pressure gradient between the dependent and nondependent lungs, favorably increasing perfusion in the healthy lung while decreasing perfusion in the diseased lung. This results in relatively even distribution of ventilation and perfusion. In infants, however, this hydrostatic pressure gradient when in the same position results in uneven distribution of ventilation and perfusion as a result of their smaller size [24]. Therefore, infants may be at risk for hypoxia during OLV in the lateral decubitus position.
OLV Techniques
The repertoire of devices suitable for OLV of the infant airway is limited. Currently, OLV in infants can be performed with a single-lumen TT or bronchial blocker, including the Fogarty® embolectomy catheter and Arndt Endobronchial Blocker® (Cook, WEB, Critical Care, Bloomington, IN, USA), because they are available in sizes suitable for the infant airway [25–27]. For neonates, these options may be more limited because of sizing availabilities in the small neonate or ex-preemie. In these patients, the surgeon may find retraction of the operative lung suitable while intermittently ventilating this lung during periods of oxygen desaturations.
A single-lumen TT can be advanced to isolate the bronchi after intubation. However, intubating the left main bronchus may be challenging. Suggested techniques to facilitate blind intubation of the left bronchus include use of a stylet to curve the distal end of the tube [28] or use of a distally curved rubber bougie which can be inserted blindly into the left main bronchus, followed by insertion of the TT over the bougie [29]. It should be noted that the right upper lobe bronchus may be obstructed when intubating the right main bronchus, causing hypoxia. Also, a single-lumen TT may not adequately seal the bronchus, risking contamination of the healthy lung and preventing collapse of the pathologic lung [25,30].
The bronchial blocker (BB) may also be used to achieve OLV in infants. An advantage of a BB versus a TT in sealing the bronchus is that the former provides a tighter seal. The Fogarty embolectomy catheter and Arndt Endobronchial Blocker have been successfully used for lung isolation in infants [31]. Proper placement of the Fogarty catheter is facilitated by bending the tip of its stylet toward the bronchus on the operative side. Positioning can be directed and confirmed with fiberoptic bronchoscopy (FOB) or fluoroscopy. Infants may require parallel insertion of the Fogarty catheter alongside the TT [32]. One such method for parallel insertion involves intubating the bronchus on the operative side with an TT, advancing a guide wire through the TT and into the bronchus, then removing the TT, and finally advancing the blocker over the guidewire into the bronchus [32]. It should be noted that since embolectomy catheters have low compliance and high pressure properties, the balloon should be inflated with incremental volumes of air until the airway is sealed. This will prevent over-distention, which can damage or rupture the airway [27,33].
More recently, the Arndt Endobronchial Blocker has been successfully used for OLV in small infants [31,34]. An advantage of the Endobronchial Blocker is that a central channel is present to allow for complete lung deflation. This feature is not present in the Fogarty catheter, so complete lung deflation may not be possible when using this device. The Arndt Endobronchial Blocker has a flexible wire loop and a three-part swivel adaptor. This adaptor allows for insertion of an FOB and balloon-tipped BB in two ports, and use of the third port as a ventilation circuit. The balloon is high-volume, low-pressure, and comes in two shapes to allow for optimal fit into the two mainstem bronchi: spherical for the right main stem bronchus and elliptical for the left main stem bronchus. Additionally, it is available in 1.0 cm length, corresponding to the length of the right main stem bronchus in the average child of two years of age. This enables it to fit entirely within the shorter right main stem bronchus, preventing obstruction of the upper lobe bronchus [35]. While coaxial insertion of the blocker and use of FOB can be accommodated in the relatively larger airway of older children, infants require a 5 French size Arndt Endobronchial Blocker and parallel insertion of the blocker due to the small diameter of their airway [27,36]. Additionally, passage of an FOB in an indwelling TT may be restricted in infants, in which case fluoroscopy may be used to guide insertion [36].
Pre- and Intraoperative Management of Mediastinal Masses
Mediastinal masses encompass a diverse group of benign and malignant tumors that may be classified based on their location in the mediastinum [37]. These tumors have respiratory and hemodynamic consequences. In general, tumors of the anterior mediastinum comprise 46 percent of mediastinal masses in infants and children [38–41]. These tumors cause the most severe complications relating to compression of the airway and vasculature. It should be noted, however, that pediatric patients have an increased incidence of neurogenic tumors compared to adults [38–41], and these masses complicate airway management [42]. Although mediastinal tumors are rare in neonates, case reports have described mediastinal teratomas and cystic hygromas as important causes of respiratory distress in this population [43,44]. Cystic hygromas may be localized to the mediastinum or arise in the neck and extend down into the mediastinum, causing airway obstruction [43,45].
Tumors on the extreme end of the disease spectrum pose the greatest difficulty to management in the perioperative period, leading to severe cardiorespiratory complications and even death in children [46,47]. These problems can be exacerbated by general anesthesia [48,49]. As management of mediastinal masses in pediatric patients typically involves surgical biopsy or resection under general anesthesia with tracheal intubation, an understanding of risk factors for complications under anesthesia, preoperative testing, and intraoperative techniques to manage these tumors is necessary.
Mediastinal masses may lead to several forms of intrathoracic compromise, including one or a combination of compression of the tracheobronchial tree, compression of the pulmonary artery and heart, and superior vena cava syndrome [50,51]. Pulmonary symptoms such as dyspnea at rest, postural dyspnea, and stridor are strong risk factors for intraoperative airway complications [50,52], and symptoms such as syncope, arrhythmias, head and neck edema, and cyanosis predict cardiovascular complications in pediatric patients [50,52]. The absence of such symptoms, though, does not exclude the possibility of airway or circulatory collapse under anesthesia [53,54]. Moreover, symptoms that are described may be worse in the postoperative period due to the effects to general anesthesia [47].
Careful preoperative testing can help gauge the severity of a patient’s respiratory compromise and estimate the risk of adverse events under anesthesia. Computed tomography (CT) scanning is routinely used and provides the greatest amount of information concerning size of the mass, its location, and incursion on surrounding structures. Pulmonary function testing may reveal limited expiratory flow predictive of airway collapse under anesthesia [46], while echocardiography is useful in characterizing masses that may not compress the airway or cardiovascular systems but which will cause airway obstruction and cardiovascular collapse after induction of general anesthesia [55]. Additional tests may be used to enhance anesthetic management for specific tumors. A thyroid scan may be helpful when a thyroid mass is suspected, but should be performed first if iodinated contrast is used in the CT scan. Magnetic resonance imaging (MRI) may elucidate information on a neurogenic tumor, and positron emission tomography can be used for follow-up of germ cell tumors after treatment.
Intraoperatively, the anesthesiologist may employ a variety of techniques to manage mediastinal masses. Inhalational induction with maintenance of spontaneous respiration is recommended for infants with anterior mediastinal masses [56]. As functional residual capacity may be reduced under anesthesia, continuous positive airway pressure may also be helpful [46]. Elevation of the head of the bed mitigates undesirable effects of the supine position such as reduction of thoracic volume due to cephalad displacement of the diaphragm [47], and airway patency can also be maintained by placing the patient in partial or full lateral decubitus position [46].
When performing tracheal intubation, the clinician should consider placing an armored endotracheal tube without the assistance of neuromuscular blocking agents, as their administration may increase the risk of severe airway compression [57,58]. Alternatively, a supraglottic airway may provide sufficient ventilation [59]. If tracheal or bronchial collapse occurs, rigid bronchoscopy may be a useful option [46]. In general, when a compressed or distorted airway is a concern during anesthesia, a helium–oxygen gas mixture may be used as it allows for laminar air flow and minimizes resistance to gas flow in the airways [59,60].
If patients are at high risk of intraoperative airway obstruction, presurgical treatment of the mediastinal mass with steroids, chemotherapy, and/or radiotherapy may be helpful [52]. However, this approach may lessen the accuracy of diagnoses drawn from biopsies. Therefore, many clinicians recommend that, except in extreme circumstances, tissue diagnoses should be obtained prior to treatment, even if general anesthesia is required [61]. In patients with severe airway narrowing and pulmonary artery involvement, cardiopulmonary bypass may facilitate intraoperative gas exchange.
Management of the Recognized and Unrecognized Difficult Airway
The difficult airway is typically defined by the inability to provide adequate mask ventilation and/or difficulty with tracheal intubation when using a traditional laryngoscope. The incidence of difficult airways is common in infants, particularly under the age of one year [62]. Therefore, it is prudent to have preplanned protocols in place to approach both the recognized and unrecognized difficult airway situations.
The Anticipated Difficult Airway
The anticipated difficult airway scenario commonly arises in patients with craniofacial syndromes. Thus, it is imperative to perform a thorough preoperative assessment to pinpoint the area of obstruction and help gauge the success of various strategies to achieve adequate oxygenation. A useful approach to predict areas of obstruction is to group syndromes according to the associated functional abnormality [63]. Table 14.2 lists several encountered syndromes with abnormal airways grouped as such, and suggests airway management strategies.
Functional abnormality | Craniofacial syndrome | Physical features contributing to difficult airway | Possible airway management strategies |
---|---|---|---|
Subglottic abnormality | Crouzon, Apert, Pfeiffer Syndromes [65–67] | Facial/maxillary hypoplasia; prematurely fused cranial sutures | Oro- or nasopharyngeal airway; SGA; CPAP; consider indirect laryngoscopy in patients who have undergone previous corrective surgery or who have a rigid external distraction device |
Pierre Robin sequence [68] | Mandibular hypoplasia; relative macroglossia/glossoptosis; ± cleft palate | Prone positioning; video laryngoscopy; lightwand, paraglossal approach with gum-elastic bougie; SGA to overcome upper airway obstruction; SGA-assisted flexible fiberoptic intubation | |
Treacher Collins syndrome [69,70] | Mandibular, maxillary, and zygomatic hypoplasia; small mouth; temporomandibular joint abnormalities | Video laryngoscopy; SGA to overcome upper airway obstruction; SGA-assisted fiberoptic intubation | |
Hemifacial/bilateral facial microsomia/ Goldenhar’s syndrome [70–73] | Mandibular, maxillary, and malar hypoplasia; facial asymmetry; cleft-like extension on affected sides(s) of the face Goldenhar’s syndrome: cervical spine defects | SGA to overcome upper airway obstruction; SGA-assisted fiberoptic intubation, videolaryngoscopy; lightwand | |
Abnormality of the entire airway, including the glottis | Mucopolysacchariodoses [68,74–77] | Mucopolysaccharide deposits causing macroglossia, thickened oropharyngeal, laryngeal, and nasal mucosae. Restricted temporomandibular joint mobility, narrow trachea | Avoidance of neuromuscular blocking agents until airway is secured; SGA; SGA-assisted flexible fiberoptic intubation; elective tracheostomy; surgical airway backup |
Subglottic abnormality | Down syndrome [68, 78–80] | Subglottic stenosis, macroglossia, hypotonia, atlantoaxial instability | Straight blade; video laryngoscopy; use of smaller diameter TTs than calculated; neutral neck position |
Larsen syndrome [81,82] | Subglottic stenosis; laryngotracheomalacia; short neck; cephalad larynx; cervical spine instability | Use of smaller diameter TTs than calculated; neutral neck position | |
Fraser syndrome | Subglottic stenosis, webbing, or atresia (may make intubation impossible) | Use of smaller diameter TTs than calculated; SGA as primary means of ventilation |
Management of tracheal intubation poses a problem in infants and children as it is often impractical to perform awake intubations due to lack of cooperation [7]. Therefore, the clinician must consider whether or not intubation is safe to perform after induction of general anesthesia. Additionally, the feasibility of direct laryngoscopy is important to consider so that alternative devices can be readily available. Generally, direct laryngoscopy is difficult in these populations, particularly in patients with limited mouth opening [83]. If intubation is deemed unsafe to perform, alternative methods of achieving adequate oxygenation such as mask ventilation or use of a supraglottic airway (SGA) should be considered. It should be noted, however, that functional airway obstructions increase difficulty of mask ventilation [84] due to poor mask seal or supraglottic obstruction [83]. Supraglottic airways can often overcome these difficulties [83]. However, awake intubation may be the safest option in the case of difficult mask ventilation, severe upper airway obstruction, or risk of regurgitation and aspiration of gastric contents. It has been shown that placement of SGAs in the awake state may be useful in infants with craniofacial syndromes that exhibit upper airway obstructions [85,86].
Neuromuscular blocking drugs (NMBDs) help support tracheal intubation and also mitigate the risk of adverse events such as reflex airway activation [84]. They are not appropriate for every intubation scenario [65,87], and their use should be guided by the underlying airway pathology, expected ability to perform mask ventilation, and whether or not native muscle tone is needed to keep the airway patent (i.e., anterior mediastinal mass) [83]. Additionally, evidence is unclear on the use of NMBDs versus maintenance of spontaneous ventilation in managing the difficult pediatric airway [83]. In typical clinical practice, however, the difficult pediatric airway is managed after anesthetic induction and with maintenance of spontaneous ventilation.
Devices to Aid in Difficult Airway Management
The difficult airway can be successfully managed with the aid of a number of available devices. These devices include the flexible fiberoptic bronchoscope, indirect laryngoscopes, and SGAs.
The flexible fiberoptic bronchoscope is the “gold standard” in navigating a difficult tracheal intubation [7] and is available in an ultra-thin 2.2 mm external diameter size that is suitable for the newborn airway [87]. Video and optical layngoscopes are a more recently available option to guide tracheal intubation when direct laryngoscopy is not feasible. A wide variety of designs are available, and all combine a blade with a video camera or fiberoptic bundle to facilitate laryngeal visualization for oral and/or nasotracheal intubation. Also, if mouth opening permits, an SGA may be used as a primary [64–65] or temporary means to secure the airway. These devices do not require optimal anatomic positioning to achieve adequate ventilation [88]. However, when used as a means to achieve tracheal intubation, the assistance of visualization techniques as opposed to blind intubation may prove more successful [89].
Table 14.3 highlights the strengths and limitations of using these devices to manage the difficult airway.
Table 14.3 Devices to aid in difficult airway management of the infant and neonate
Device | Strengths | Limitations |
---|---|---|
Flexible fiberoptic bronchoscope [7,68,83,87, 90–94] | • Allows for tracheal intubation via oral or nasal route or through an SGA • Useful in patients with limited mouth opening • Permits lower airway examination • Assists in positioning bronchial blockers • Most sizes have a suction port to remove secretions in the airway • Working channel allows for delivery of local anesthesia | • Difficult to manipulate, particularly the ultra-thin bronchoscope (<3.0 mm OD) • Ultra-thin bronchoscope lacks a suction port • Image may be obscured by blood and secretions in the airway • Steep learning curve, regular practice needed to maintain proficiency • Higher cost, delicate, and costly to repair • Time intensive to setup and clean up |
Indirect laryngoscope [68,95–104] GlideScopeTM Stortz DCITM Truview PCDTM AirtraqTM Pentax Airway Scope (AWS)TM Optical stylets: Bonfils Intubation Stylet and Shikani Optical Stylet | • Less head and neck movement required vs. direct laryngoscopy • Improved glottic views compared with direct laryngoscopy • Laryngeal inlet may be visualized without alignment of the oral, pharyngeal, and laryngeal axis • LEDs of the GlideScope and Pentax AWS prevent fogging by providing heat • Most devices have a quick learning curve | • Airway visualization may be obscured due to blood and secretions • Difficult to manipulate TT through the glottis • Risk of failure with altered neck anatomy associated with a mass, radiation changes, or a surgical scar • Evidence for the efficacy of some devices in managing the difficult infant airway is lacking |
Supraglottic airway devices [7,64, 68,89, 105, 106, 107–112] Air-QTM Classic LMATM Supreme LMATM Proseal LMATM i-gelTM | • Useful alternative when mask ventilation is difficult • Functions as a conduit for tracheal intubation • Adequate and efficient ventilation: does not rely on optimal device positioning even in the difficult airway • Devices with gastric drain tubes are available to help protect against regurgitation of gastric contents • air-Q can accommodate cuffed TTs | • Two-step process when used as a conduit for intubation • Classic LMA may produce delayed airway obstruction in neonates • Narrow lumen of LMA Supreme restricts TT insertion and fiberoptic intubation • Narrower lumen of LMA ProSeal restricts TT insertion |
LMA, laryngeal mask airway; TT, tracheal tube: LED, light-emitting diode.
The Unanticipated Difficult Airway
The unanticipated difficult airway often becomes known after anesthetic induction. A preplanned structured approach facilitates successful navigation of the situation, though management should ultimately be guided by the patient’s condition and available resources.
Achieving adequate mask ventilation alone may be difficult. In this scenario, consider recruiting help and attempting a two-handed bag-mask technique. Alternatively, the SGA is a useful rescue device when mask ventilation or intubation are unsuccessful [113].
Difficult intubation may also be an issue. If direct laryngoscopy proves impractical, methods of indirect visualization are useful in increasing the frequency of successful intubation and successful first attempt intubation [113]. Additionally, an SGA may be used as a conduit for tracheal intubation [113]. However, care should be taken to avoid an excessive number of intubation attempts as even gentle instruments may cause iatrogenic injury to the fragile infant airway [83]. When these methods fail to adequately ventilate and oxygenate the patient, a surgical airway may be a necessary. Figure 14.1 is a proposed algorithm for management of the unanticipated difficult airway in the child.
Figure 14.1 A proposed algorithm for management of the unanticipated difficult airway in the child.
Cannot Intubate, Cannot Ventilate
The “cannot intubate, cannot ventilate” situation can be a very stressful scenario to encounter, particularly because there are limited rescue options for infants. Both surgical tracheostomy and percutaneous cricothyroid puncture are invasive and high-risk [83]. Ideally, the assistance of an otolaryngologist should be solicited for creating a surgical airway [83]. However, if help is not close at hand, the more efficient, but risky, method for invasive tracheal access is needle cricothyrotomy [83,114]. This procedure has limited efficacy in infants [83,115]. This is due in part to their proportionately smaller cricothyroid membrane, which restricts the size of the transtracheal catheter that may be used for oxygenation [83,116]. There is also a high incidence of complications associated with this procedure, such as posterior tracheal wall puncture, and esophageal puncture [114,117].
If needle cricothyrotomy is necessary, procedure-specific equipment such as the Ventilation-Catheter (VBM, Medizintechnik GmBH, Sula and Neckar, Germany) may be used with either bag or jet ventilation [114]. In the resource-limited setting, a makeshift device composed of a large-bore intravenous catheter, a syringe, and 3.0 mm internal diameter tracheal tube will suffice with bag ventilation [114]. The Enk Oxygen Flow ModulatorTM (Cook Medical, Bloomington, IN, USA) is a lower-pressure jet ventilation system that can provide adequate oxygenation utilizing standard wall oxygen, with a lower risk of barotrauma [114]. However, current knowledge suggests that jet ventilation through transtracheal catheters is associated with serious complications and barotrauma [19,118], and further evidence is needed to elucidate whether or not these devices minimize pressure-related complications [119].
Management for Tongue–Lip Adhesion and Mandibular Distraction Osteogenesis
Mandibular distraction osteogenesis (MDO) and tongue–lip adhesion are common surgical interventions to relieve upper airway obstruction in infants and neonates with craniofacial deformities. A difficult airway should be anticipated in these patients.
Nasal intubation is preferred for both MDO and tongue–lip adhesion as it minimizes disturbance to the surgical field. While awake fiberoptic intubation is the most conservative approach to achieve ventilation in these patients, lack of patient cooperation may make it unfeasible. A more practical option involves mask induction followed by asleep fiberoptic intubation. Use of a nasopharyngeal airway during nasal fiberoptic intubation in small children with difficult airways may help overcome obstruction and allow the clinician to provide CPAP, analogous to using an SGA for fiberoptic intubation [120]. If an ultra-thin flexible fiberoptic bronchoscope is available, a TT may be advanced over the scope for successful intubation of the infant or neonate [121]. However, if this approach is unsuccessful, or only a larger diameter scope is available, a guidewire may be used to facilitate intubation [122]. The guidewire is threaded through the working channel of a larger diameter bronchoscope, the bronchoscope is withdrawn and an airway exchange catheter can be placed over the guidewire to allow for railroading of the TT over it.
Retrograde nasotracheal intubation is also an alternative to achieve adequate ventilation in neonates and infants [123,124]. The airway is first secured orally, typically via SGA-assisted tracheal intubation. After the SGA is removed, a smaller TT is placed into the nose and the end is exited out of the mouth. It is then telescoped into the larger TT and secured with a suture. Both TTs are pulled retrograde out of the nose and separated, leaving the larger TT trans-nasal.
It should be noted that glossoptosis may make intubation difficult in patients with Pierre Robin sequence. In this case, the tongue can be held with Magill forceps during intubation [122], or nasotracheal intubation may be performed in the prone position. Jaw thrust is another helpful maneuver to increase airway patency.
References
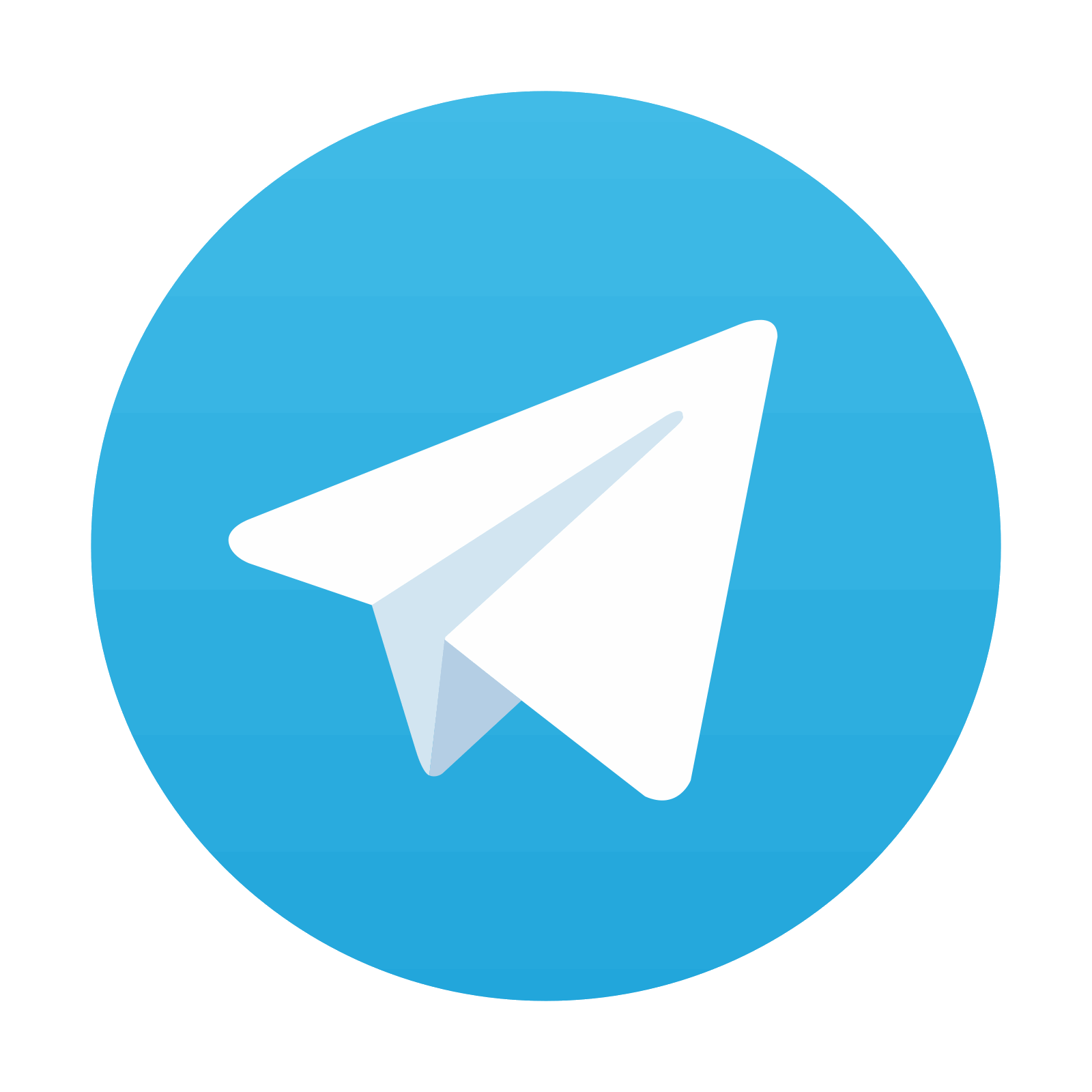
Stay updated, free articles. Join our Telegram channel
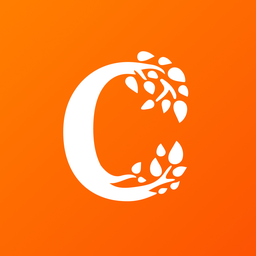
Full access? Get Clinical Tree
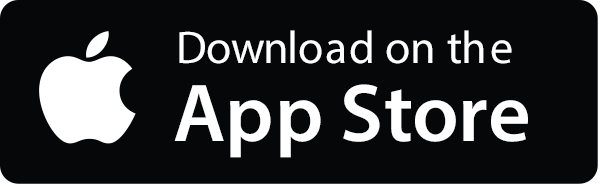
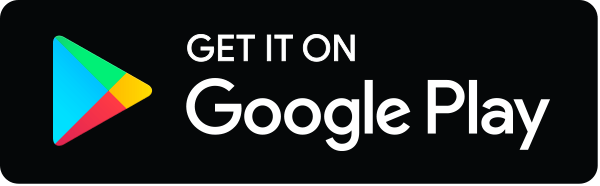
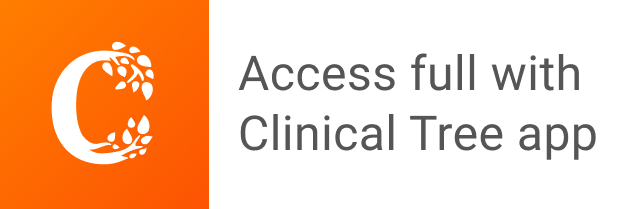