In 1516, Peter Martyr D’Anghera (1457–1526) described in his book, De Orbo Novo (The New World) the effects of poisoned arrows (proven later to contain crude curare) used by South American Indians for hunting and for fighting their enemies.1 His description clearly demonstrates the use of curare. He stated “Despite their nakedness, it must be admitted that in some places, the natives have exterminated entire groups of Spaniards, for they are ferocious and are armed with poisoned arrows.” D’Anghera then goes to give more details such as, “It was discovered that their poisoned arrows contained a kind of liquid which oozed out when the point broke.” Referring to the time course of the effect of curare, he reported, “Hojeda, under the influence of the poison, saw his strength ebbing … away” and stated that, “ .….…. the strength of the poison is such, that the mere odor of it, while compounding almost kills its makers. Whoever is wounded by one of these poisoned arrows dies, but not instantly, and no Spaniard has yet found a remedy for such wounds.”1 In 1596, Sir Walter Raleigh in his book, The Discoverie [sic] of the Large, Rich and Bewtiful Empyre [sic] of Guiana2 reported on this strong native arrow poison and referred to it as “Tupara, curare or ourari.” This poison was later found to be derived from the rubber plant Chondodendron tomentosum. During the 19th century, the paralyzing effect of curare on skeletal muscles3,4 and the antagonistic effects of physostigmine5 were studied in several animal experiments.
The clinical utility of curare was first explored in 1912 by Arthur Läwen, a German surgeon from Leipzig, who administered 0.8 mg of curarine intramuscularly to provide relaxation for intraperitoneal surgery.6 However, due to the lack of supplies of curarine, Läwen could not develop its clinical applications further. In 1935, King successfully extracted tubocurarine from crude curare and determined its chemical structure.7 In 1942, Harold Griffith and Enid Johnson were the first clinicians to use curare (Intocostrin), on some 25 patients.8
A few years later, Dr R.E. Pleasance, in his Presidential Address to the Society of Sheffield Anaesthetists on January 15, 1948, described his clinical experience using curare.9 Pleasance never mentioned the need for antagonizing the residual effects of curare in his patients. In fact, he stated that, during recovery, “there is no evidence that curare has any latent toxicity. It is completely and fairly rapidly eliminated.” It should be noted, however, that when curarization was initially introduced, tracheal intubation was the exception in routine surgical practice, and most patients undergoing anesthesia were breathing spontaneously.
It is interesting to note that the Intocostrin package insert in 1943 stated, “When dangerous respiratory embarrassment occurs, resuscitation by.….…. artificial respiration may be expected to carry the patient through the paralysis. Particularly one should be certain that an airway exists. Prostigmin [neostigmine] is also a physiologic antidote; the respiratory paralysis if not too profound is removed by this drug.” Nevertheless, no dosage for neostigmine was suggested at that time. It was not until 1948 that intravenous administration of neostigmine 1 mL of a 1:2000 dilution (0.5 mg) to treat moderate curare overdosage was suggested by E.R. Squibb and Sons. At that time, the use of neostigmine to antagonize the effects of curare on neuromuscular function was gaining momentum. In the same year, Burke and colleagues stated, in one of the early papers on the use of neostigmine for antagonism of d-tubocurarine, that “the use of neostigmine will shorten the period for the necessity of artificial respiration, but its administration should be considered as an adjuvant to treatment rather than as a substitute.”10 The recommendation for neostigmine dosages came from Prescott and colleagues in 1946.11 They stated “[I]f [P]rostigmin is to be effective, doses of the order of 5 mg or more must be used. Atropine 1.3 mg should also be given, to balance the parasympathomimetic action of [P]rostigmin.”
Inadequate recovery of neuromuscular function at the end of surgery was therefore common in the mid-1950s. It was termed neostigmine-resistant curarization12 and was attributed to mechanisms (such as depression of the acetylcholine cholinesterase system) other than the presence of a profound block induced by d-tubocurarine that could not be antagonized with neostigmine.13 Therefore, it was not surprising that the use of neuromuscular blockers in 1950s was associated with a mortality rate 6 times greater (1:370 anesthetics) than the mortality rate when neuromuscular blockers were avoided (1:2100 anesthetics).14 Furthermore, 63% of deaths that involved the use of a neuromuscular blocker were caused by respiratory failure. To date, perioperative management of neuromuscular blocking drugs remains suboptimal, and significant patient weakness in the recovery room associated with the residual effects of neuromuscular blocking drugs still occurs.15
The current consensus is that a train-of-four (TOF) ratio of at least 0.9 should be attained prior to tracheal extubation. At this level of recovery, esophageal tone, pharyngeal coordination,16 hypoxic ventilatory drive,17 and muscle strength18 appear to return toward baseline. A TOF ratio of less than 0.9 in unanesthetized volunteers has been associated with difficulty in speaking and swallowing as well as with visual disturbances.18
Postanesthetic morbidity in the form of incomplete reversal and residual postoperative weakness (also known as “residual paralysis” or “residual curarization”) is a frequent occurrence. A recent survey indicated that anesthesia providers have very different opinions about the best way to clinically demonstrate adequate recovery from neuromuscular block, and more than 75% of the respondents from both the United States and Europe believed that postoperative residual weakness was a significant anesthetic complication.15 A 45% incidence of postoperative residual neuromuscular blockade in patients arriving in the postanesthesia care unit was reported in 2003.19 This incidence is significantly higher following the use of long-acting (~70%–74%) compared to intermediate-acting (~35%–54%) neuromuscular blocking drugs.20 In addition, the incidence of critical respiratory events in the postoperative care unit remained a significant 0.8%.21 Thus, it is possible that as many as 112,000 patients annually in the United States are at risk for adverse events associated with undetected residual neuromuscular blockade.22 Monitoring the effects of neuromuscular blockers ensures their appropriate intraoperative use, effective antagonism, and prevention of residual neuromuscular weakness.
Synaptic plasticity is the “ability of individual synaptic junctions to respond [to change in strength in response] to either use or disuse.”23 The plasticity of neuromuscular junction is dependent on a highly orchestrated mechanism involving the (1) synthesis, storage, and release of acetylcholine from motor nerve endings (presynaptic region) at the neuromuscular junction; (2) binding of acetylcholine to nicotinic receptors on the muscle membrane (postsynaptic region) and generation of action potentials; and (3) rapid hydrolysis of acetylcholine by the enzyme acetylcholinesterase, which is present in the synaptic cleft.24
At the neuromuscular junction, the acetylcholinesterase enzyme is responsible for the rapid hydrolysis of released acetylcholine, thereby controlling the duration of receptor activation.25 Approximately 50% of the released acetylcholine is hydrolyzed during its diffusion across the synaptic cleft, before reaching nicotinic acetylcholine receptors located on the postsynaptic muscle membrane. The efficiency of acetylcholinesterase depends on its unusually high catalytic activity, which is one of the highest known.25 The active site of acetylcholinesterase lies near the bottom of a deep and narrow gorge that reaches halfway into the protein (Figure 10–1).26 A second anionic (peripheral) site is located at the top of the active site gorge and is probably involved in electrostatic interactions with acetylcholine.26,27 Acetylcholine must enter this cleft in the enzyme to the active site.28
Figure 10–1
The enzyme acetylcholinesterase (AChE). The active catalytic site (lined with hydrophobic amino acid side chains) lies near the bottom of a deep and narrow cleft (gorge). Acetylcholine (ACh) must enter this cleft in the enzyme, which is blocked by a mobile ring of molecules more than 97% of the time. Molecular dynamics simulations showed that the entrance to the cleft opens and shuts so frequently that any ACh molecules lingering nearby may have ample chances to diffuse in.28 AChE promotes hydrolysis of ACh by forming an acetyl-AChE intermediate with the release of choline and then hydrolysis of the intermediate to release acetate. This reaction is antagonized by AChE inhibitors such as neostigmine, edrophonium, and pyridostigmine, thereby increasing the concentration of ACh.

Acetylcholinesterase is highly concentrated at the neuromuscular junction but is also present in a lower concentration throughout the length of muscle fiber membrane.29 The distribution of acetylcholinesterase molecules at the neuromuscular junction closely matches the distribution of nicotinic acetylcholine receptors.30
In addition to acetylcholinesterase, butyrylcholinesterase is also present at the neuromuscular junction at low concentrations.31 Butyrylcholinesterase is synthesized by the liver and is found in the plasma and is responsible for metabolism of succinylcholine, mivacurium, procaine, chloroprocaine, tetracaine, cocaine, and heroin. The exact function of butyrylcholinesterase at the neuromuscular junction is not known, but there is evidence that butyrylcholinesterase may act as a poison scavenger (eg, cocaine poisoning), protecting the integrity of acetylcholinesterase.32
Enzymatic inhibition is an indirect mechanism for antagonizing the residual effects of neuromuscular blockers; it affects neither the rate of elimination of the neuromuscular blocker from the body, nor the plasma or tissue (biophase) concentration of the neuromuscular blocking agent. Acetylcholinesterase inhibitors (eg, neostigmine and, less commonly, edrophonium and pyridostigmine) are used clinically to antagonize the residual effects of neuromuscular blockers and to accelerate recovery from nondepolarizing neuromuscular blockade. This antagonism results in a decrease in the rate of acetylcholine hydrolysis and in increase in acetylcholine concentrations. The increased amount of acetylcholine competes with the residual unbound (free) molecules of the neuromuscular blocking drug for the available unoccupied nicotinic acetylcholine receptors at the neuromuscular junction. This mechanism of antagonism has a ceiling effect.33,34 Once the inhibition of acetylcholinesterase is maximal, administering additional doses of an acetylcholinesterase inhibitor (eg, neostigmine) will serve no useful purpose. If neostigmine is administered at a deep level of neuromuscular blockade (ie, no response to TOF stimulation), the concentration of neuromuscular agent will be high at the neuromuscular junction; even with full cholinesterase antagonism, the amount of free acetylcholine at the junction will be insufficient to effectively compete with the neuromuscular agent. Indeed, administering additional neostigmine at this point may in fact worsen neuromuscular recovery.35 This underscores the limitations of neostigmine (or any other acetylcholinesterase inhibitor) in clinical practice and explains, in part, the high incidence of postoperative residual neuromuscular blockade.20
The pharmacokinetic parameters of the acetylcholinesterase inhibitors are listed in Table 10–1.36,37,38, and 39 The elimination half-life of edrophonium is similar to that of neostigmine and pyridostigmine,38 although the duration of action of pyridostigmine is somewhat longer.36,37 Renal excretion accounts for about 50% of the elimination of neostigmine and about 75% of that of pyridostigmine and edrophonium. Renal failure decreases the plasma clearance of neostigmine, pyridostigmine, and edrophonium as much as, if not more than, that of the long-acting neuromuscular blockers.
Encapsulation of free molecules of rocuronium and vecuronium (steroidal-type muscle relaxant agents) represents an innovative approach for antagonizing the effects of neuromuscular blockers. Sugammadex is a modified γ-cyclodextrin (Figure 10–2).40,41,42, and 43 Cyclodextrins are cyclic dextrose units joined through 1 to 4 glycosyl bonds that are produced from starch or starch derivatives using cyclodextrin glycosyltransferase. The 3 natural unmodified cyclodextrins consist of 6-, 7-, and 8-cyclic oligosaccharides and are called α-, β-, and γ-cyclodextrin, respectively. Their 3-dimensional structures, which resemble a hollow, truncated cone or a doughnut, have a hydrophobic cavity and a hydrophilic exterior because of the presence of polar hydroxyl groups. Hydrophobic interactions trap the drug into the cyclodextrin cavity (the doughnut hole), resulting in formation of a water-soluble guest–host complex.
Compared with α- and β-cyclodextrins, γ-cyclodextrin exhibits more favorable properties with regard to steroidal relaxant interaction in terms of the size of its internal cavity, water solubility, and bioavailability. This is because the α- and β-cyclodextrins have smaller lipophilic cavities (diameter < 0.65 nm) and form less stable complexes with the bulky aminosteroid neuromuscular blocker molecule (eg, rocuronium or vecuronium; molecular width, ~ 0.75 nm). In contrast, the γ-cyclodextrin molecule has a larger lipophilic cavity (0.75–0.83 nm in diameter).40
To improve the fit of the larger, rigid structure of the aminosteroid neuromuscular blocker molecule within the cavity of γ-cyclodextrin, the sugammadex ring was modified by adding 8 side chains to extend the cavity. This modification allowed the 4 hydrophobic steroidal rings of rocuronium to be better accommodated within the hydrophobic cavity. Addition of negatively charged carboxyl groups at the end of each of the 8 side chains serves 2 purposes. First, the repellent forces of the negative charges keep propionic acid side chains from being disordered, thereby allowing the cavity to remain open until encapsulation. Second, these negatively charged carboxyl groups enhance the electrostatic binding to the positively charged quaternary nitrogen of rocuronium, rendering the complexation irreversible (see Figure 10–2).40,41
These modifications resulted in sugammadex, a compound that is highly water soluble and that contains a hydrophobic cavity large enough to encapsulate steroidal neuromuscular blocking drugs, especially rocuronium.40,41,42, and 43 The aqueous solution of sugammadex has a pH of approximately 7.5 and osmolality of 300 to 500 mOsm/kg. Sugammadex exerts its effect by forming very tight complexes in a 1:1 ratio with steroidal neuromuscular blocking agents (rocuronium > vecuronium >> pancuronium).40,41,42, and 43 The intermolecular (van der Waals) forces, thermodynamic (hydrogen) bonds, and hydrophobic interactions of the sugammadex–rocuronium complex make it very tight.40 The sugammadex–rocuronium complex has a very high association rate and a very low dissociation rate. Estimates are that for every 30 million sugammadex–rocuronium complexes, only one complex dissociates.
Sugammadex is biologically inactive.44,45, and 46 When administered by itself to volunteers who had not received a neuromuscular blocking agent, doses of 0.1 to 8.0 mg/kg of sugammadex had a clearance rate of 120 mL/min, an elimination half-life of 100 minutes, and a volume of distribution of 18 L.45 Approximately 75% of the sugammadex dose was eliminated through the urine. The clearance of sugammadex/rocuronium complex is 109 mL/min.47 Between 59% and 80% of total dose of sugammadex is excreted in the urine in the first 24 hours after administration.45 The kinetics of sugammadex appear to be dose dependent, in that clearance increased and elimination half-life decreased, when the sugammadex dose was increased from 0.15 to 1.0 mg/kg.45 The clearance of sugammadex decreases with advancing age, and this reduction is correlated with reduced creatinine clearance seen in the elderly.47 In elderly patients (age > 75 year), sugammadex clearance is decreased by 50% compared with adult (18–64 year) patients (52 versus 103 mL/min) and by approximately 30% compared with older (65–74 year) patients (52 versus 76 mL/min).47
In the absence of sugammadex, rocuronium is eliminated mainly by biliary excretion (> 75%) and to a lesser degree by renal excretion (10%–25%). The plasma clearance of sugammadex alone is approximately three times lower than that of rocuronium alone.48 In volunteers, the plasma clearance of rocuronium was decreased by a factor of greater than 2 after administration of a dose of sugammadex of equal or greater than 2.0 mg/kg.45 This prolongation is due to the biliary route of excretion, which becomes unavailable for the rocuronium–sugammadex complex, and rocuronium clearance decreases to a value approaching the glomerular filtration rate (120 mL/min). As noted earlier, after administration of sugammadex, the plasma concentration of free rocuronium decreases rapidly, but the total plasma concentration of rocuronium (both free and bound to sugammadex) increases due to redistribution of free rocuronium from the peripheral compartments to the plasma (Figure 10–3).47,49
Figure 10–3
Relationship between rocuronium plasma concentration and twitch height. Rocuronium-induced block was reversed while the rocuronium infusion was ongoing. Note the increase in the total plasma concentration of rocuronium (free and complexed) and recovery of twitch height, even though the infusion rate of rocuronium was maintained. (Reproduced with permission from Epemolu O, Bom A, Hope F, Mason R: Reversal of neuromuscular blockade and simultaneous increase in plasma rocuronium concentration after the intravenous infusion of the novel reversal agent Org 25969. Anesthesiology 2003;Sep;99(3):632-637.)

The soluble nature of the sugammadex–rocuronium complex results in urinary excretion of the complex as the major route of elimination of rocuronium (65%–97% of the administered dose).45,48 Excretion is rapid, with approximately 70% of the dose being excreted within 6 hours and more than 90% within 24 hours. Renal excretion of rocuronium is increased by more than 100% after administration of 4 to 8 mg/kg of sugammadex.48
Sugammadex does not bind to human plasma proteins and erythrocytes to a significant extent. Metabolism of sugammadex is at most very limited, and the drug is predominantly eliminated unchanged by the kidneys. In patients with substantial renal impairment, clearance of sugammadex and rocuronium decreased by factors of 16 and 3.7, respectively, relative to clearance in healthy subjects, and the elimination half-lives were increased by factors of 15 and 2.5, respectively. The effectiveness of dialysis in removing sugammadex and rocuronium from plasma has not been demonstrated consistently. Therefore, sugammadex administration should be avoided in patients with a creatinine clearance less than 30 mL/min.
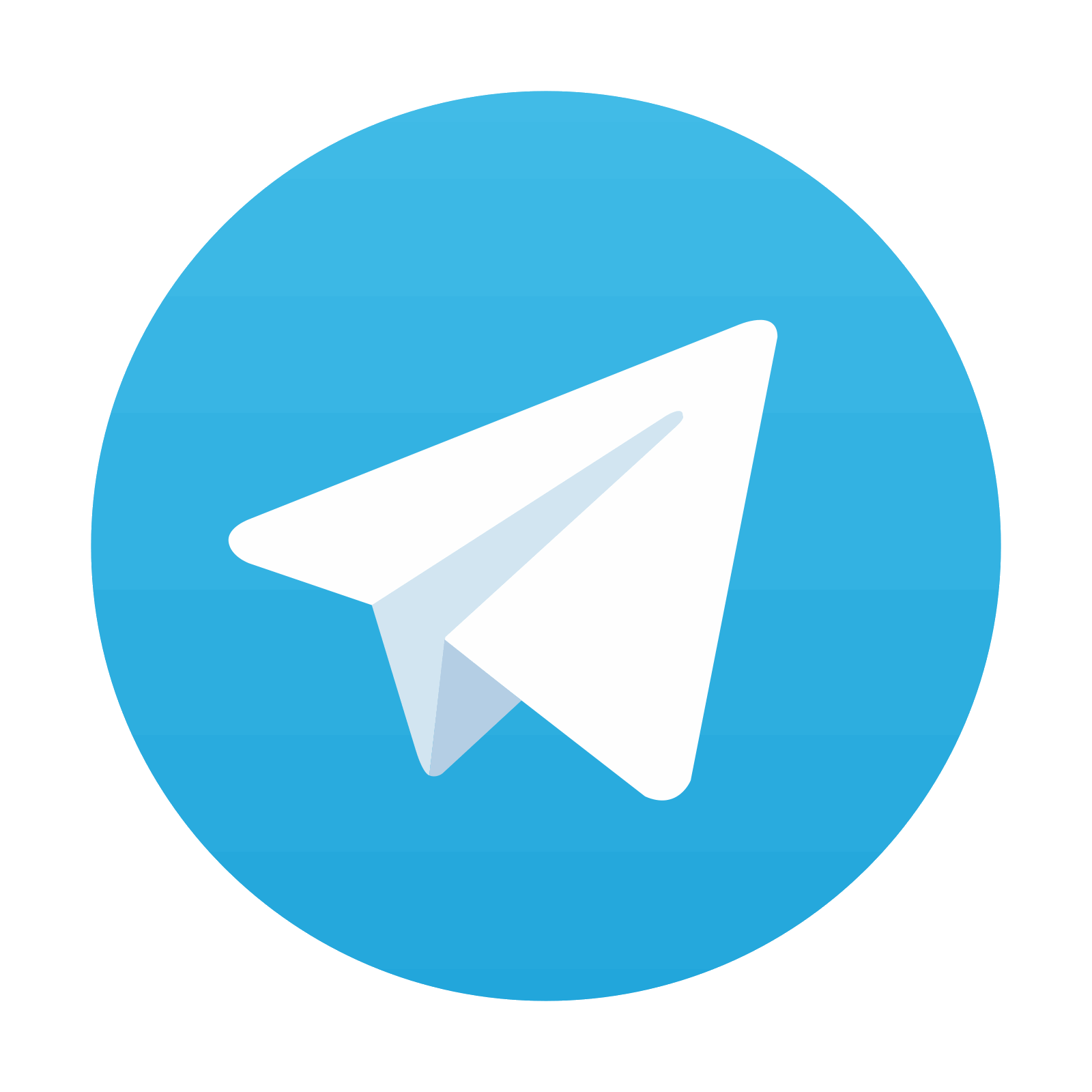
Stay updated, free articles. Join our Telegram channel
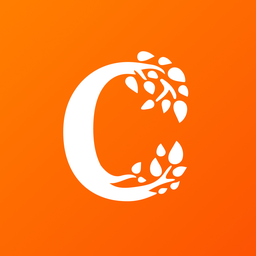
Full access? Get Clinical Tree
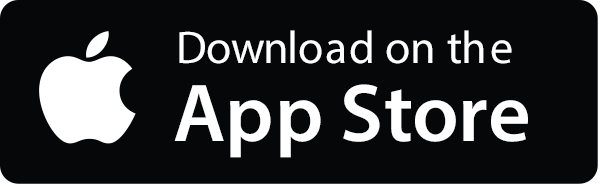
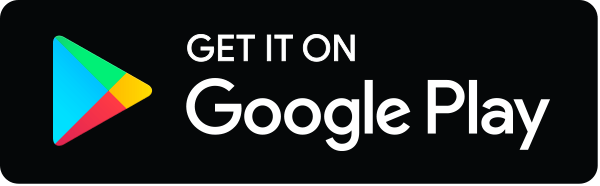