Respiratory Failure Part VI: Acute Respiratory Failure in Pregnancy
Christine Campbell-Reardon
Helen M. Hollingsworth
The overall pregnancy-related maternal mortality ratio in the United States during 1991 to 1999 was 11.8 deaths per 100,000 live births [1]. Acute respiratory failure remains an important cause of maternal and fetal morbidity and mortality. Thromboembolism, amniotic fluid embolism (AFE), and venous air embolism together account for approximately 20% of maternal deaths [2], and other causes of respiratory failure probably account for another 11% [1].
This chapter focuses on the causes of acute respiratory failure that are increased in frequency during pregnancy, are unique to pregnancy, or present special management requirements during pregnancy. The spectrum of problems associated with eclampsia is discussed in Chapter 156. Management of the acute respiratory distress syndrome (ARDS) caused by sepsis, trauma, or other etiologies unrelated to pregnancy is discussed in Chapter 47. Table 51.1 lists causes of acute respiratory failure in pregnancy.
Normal Alterations in Cardiopulmonary Physiology During Pregnancy
Pregnancy alters respiratory physiology by causing changes in lung volumes, mechanics of ventilation, and control of respiration. Despite mucosal changes to the airway of edema and hyperemia, spirometry studies reveal no significant changes in measurements of the forced expiratory volume in 1 second (FEV1) during pregnancy, suggesting that airway function is maintained during pregnancy. Changes in lung volume associated with gestation are relatively small: total lung capacity decreases 4% to 6%, functional residual capacity (FRC) decreases approximately 15% to 25%, and residual volume remains constant. Despite the decrease in FRC, early airway closure has not been demonstrated and specific airway conductance remains constant [3]. Diffusing capacity is elevated in the first trimester but then declines, despite continued increases in cardiac output and plasma volume.
As gestation progresses, the resting level of the diaphragm rises, but diaphragmatic excursion with tidal breathing increases. An increased tidal volume (25% to 35%) accounts for much of the 20% to 40% increase in minute ventilation and the mild respiratory alkalosis that are characteristic of early-to-middle pregnancy. An increased respiratory rate also contributes to the increased minute ventilation late in pregnancy (Fig. 51.1).
Normal carbon dioxide tension (PaCO2) during pregnancy is 27 to 34 mm Hg, suggesting chronic mild hyperventilation. The degree of hyperventilation has been found to be in excess of the amount needed to compensate for increased oxygen consumption; in fact, hyperventilation develops early in gestation, before any significant increase in oxygen consumption occurs. This has been attributed to elevation in levels of progesterone, which has a known respiratory stimulating effect. The exact mechanism by which it produces this effect is not known, but it is thought to include an increase in the central chemoreflex drive to breathe and to changes in acid–base balance such that central and plasma hydrogen ion concentration is increased for any given PCO2. In addition, pregnancy is associated with increased sensitivity to CO2 as measured by CO2 ventilatory response curves, reflecting the new, lower set point in PaCO2, possibly mediated by estrogen and progesterone. The respiratory alkalosis seen during pregnancy causes a compensatory renal excretion of bicarbonate to maintain an arterial pH between 7.40 and 7.45.
The normal arterial oxygen tension (PaO2) in pregnant women ranges from 100 to 110 mm Hg. Oxygen consumption increases by 20% to 33% by the third trimester, secondary to both fetal and maternal demands. This increased rate of oxygen consumption and low oxygen reserve secondary to a reduced FRC place pregnant patients at risk for the rapid onset of hypoxia.
Circulatory changes occur during gestation to supply oxygen-rich blood to the placenta and to accommodate the stress of labor and delivery. Cardiac output begins to rise in the first trimester and peaks around the 20th week of gestation at 30% to 45% above resting, nonpregnant levels (Fig. 51.2). Thus, measured cardiac output during gestation that is in the normal range for a nonpregnant patient would represent significant hemodynamic compromise for the pregnant patient and, potentially, decreased oxygen delivery for the fetus. As pregnancy progresses, cardiac output becomes dependent on body position. In the supine position, cardiac output can be reduced by 25% to 30% due to compression of the inferior vena cava by the gravid uterus and a resultant decrease in venous return. Cardiac output is higher when the pregnant woman is in the left lateral decubitus position. Estimates of expected cardiac output during gestation should be revised upward for intercurrent stresses such as fever, infection, and pain.
The gestation-related increase in cardiac output reflects a combination of increases in heart rate and stroke volume. Heart rate increases progressively throughout gestation, reaching a 20% or 15 beats per minute increase over nonpregnant levels. Stroke volume increases more rapidly at first and then stabilizes. Left ventricular compliance must increase in pregnancy because the increased stroke volume appears to be related more to left ventricular enlargement than to increased emptying. The cardiac silhouette on chest radiography may appear enlarged as a result of mild normal left ventricular enlargement and lateral and upward displacement by the gravid uterus.
Further increases in cardiac output occur during labor; cardiac output increases up to 45% over third trimester values,
and during uterine contraction, cardiac output transiently increases another 10% to 15% because of increased venous return. Another factor that may be important in patients who are sensitive to left ventricular afterload is inhibition of blood flow to the uterus during labor contractions. Because uterine blood flow at term accounts for a significant proportion of the cardiac output, marked increases in afterload during contractions and immediately postpartum may occur. During labor, contractions are associated with increased blood return from the uterus. These “autotransfusions” may reach 500 mL when the uterus contracts after parturition. This effect, however, may be offset by blood loss. In the first few minutes postpartum, cardiac output may increase as much as 80% over prelabor levels, then decrease to 40% to 50% over prelabor values by 1 hour postpartum, and finally return to nearly pre-pregnant levels by 1 to 2 weeks postpartum.
and during uterine contraction, cardiac output transiently increases another 10% to 15% because of increased venous return. Another factor that may be important in patients who are sensitive to left ventricular afterload is inhibition of blood flow to the uterus during labor contractions. Because uterine blood flow at term accounts for a significant proportion of the cardiac output, marked increases in afterload during contractions and immediately postpartum may occur. During labor, contractions are associated with increased blood return from the uterus. These “autotransfusions” may reach 500 mL when the uterus contracts after parturition. This effect, however, may be offset by blood loss. In the first few minutes postpartum, cardiac output may increase as much as 80% over prelabor levels, then decrease to 40% to 50% over prelabor values by 1 hour postpartum, and finally return to nearly pre-pregnant levels by 1 to 2 weeks postpartum.
Table 51.1 Causes of Acute Respiratory Failure in Pregnancy | ||
---|---|---|
|
Systemic vascular resistance is reduced in pregnancy due to vasodilatation and the low resistance of the uteroplacental vascular circuit. Possible factors leading to vasodilatation include a reduction in vascular responsiveness to norepinephrine and angiotensin II, increased endothelial prostacyclin production, and increased nitric oxide production. The mean blood pressure remains relatively constant despite increases in cardiac output. Pressures in the right ventricle, pulmonary artery, and pulmonary capillaries are no different from nonpregnant values.
![]() Figure 51.1. Changes in respiratory function during pregnancy. [Reprinted from Leontic EA: Respiratory disease in pregnancy. Med Clin North Am 61:111, 1977, with permission.] |
During pregnancy, there is expansion of the extracellular fluid volume, with the plasma fluid volume increasing more than the interstitial volume. Maternal blood volume reaches its peak at 32 weeks and is 25% to 52% above prepregnancy levels. The erythrocyte mass increases by 20% to 30%. However, the plasma volume increases more than the erythrocyte volume, resulting in the physiologic anemia of pregnancy.
Colloid osmotic pressure measurements during gestation reveal a mean decrease of 5 mm Hg, which reaches a plateau at 26 weeks. This parallels a decrease in serum albumin concentrations from approximately 4.0 to 3.4 g per dL. A further decline in colloid osmotic pressure of roughly 4 mm Hg occurs immediately postpartum, probably as a result of a combination of factors, such as recumbency, crystalloid administration, and blood loss. These changes may be even more marked in patients with pregnancy-induced hypertension. Neither the absolute value of colloid osmotic pressure nor the colloid osmotic pressure–pulmonary capillary wedge pressure gradient is an accurate predictor of pulmonary edema because of the multiplicity of contributing variables. However, these trends in colloid osmotic pressure should be considered when interpreting pulmonary capillary wedge pressures, especially in patients who have received large amounts of crystalloid.
Determinants of Fetal Oxygen Delivery
Oxygen delivery to fetal tissues can be affected at many levels: maternal oxygen delivery to the placenta, placental transfer, and fetal oxygen transport from the placenta to fetal tissues. The major determinants of oxygen delivery to the placenta are the oxygen content of uterine artery blood, which is determined by maternal PaO2; hemoglobin concentration and saturation; and uterine artery blood flow, which depends on maternal cardiac output. Thus, a decreased PaO2 can be offset somewhat
by increased blood hemoglobin concentration or by increased cardiac output. The combination of maternal hypoxemia and decreased cardiac output likely has a profoundly deleterious effect on fetal oxygenation.
by increased blood hemoglobin concentration or by increased cardiac output. The combination of maternal hypoxemia and decreased cardiac output likely has a profoundly deleterious effect on fetal oxygenation.
Variations in maternal pH also influence oxygen delivery. Alkalosis causes vasoconstriction of the uterine artery, resulting in decreased fetal oxygen delivery. This effect is magnified by a leftward shift in the maternal oxyhemoglobin saturation curve, which increases oxygen affinity and consequently decreases oxygen transfer to the umbilical vein. Although mild maternal acidosis does not enhance uterine blood flow because the uterine vasculature is already maximally dilated, it shifts the maternal oxyhemoglobin saturation curve to the right, which leads to decreased oxygen affinity and increased fetal oxygen delivery. Maternal hypotension and increased sympathetic stimulation (exogenous or endogenous) both cause uterine arterial vasoconstriction.
The importance of maternal cardiac output is supported by the observation that women with left ventricular outflow obstruction have an increased incidence of fetal death and surviving infants have an increased incidence of congenital heart disease. Data from a sheep model, however, suggest that a decrease in uterine blood flow up to 50% for brief periods does not appreciably decrease fetal and placental oxygen uptake. Chronically decreased maternal cardiac output may have other effects, perhaps on placental development, that explain the results in women with left ventricular outflow obstruction.
The interaction of maternal and fetal circulations in the placenta most likely follows a concurrent exchange mechanism. This is less efficient than a countercurrent exchange mechanism and partly explains why the PaO2 in the fetal umbilical vein, which carries oxygenated blood to fetus, is in the range of 32 mm Hg, far lower than uterine vein PaO2, and why increased maternal inspired oxygen increases uterine artery oxygen tension but does not cause major increases in umbilical vein PaO2. Despite low umbilical vein PaO2, fetal oxygen content is actually quite close to maternal oxygen content because of the shape of the oxyhemoglobin saturation curve for fetal hemoglobin (Fig. 51.3). This is one of the major protective mechanisms for fetal oxygenation. The fetal oxyhemoglobin saturation curve is relatively unaffected by changes in pH; although acidosis may decrease maternal oxygen affinity, fetal oxygen affinity is unchanged.
Other placental factors that determine fetal oxygenation are the amount of intraplacental shunt, degree of matching of maternal and fetal blood flows, and the presence of any placental abnormalities, such as placental infarcts. There seem to be no placental autoregulatory mechanisms that increase blood flow in response to decreased maternal PaO2.
Mathematical models predicting the optimal apportionment of fetal cardiac output between umbilical (to collect oxygen) and systemic (to deliver oxygen) circulations have yielded values surprisingly close to those measured under normal physiologic conditions. This appears to be another compensation mechanism for the apparent inefficiency (concurrent exchange mechanism) of the placenta. One disadvantage in terms of oxygen delivery to fetal tissues is that oxygenated umbilical vein blood is mixed in the fetal inferior vena cava with deoxygenated systemic venous blood before delivery to the systemic circulation. Thus, fetal arterial blood has an even lower PaO2 than umbilical vein blood. This is compensated for in part by a high fetal cardiac output relative to oxygen consumption, thus enhancing oxygen delivery to fetal tissues. The fetal circulation appears to have the ability to autoregulate in the face of hypoxemia to protect the brain, adrenal glands, and heart. How long this adaptation can be depended on safely before organ damage occurs is not known.
How well do the compensatory mechanisms that provide adequate oxygen supply to the fetus under normal conditions manage during maternal hypoxia? Calculation of oxygen stores in the term infant with 60% hemoglobin saturation yields a total oxygen content of 40 mL. Given an oxygen consumption of 6 mL per kg per minute, or approximately 18 mL per minute at term, this reserve lasts barely 2 minutes when the maternal oxygen supply is completely interrupted. The shape of the fetal oxyhemoglobin dissociation curve places umbilical vein PaO2 values below 30 mm Hg on the steep part of the curve, so small changes in maternal PaO2 may cause significant changes in fetal oxygen content. A maternal PaO2 greater than 70 mm Hg should be maintained to prevent adverse consequences to the fetus. Concern regarding the adequacy of fetal oxygen supply is further reduced if a normal maternal PaO2 of 90 mm Hg or greater is achieved without too great a risk of maternal barotrauma or oxygen toxicity. Extensive referencing supporting this section can be found in Chapter 50 of sixth edition [4].
Causes of Acute Respiratory Failure
This section describes the more common causes of acute respiratory failure in pregnancy in terms of frequency, clinical presentation, pathophysiology, and diagnosis.
Thromboembolic Disease
Fatal pulmonary embolism is a rare complication in pregnancy, but it accounts for 20% of all pregnancy-related deaths in the United States [5]. Thromboembolic complications have been estimated to occur in 0.76 to 1.72 per 1,000 pregnancies [6]. The increased frequency of thromboembolic disease in pregnancy may be attributable to a hypercoagulable state along with venous stasis. During pregnancy, there is a progressive increase in coagulation factors I, II, VII, VIII, IX, and X. There is a decrease in protein S and a progressively increased resistance to activated protein C [6]. The activity of plasminogen
activator inhibitor types 1 and 2, which are inhibitors of fibrinolysis, also increases [7]. Venous stasis may occur because of a hormonally induced dilation of capacitance veins and uterine pressure on the inferior vena cava [8]. Factors that further increase the risk of thromboembolic disease during pregnancy and the puerperium include (a) cesarean section, which has a 10 times greater risk of fatal pulmonary embolism than does vaginal delivery; (b) increased maternal age; (c) multiparity; (d) obesity, especially in association with bed rest; (e) personal or family history of thromboembolism; (f) suppression of lactation with estrogen; (g) surgical procedures during pregnancy and early puerperium; and (g) inherited thrombophilias such as deficiencies of proteins C or S, the presence of antiphospholipid antibodies, the presence of factor V Leiden, and prothrombin gene mutations [8,9,10].
activator inhibitor types 1 and 2, which are inhibitors of fibrinolysis, also increases [7]. Venous stasis may occur because of a hormonally induced dilation of capacitance veins and uterine pressure on the inferior vena cava [8]. Factors that further increase the risk of thromboembolic disease during pregnancy and the puerperium include (a) cesarean section, which has a 10 times greater risk of fatal pulmonary embolism than does vaginal delivery; (b) increased maternal age; (c) multiparity; (d) obesity, especially in association with bed rest; (e) personal or family history of thromboembolism; (f) suppression of lactation with estrogen; (g) surgical procedures during pregnancy and early puerperium; and (g) inherited thrombophilias such as deficiencies of proteins C or S, the presence of antiphospholipid antibodies, the presence of factor V Leiden, and prothrombin gene mutations [8,9,10].
The appropriate diagnostic steps and treatment of venous thrombosis and pulmonary embolism in nonpregnant patients are reviewed in Chapter 52. This chapter focuses on the diagnosis and management of massive pulmonary embolism associated with severe respiratory and hemodynamic compromise during pregnancy. Respiratory failure may ensue in pulmonary embolism when extensive occlusion of the pulmonary vasculature or concomitant pulmonary edema occurs. Pulmonary edema has been associated with pulmonary embolism in areas of intact blood flow and has been attributed to increased hydrostatic forces in nonoccluded vessels, vigorous crystalloid resuscitation, and increased microvascular permeability caused by platelet-derived mediators [11,12].
Although none of the symptoms, physical signs, or results of laboratory, radiographic, or electrocardiographic studies are specific for pulmonary embolism, these investigations can help rule out other diseases in the differential diagnosis. The usefulness of the serum D-dimer levels in diagnosing thromboembolic disease in pregnancy is limited because D-dimer levels are increased during normal pregnancy, with levels increasing as gestation progresses and peaking at delivery and in the early postpartum period [13,14]. Likewise, hemodynamic data obtained at pulmonary artery catheterization are more helpful in excluding other processes and in guiding hemodynamic management than in making a definitive diagnosis of pulmonary embolism.
The typical hemodynamic findings in nonpregnant patients with massive pulmonary embolism are delineated in Chapter 52. Although there are no data for pregnant patients with massive pulmonary embolism, similar findings would be anticipated because pregnancy does not significantly alter right heart and pulmonary artery pressures. Thus, in a pregnant patient with massive pulmonary embolism, pulmonary artery balloon occlusion pressure (i.e., pulmonary capillary wedge pressure) would be expected to be normal or low, mean pulmonary artery pressure moderately elevated (≥ 35 mm Hg), and right atrial pressure moderately elevated (> 8 mm Hg).
Doppler ultrasound of the lower extremities to assess for lower extremity deep venous thrombosis (DVT) may be chosen as the initial test in the evaluation for a pulmonary embolism. Further diagnostic evaluation is not required when a DVT is found in the legs, as the treatment for DVT and pulmonary embolism is the same. A negative Doppler ultrasound of the lower extremities does not rule out the presence of pulmonary embolism, so further diagnostic investigation is required. In the nonpregnant patient population, helical CT scanning with intravenous contrast has become the study of choice for pulmonary embolism. Data from PIOPED 2 determined the sensitivity and specificity of CT angiography for detecting pulmonary embolism to be 83% and 96%, respectively [15]. Pregnancy was an exclusion criteria in this study, so there is a lack of prospective data assessing CT angiography in pregnancy. However, CT angiography is now being used more commonly as the first screening examination for pulmonary embolism during pregnancy [16]. This trend is based on evidence that the dose of ionizing radiation from a helical CT scan is safe in all trimesters. The radiation dose to the fetus ranged from 0.00033 rad in the first trimester to 0.01308 rad in the third trimester. This radiation dose is comparable with the dose exposure during ventilation–perfusion scanning [17]. CT angiography also provides the opportunity of diagnosing other abnormalities that may be causing the patient’s symptoms even if the scan is negative for thromboembolic disease.
Ventilation–perfusion lung scanning remains a useful diagnostic test for pulmonary embolism during pregnancy in patients who have a contraindication to radiocontrast. Pulmonary angiography may still be required for definitive diagnosis of a pulmonary embolism in some patients. Fetal exposure to radiation during imaging studies can be minimized by abdominal shielding and using brachial access.
Amniotic Fluid Embolism
AFE, also known as anaphylactoid syndrome of pregnancy, is a rare, but usually catastrophic, complication of pregnancy and delivery [18,19,20]. The incidence of AFE is approximately 1 in every 8,000 to 80,000 deliveries [21,22,23]. A retrospective, population-based cohort study of 3 million birth records in the United States reported an incidence of 7.7 cases per 100,000 births [23]. The mortality rates reported in the literature have been reported to be from 22% to 86% [21,22,23]. Of the women who survive AFE, only 15% of them are neurologically intact [21].
It is unknown why amniotic fluid enters the maternal circulation in some patients, although certain potential predisposing clinical factors have been suggested based on registry and cohort studies. These factors include older maternal age (mean, 32 years), multiparity (88% of cases), amniotomy, cesarean section, abruptio placentae, insertion of intrauterine fetal or pressure monitoring devices, and term pregnancy in the presence of an intrauterine device [23]. Amniotic fluid enters the maternal circulation through one of three ports: endocervical veins; uterine tears (small tears may occur in the lower uterine segment as a part of normal labor); and uterine injury secondary to iatrogenic manipulation, such as cesarean section, insertion of monitoring devices, or membrane rupture [18].
The two life-threatening consequences of AFE are cardiopulmonary collapse and disseminated intravascular coagulation (DIC). These may occur simultaneously or in sequence. The pathophysiologic process of cardiopulmonary collapse remains controversial. It is possible that amniotic fluid contains vasoactive substances or fetal antigens that provoke an abnormal hemodynamic and immunologic response in the mother that results in the AFE syndrome [24]. There may be a biphasic response to AFE with initial hypoxemia and acute pulmonary hypertension, followed by left ventricular failure. Elevation of the pulmonary balloon occlusion pressure and reduction in cardiac output and left ventricular stroke work index have been documented [25,26,27].
Although Morgan [28] described only a 24% incidence of pulmonary edema, an autopsy review demonstrated that most lungs exhibited pulmonary edema (10% severe, 60% moderate) [29]. Most cases are rapidly fatal, so radiographs have been infrequently obtained, which may explain the low incidence of pulmonary edema reported by Morgan [28]. The cause of pulmonary edema has variably been ascribed to vigorous fluid resuscitation, increased permeability pulmonary edema, and cardiac decompensation caused by hypoxia and tachycardia [26].
The other major consequence of AFE is coagulation failure. In 10% to 15% of patients, excessive bleeding, particularly uterine bleeding, may be the first sign of AFE. Up to 50% of
patients who survive the first 30 to 60 minutes have clinical evidence of coagulopathy, and most of the remaining patients have laboratory evidence of DIC [28]. The initiating factors precipitating DIC are not known.
patients who survive the first 30 to 60 minutes have clinical evidence of coagulopathy, and most of the remaining patients have laboratory evidence of DIC [28]. The initiating factors precipitating DIC are not known.
The abrupt onset of severe dyspnea, tachypnea, and cyanosis during labor or the early puerperium is the classic presentation of AFE, characterizing more than one half of cases. Shock, which is out of proportion to blood loss, is the first manifestation in another 10% to 15%. Seizure activity may be the presenting sign in 30% of cases. In addition, fetal bradycardia is seen in 17% of U.S. registry cases. Bleeding is the forerunning sign in 10% to 15% of patients, and the longer the survival, the greater the likelihood that the patient will manifest respiratory failure, cardiovascular collapse, and DIC. Whatever the presenting symptom complex, 90% of cases occur before or during labor [28]. Other complications, such as acute renal failure and signs of central nervous system injury, are probably secondary to hypotension and hypoxemia. Prodromal symptoms, such as vomiting and shivering, are nonspecific and frequently associated with otherwise uneventful deliveries.
Diagnostic criteria for AFE previously rested on demonstration of fetal elements such as epithelial squamous cells from fetal skin, lanugo hairs, fat from the vernix caseosa, mucin from fetal gut, and bile-containing meconium in the maternal circulation. These elements are not pathognomonic for AFE, as these amniotic fluid components are found in the maternal circulation of healthy pregnant women without AFE [30,31]. Therefore, the antemortem diagnosis of AFE still rests predominantly on the clinical setting and the exclusion of other causes of acute respiratory failure. The role of echocardiography in the diagnosis of AFE is not yet known. Cardiac echo may show decreased left ventricular function or echodense material in the right atrium or right ventricular outflow tract [32]. A serologic assay has been developed using a monoclonal antibody TKH-2 to detect a meconium and amniotic fluid-derived mucin-type glycoprotein. This assay is reported to have a high sensitivity for detecting AFE, but it is not yet recommended for routine clinical practice [33,34].
Fetal outcome is also poor in AFE. The perinatal mortality from the national registry was 21%, with 50% of the survivors experiencing permanent neurologic injury [21].
Venous Air Embolism
Venous air embolism has been described during normal labor, delivery of patients with placenta previa, criminal abortions using air, and insufflation of the vagina during gynecologic procedures [35,36]. There are also cases reported in the literature of venous embolism occurring following orogenital sex and after the use of a birth training device designed to stretch the peritoneum to prevent perineal injury by inflating and deflating a balloon [37,38]. Venous air embolism may account for as many as 1% of maternal deaths [35]. Presumably, the subplacental venous sinuses are the sites of air entry when antepartum or peripartum air embolism occurs [35].
Sudden, profound hypotension is the most common presenting sign of venous air embolism. Cough, dyspnea, dizziness, tachypnea, tachycardia, and diaphoresis also may be noted. Hypotension is usually followed quickly by respiratory arrest. The classic sign associated with air embolism is the mill wheel murmur, which is audible over the precordium [39]; a drumlike or bubbling sound may also be heard. Electrocardiographic evidence of ischemia, right heart strain, and arrhythmias have been described, and metabolic acidosis, presumably caused by lactic acid production, may be present [39] (see Chapter 61). Transesophageal echocardiography and transthoracic echocardiography have been utilized to identify air embolism, the route of the embolism, and the severity of the air embolism [40]. Precordial Doppler ultrasound may also be used for surveillance of air embolism by detection of alterations in the ultrasonic pattern caused by the embolism [41].
The volume of air that is likely to be lethal seems to vary with the rate of infusion and patient position. Any amount greater than 100 mL may cause death, but some patients have survived after infusion of up to 1,600 mL [42]. The mechanism by which air embolism leads to noncardiogenic pulmonary edema is not known. It is thought that entrapment of air bubbles in the pulmonary circulation leads to activation of complement, neutrophil, and platelets, resulting in mediator release and then endothelial injury [43]. This inflammatory response would then precipitate noncardiogenic pulmonary edema [44].
Aspiration of Gastric Contents
Aspiration of acidic gastric contents into the tracheobronchial tree was first described in 1946 by Mendelson [45] in women during labor and delivery. Maternal deaths from pulmonary aspiration have been steadily declining as a result of changing anesthesia practices including a shift to regional anesthesia from general anesthesia for delivery [46].
At term, several factors contribute to an increased risk of aspiration of stomach contents: (a) increased intragastric pressure caused by external compression by the gravid uterus, (b) progesterone-induced relaxation of the lower esophageal sphincter, (c) delayed gastric emptying during labor, (d) supine position, and (e) analgesia-induced decreased mental status and decreased vocal cord closure [47]. The pulmonary pathophysiologic consequences of gastric aspiration are a consequence of the acidity and the particulate content of the gastric contents and the risk of bacterial superinfection. Acid aspiration causes a direct injury to the airway resulting in desquamation and loss of ciliated and nonciliated cells including the alveolar type II cells. An inflammatory response is also triggered by the acid aspiration leading to an increase in alveolar permeability with a loss in lung compliance and a decrease in ventilation–perfusion matching [47]. Inhaled particulate matter may cause acute airway obstruction and immediate death.
The volume of acid aspiration determines, in part, the rapidity of symptom onset. Aspiration of smaller volumes may go unnoticed clinically until 6 to 8 hours later, when tachypnea, tachycardia, hypoxemia, hypotension, bronchospasm, and production of frothy, pink sputum are noted in association with diffuse infiltrates on chest radiography. Progression of chest radiographic findings may continue for up to 36 hours. The clinical course may follow one of three patterns: (a) rapid improvement during 4 to 5 days; (b) initial improvement followed by deterioration caused by supervening bacterial pneumonia, with a fatal outcome in up to 60%; and (c) early death as a result of intractable hypoxia [46]. Predictors of poor outcome include low pH, large volume, and a greater amount of particulate content of the aspirate. The bacterial pathogens in this setting are usually oropharyngeal anaerobes, although the longer the patient is in the hospital before clinical development of pneumonia, the greater the likelihood of facultative, Gram-negative bacillary and Staphylococcus aureus infections [48].
Respiratory Infections
The prevalence of pneumonia in pregnancy ranges from 0.78 to 2.7 cases per 1,000 deliveries. The maternal mortality rate from pneumonia has decreased from 20% to 3% since the advent of antibiotics [49]. The major factors in improving fetal and maternal outcome seem to have been earlier presentation and prompt institution of antibiotic therapy. Although pneumonia rarely progresses to respiratory failure, it is advisable to
assess maternal oxygenation in all cases of maternal pneumonia. The spectrum of organisms to consider is similar to that in the nonpregnant population; the most common organisms are Streptococcus pneumoniae, Haemophilus influenzae, and Mycoplasma pneumoniae. Legionella pneumonia accounts for up to 22% of community-acquired pneumonia [50] and has been reported to cause respiratory failure in pregnancy [51].
assess maternal oxygenation in all cases of maternal pneumonia. The spectrum of organisms to consider is similar to that in the nonpregnant population; the most common organisms are Streptococcus pneumoniae, Haemophilus influenzae, and Mycoplasma pneumoniae. Legionella pneumonia accounts for up to 22% of community-acquired pneumonia [50] and has been reported to cause respiratory failure in pregnancy [51].
Certain other respiratory infections (e.g., influenza, varicella, coccidioidomycosis, tuberculosis, listeriosis, and severe acute respiratory syndrome [SARS]) have been associated with increased maternal and fetal morbidity and mortality. These particular infections can be virulent in the pregnant patient because of alterations in the immune status. Specifically, during pregnancy there is a decreased lymphocyte proliferative response, a decrease in the natural killer cell activity, and a decrease in the number of helper T4 cells [52]. Fortunately, the impairment in maternal immune response is mild and the increase in maternal morbidity is small.
In the influenza pandemics of 1918 and 1957, an excess incidence of influenza pneumonia was noted among pregnant women. A 50% incidence of influenza pneumonia and an overall mortality of 27% for influenza in pregnancy were found in 1918 [53]. In the 1957 pandemic, several studies noted that 50% of deaths from influenza in women of childbearing age were in pregnant patients [53]. Autopsy reports noted that the cause of death in pregnant women was respiratory insufficiency caused by fulminant influenza pneumonia, rather than secondary bacterial infection, the more common cause of death in nonpregnant influenza patients. Similarly, the new strain of influenza A (novel influenza A H1N1), identified in 2009, has been associated with increased morbidity in pregnant women [54]. During the first weeks of this outbreak, 20 cases were identified in pregnant women; three were hospitalized and one died. These women presented with the typical symptoms of cough, fever, sore pharyngitis, rhinorrhea, diarrhea, headache, and myalgias [54].
Primary varicella-zoster infections progress to pneumonia more commonly in adults than in children, although only 20% of varicella cases occur in adults [55]. Cigarette smoking appears to be an important risk factor in the progression of varicella into pneumonia [56]. Progression to pneumonia has also been noted more frequently in pregnant women in their second and third trimesters; 10% of reported cases of varicella pneumonia have occurred in pregnant women. Historically, the maternal mortality rate for varicella pneumonia in pregnancy was 41%. Utilization of antiviral therapy has led to a decline in maternal mortality now in the range of 11% to 35% [49,56]. Respiratory failure requiring mechanical ventilation may occur in 40% to 57% of pregnant patients with varicella pneumonia, with a mortality rate of 25% [49,56].
Respiratory symptoms usually develop 2 days after the onset of fever, rash, and malaise. Typical symptoms are cough, dyspnea, hemoptysis, and chest pain [56]. Generalized varicella-zoster infections may also be associated with hepatitis, myocarditis, nephritis, thrombocytopenia, and adrenal hemorrhage [56]. Varicella during pregnancy can lead to intrauterine infection, which may result in prematurity, spontaneous abortion, and stillbirth [56]. In the absence of dissemination, herpes zoster does not appear to be associated with significant maternal morbidity or evidence of fetal infection [57].
SARS is an atypical pneumonia first described in 2002 that is caused by a coronavirus [58]. Symptoms of fever, chills, rigors, headache, malaise, and myalgias develop 2 to 7 days after exposure. A nonproductive cough or dyspnea may develop over 3 to 7 days. This may progress to hypoxemia and respiratory failure. The chest radiograph may show bilateral patchy interstitial infiltrates. The overall mortality rate for SARS is 3% [58]. One review of 12 cases of SARS during pregnancy demonstrated that 33% of pregnant women required mechanical ventilation, and the maternal mortality rate was 25% [59]. Pregnant patients with suspected or probable SARS should be placed on airborne precautions in a negative pressure isolation room [60].
Maternal Coccidioidomycosis immitis infections are rare, with less than 1 case in every 1,000 pregnancies. Historically, Coccidioidomycosis infection during pregnancy has been reported as having a 20.0% dissemination rate, compared with 0.2% in nonpregnant patients; infections contracted in the second or third trimester have a higher rate of dissemination [61]. Maternal mortality and fetal loss are preventable with appropriate treatment [61]. Case reports of cryptococcosis, blastomycosis, and sporotrichosis in pregnancy are rare enough to suggest that there is no increased susceptibility to these infections [61].
Disseminated coccidioidal infection should be suspected in patients with primary or chronic progressive coccidioidal pneumonia in whom rapidly progressive respiratory failure and a clinical picture resembling miliary tuberculosis develop. Diagnosis is sometimes difficult because sputum is positive in less than 40% of cases, and complement fixation titers may be low [61,62]. Evaluation of these patients should include a careful search for extrapulmonary disease (e.g., lumbar puncture, urinalysis, culture of skin lesions) [61,62].
Respiratory failure due to infection with Mycobacterium tuberculosis is rare, although before the advent of effective chemotherapy, maternal and infant mortality in cases of advanced disease approached 40% [63]. Pregnancy does not alter the pathogenesis of tuberculous infection or increase the likelihood of latent tuberculosis infection progressing to active disease [64]. In addition, pregnancy does not alter the response to purified protein derivative skin testing, so all pregnant women from populations recommended for screening should have a skin test performed if one has not been done previously [65].
In 2004, 27% of acquired immunodeficiency syndrome (AIDS) cases in the United States were in adult women [66]. As the number of women infected with the human immunodeficiency virus grows, the spectrum of respiratory disease in pregnancy will include an increasing proportion of opportunistic infections and other respiratory complications related to AIDS. Pneumocystis jirovecii (formerly Pneumocystis carinii) is the most common cause of AIDS-related death in pregnant patients [67]. A review of 22 cases of Pneumocystis jirovecci in pregnancy found a 59% rate of mechanical ventilation and a maternal mortality rate of 50%, compared with a mortality rate of 1% to 16% in nonpregnant patients [67]. Diagnostic evaluation follows the same protocol as in a nonpregnant patient with suspected PCP. Induced sputum should be examined for the presence of P. jirovecii; if this is negative, fiberoptic bronchoscopy with bronchoalveolar lavage should be performed.
Listeria monocytogenes, a cause of meningitis and sepsis in immunocompromised hosts, also has a predilection for pregnant women, most commonly resulting in abortion or neonatal sepsis. The incidence of Listeria infection among pregnant women is estimated at 12 per 100,000 compared with 0.7 per 100,000 in the general population [68]. The usual sporadic incidence is two to three cases for every 1 million of the population each year, but local outbreaks may occur as a result of ingestion of contaminated cheese, cabbage, or milk [68]. In an outbreak that caused 29 fetal and neonatal deaths, maternal morbidity was limited to fever and gastrointestinal symptoms [68]. However, in a few reported cases of maternal sepsis caused by L. monocytogenes, ARDS has developed [69]. In these cases, the fetal outcome was excellent despite L. monocytogenes sepsis. Diagnosis may be problematic because of difficulties in isolating the organism from respiratory tract secretions. When L. monocytogenes sepsis is suspected, cultures should be obtained from the blood, sputum, rectum, cervix, and amniotic fluid [68].
Asthma
Asthma affects between 3.7% and 8.4% of pregnant women in the United States [70]. The scope of this section is limited to asthmatic exacerbations during pregnancy that lead to respiratory failure. Studies have shown that poor asthma control during pregnancy is associated with adverse fetal and maternal outcomes. Pregnant women with frequent or severe asthma attacks were more likely to have fetal complications including growth retardation, preterm birth, low birth weight, neonatal hypoxia, and perinatal mortality. The maternal complications included preeclampsia, gestational hypertension, vaginal hemorrhage, hyperemesis, and complicated labor [71]. One study reported on the pregnancy outcomes of 486 asthmatic women who were enrolled in an active asthma management program compared with nonasthmatic, pregnant controls. There were no significant differences in either the fetal or maternal outcomes between the two groups. When active management of asthma during pregnancy is provided, maternal and fetal outcomes are no different than those of healthy, nonasthmatic women [70].
The initial clinical assessment of a pregnant woman with asthma should include personal history (detailing etiologic factors and prior therapy), physical examination, and either peak expiratory flow rate or spirometric pulmonary function testing (see Chapter 48). Peak expiratory flow rates and spirometry do not change with pregnancy and advancing gestation. Therefore, peak expiratory flow rates can be used as diagnostic and monitoring tools in the care of pregnant asthmatic women [72]. Although asthma may be the most common cause of airway obstruction during pregnancy, wheezing, shortness of breath, coughing, and sensation of chest tightness are nonspecific, and several other entities may mimic asthma (see Chapter 48).
Assuming the diagnosis of asthma is secure, certain findings taken together can be used to predict which patients are likely to require hospitalization [73]. These include diaphoresis, use of accessory muscles, assumption of upright posture, altered level of consciousness, pulse rate greater than 120 beats per minute, respiratory rate greater than 30 breaths per minute, pulsus paradoxus greater than 18 mm Hg, and peak expiratory flow rate less than 120 L per minute. When the FEV1 is no more than 15% of predicted or is less than 0.5 L, both a pulsus paradoxus of 10 mm Hg or greater and use of accessory muscles of respiration are almost always found [74,75]. Conversely, the absence of both an elevated pulsus paradoxus and use of accessory muscles usually correlates with an FEV1 greater than 40% of predicted or greater than 1.25 L [74,75]. Peak flows have been used in the evaluation of nonpregnant patients with asthma to predict the need for arterial blood gas determination. Flows greater than 200 L per minute (50% of predicted) are virtually never associated with significant hypoxemia or hypercapnia (see Chapter 48). However, as alveolar-arterial oxygen tension gradients are known to be widened in pregnancy [3], it seems prudent to obtain arterial blood gas measurements in pregnant women with asthma who do not show a significant improvement (> 20%) in peak expiratory flow rate after an initial inhaled bronchodilator treatment. Continuous oxygen saturation monitoring is also appropriate.
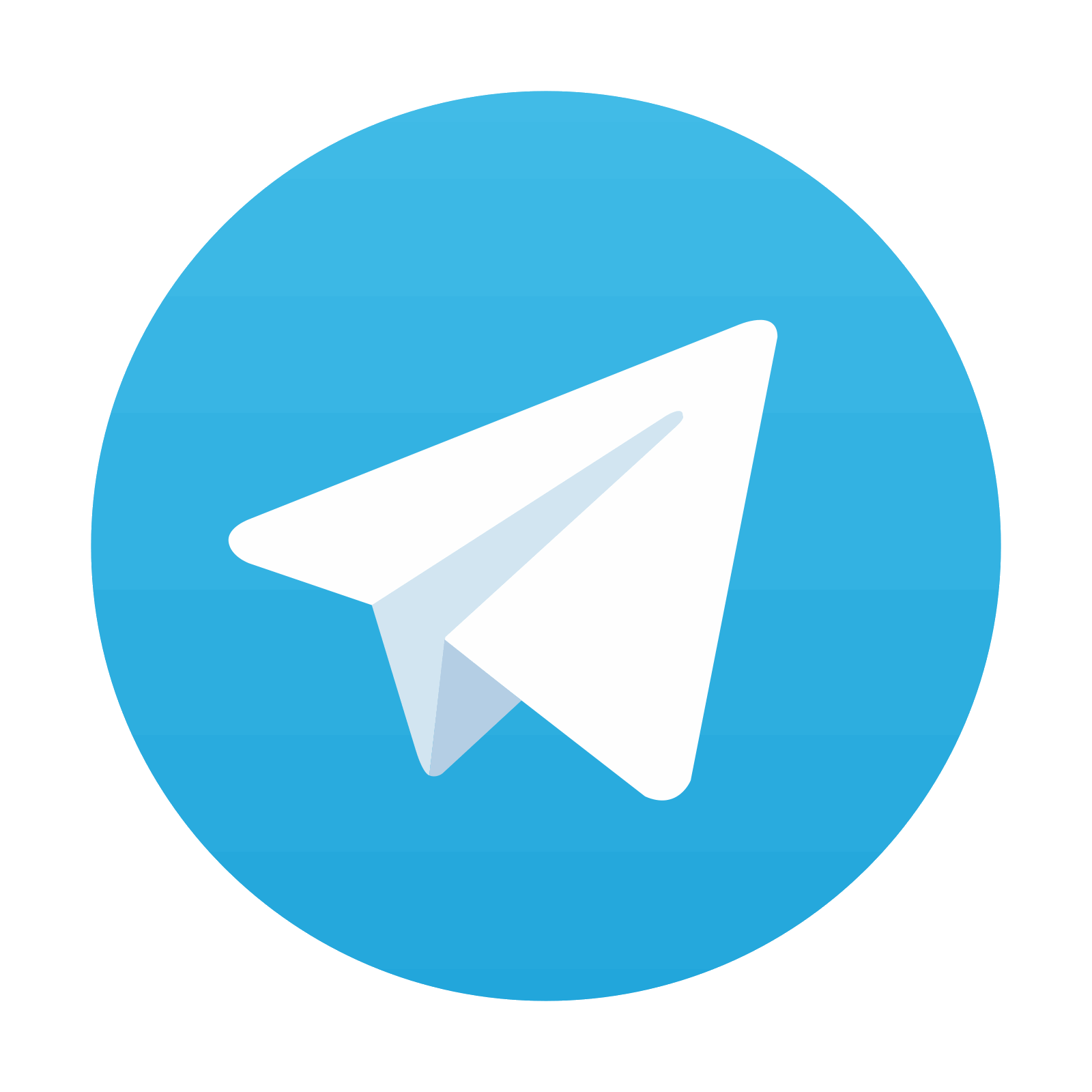
Stay updated, free articles. Join our Telegram channel
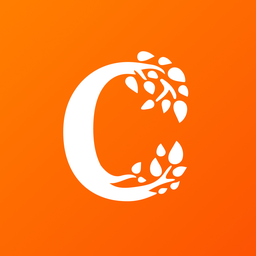
Full access? Get Clinical Tree
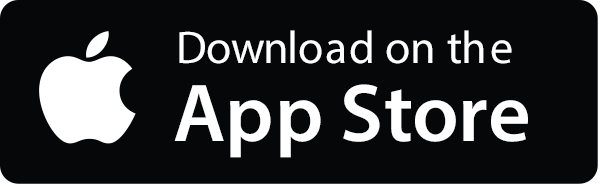
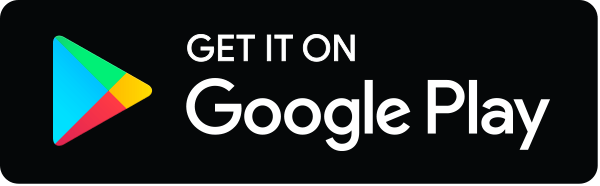