Respiratory Failure Part I: A Physiologic Approach to Respiratory Failure
Thaddeus C. Bartter
Melvin R. Pratter
Wissam Abouzgheib
Richard S. Irwin
Overview
Respiration serves to oxygenate blood and to remove the volatile waste product of metabolism, carbon dioxide and results in hypoxemia, hypercapnia, or both combined. Although it is traditional to define respiratory failure with abrupt boundaries [i.e., arterial carbon dioxide tension (PaCO2) greater than 49 mm Hg or arterial oxygen tension (PaO2) less than 50 to 60 mm Hg] [1,2], this is too simplistic for the understanding and management of respiratory insufficiency. This chapter discusses the physiologies leading to the different presentations of respiratory failure and briefly discusses management.
The alveolar PO2 and PCO2 are determined by the relationship between alveolar ventilation and perfusion ([V with dot above]/[Q with dot above] ratio). The ratio of [V with dot above] to [Q with dot above] is approximately 0.8 under normal resting conditions. In [V with dot above]/[Q with dot above] mismatch, the ratio is altered and the exchange of gaseous O2 and CO2 becomes inefficient. There are two [V with dot above]/[Q with dot above] mismatch scenarios, termed “high [V with dot above]/[Q with dot above] mismatch” and “low [V with dot above]/[Q with dot above] mismatch.” High-[V with dot above]/[Q with dot above] mismatch occurs in a lung region that receives a disproportionate increase in ventilation or decrease in blood flow. Low [V with dot above]/[Q with dot above] mismatch occurs in a lung region that receives a disproportionate decrease in ventilation or increase in blood flow. As will be seen, low [V with dot above]/[Q with dot above] mismatch plays a major physiologic role in respiratory failure.
In many cases of respiratory failure, a low PaO2 is coupled with an elevated PaCO2, but the physiology of oxygenation is different from that of CO2 removal. The differences stem in part from the differences in the capacity of blood to carry each of the two gases. Oxygen must bind to hemoglobin for effective transport. Saturated hemoglobin can carry 1.39 mL of O2 per gram, whereas plasma can carry only 0.003 times the PaO2; only approximately 1% of oxygen transport is independent of hemoglobin. The amount of O2 that blood can carry is thus limited by hemoglobin concentration (and function). Once hemoglobin is saturated, a doubling of the alveolar oxygen concentration has no meaningful impact on oxygen transport. For this reason, alveoli with high [V with dot above]/[Q with dot above] mismatch cannot add extra oxygen to the pulmonary capillary blood to compensate for alveoli with low [V with dot above]/[Q with dot above] mismatch in which the hemoglobin of the associated pulmonary capillary blood is not fully saturated [3].
The biochemistry of CO2 is very different. CO2 diffuses readily into blood; its quantity increases almost linearly as the PaCO2 increases. The mechanisms for CO2 transport include a buffering system mediated by carbonic anhydrase and the formation of carbonyl compounds. The net result is that a doubling of alveolar ventilation essentially doubles CO2 elimination. For this reason, unlike the physiology of O2 transport, lung units with normal or high [V with dot above]/[Q with dot above] relationships can compensate for areas with low [V with dot above]/[Q with dot above] relationships. Because of these differences between the two gases, abnormalities in their values are not always linked, and it is useful to approach the factors that can cause each to be abnormal.
Normal Blood Gas Values
“Normal” PaO2 can be shown to decrease with age and with the supine position [4]. There is significant standard deviation, and in clinical practice the normal range for most laboratories, 80 to 100 mg Hg, suffices.
In normal human homeostasis, the PaCO2 is tightly regulated by respiration at or close to 40 mm Hg. Unlike the PaO2, the normal PaCO2 remains at 40 mm Hg throughout life. It is unaffected by age [4] or position [5].
The normal pH of human arterial blood is at or close to 7.40. Like the PaCO2, there is no predicted change with age.
Hypoxemia and Hypercapnia
There are six basic pathophysiologic mechanisms that can lead to hypoxemia. Some also cause hypercapnia [1,6,7]:
Low partial pressure of inspired O2 (PIO2)
Diffusion impairment
Right-to-left shunt
Low [V with dot above]/[Q with dot above] mismatch
Hypoventilation
High partial pressure of inspired CO2
Only three are clinically important: low [V with dot above]/[Q with dot above] mismatch, right-to-left shunt, and hypoventilation. [V with dot above]/[Q with dot above] mismatch and hypoventilation can cause both hypoxemia and hypercapnia.
Low Partial Pressure of Inspired Oxygen
Low PIO2 is a potential cause of hypoxemia. A low PIO2 occurs only at high altitudes and in conditions when other gases are present; it is not in the differential diagnosis of normal clinical management.
Diffusion Impairment
It was once thought that thickening of alveolar walls could lead to an increase in the diffusion distance great enough to prevent equilibrium of the partial pressure of oxygen between the alveoli and the associated pulmonary capillary blood. This
physiologic concept is known as “alveolar–capillary block syndrome,” [8]. Subsequent data, however, indicated that even radiographically “homogeneous” pulmonary disease rarely alters alveolar–capillary membranes uniformly throughout the lung. In addition, the efficiency of gas exchange within any single alveolus is such that even with a barrier to diffusion, diffusion impairment is not a factor; by the time blood leaves the alveolar capillaries, alveolar and capillary gas partial pressures are equal. Thus, hypoxemia at rest in patients with pathologies such as interstitial disease is due not to “alveolar–capillary block” but rather to areas of low [V with dot above]/[Q with dot above] mismatch. In contrast, there may be a component of “alveolar–capillary block” in exercise; as the transit time of blood through alveolar capillaries decreases, there may be some true physiologic impairment of capillary/alveolar gas equilibrium that could result in hypoxemia [9].
physiologic concept is known as “alveolar–capillary block syndrome,” [8]. Subsequent data, however, indicated that even radiographically “homogeneous” pulmonary disease rarely alters alveolar–capillary membranes uniformly throughout the lung. In addition, the efficiency of gas exchange within any single alveolus is such that even with a barrier to diffusion, diffusion impairment is not a factor; by the time blood leaves the alveolar capillaries, alveolar and capillary gas partial pressures are equal. Thus, hypoxemia at rest in patients with pathologies such as interstitial disease is due not to “alveolar–capillary block” but rather to areas of low [V with dot above]/[Q with dot above] mismatch. In contrast, there may be a component of “alveolar–capillary block” in exercise; as the transit time of blood through alveolar capillaries decreases, there may be some true physiologic impairment of capillary/alveolar gas equilibrium that could result in hypoxemia [9].
Right-to-Left Shunt
In right-to-left shunt, blood from the right heart does not come into contact with oxygenated air before reaching the left heart; ventilation and perfusion are uncoupled [5]. Three kinds of shunt are recognized: cardiac, pulmonary vascular, and pulmonary parenchymal [10]. In a cardiac shunt, a defect allows blood to pass directly from the right atrium or ventricle into the left-sided chamber. For cardiac shunt to occur, there must be some relative increase in right-sided pressures. In a pulmonary vascular shunt, the shunting of blood occurs through arteriovenous malformations within the pulmonary vascular bed. These arteriovenous malformations can be small and not visible on chest imaging (as in some cases of cirrhosis), or large and visible as parenchymal densities (as with hereditary hemorrhagic telangiectasia) [11]. In pulmonary parenchymal shunt, alveolar consolidation or atelectasis prevents gases from reaching alveoli while blood flow continues through their capillary beds. Examples of conditions that cause parenchymal shunt are pneumonia, lobar collapse, and acute respiratory distress syndrome.
Note that right-to-left shunt is listed as a cause of hypoxemia but not of hypercapnia because of the capacity of alveoli with normal or high [V with dot above]/[Q with dot above] ratios to compensate for the lack of clearance of CO2 from shunted blood [6]. If the only gas exchange defect present is shunt, increased ventilation to the perfused alveoli leads to a normal PaCO2 [6]. This increased ventilation has no effect on the PaO2; as already noted, the dependency on hemoglobin for blood to carry oxygen results in an inability of areas with normal or elevated [V with dot above]/[Q with dot above] ratios to compensate for areas with low [V with dot above]/[Q with dot above] ratios. Thus, shunt is a cause of nonhypercapnic hypoxemic respiratory failure.
[V with dot above]/[Q with dot above] Mismatch
Low [V with dot above]/[Q with dot above] mismatch is the dominant physiology in abnormalities of gas exchange. Mild to moderate degrees of low [V with dot above]/[Q with dot above] mismatch can cause hypoxemia alone, whereas more severe [V with dot above]/[Q with dot above] mismatching leads to hypoxemia with hypercapnia. (For a patient breathing room air, [V with dot above]/[Q with dot above] mismatch never causes hypercapnia in the absence of hypoxemia.) There are two reasons why a substantially greater amount of low [V with dot above]/[Q with dot above] mismatch must be present to cause hypercapnia than to cause hypoxemia. The first reason is the higher solubility of CO2 in blood as discussed earlier [6]; there is no saturation limit for CO2. Thus while normal alveoli cannot increase oxygen uptake significantly after hemoglobin saturation, they can increase CO2 removal as venous CO2 content rises. The second reason is that, just as with shunt, patients with low [V with dot above]/[Q with dot above] mismatch increase their minute ventilation to compensate for the potential elevation in CO2 that the low [V with dot above]/[Q with dot above] areas would otherwise generate [6]. With severe [V with dot above]/[Q with dot above] mismatch, there are not enough normal and high [V with dot above]/[Q with dot above] alveoli to compensate for the hypercarbia of those with low [V with dot above]/[Q with dot above] mismatch; hypercapnia occurs in addition to hypoxemia.
Hypoventilation
Hypoventilation refers to conditions in which minute ventilation is reduced relative to the metabolic demand present for oxygen uptake and CO2 production. By necessity, when minute ventilation is reduced alveolar ventilation must also be abnormally low, resulting in decreased gas exchange between the external environment and the alveoli [6]. Hypoventilation by definition causes both arterial hypoxemia and a raised arterial PCO2. Some physicians use the terms “hypoventilation” and “carbon dioxide retention” interchangeably, a usage that confuses physiologies. Pure hypoventilation represents decreased minute ventilation with normal lungs. In contrast, other conditions that cause carbon dioxide retention (low [V with dot above]/[Q with dot above] mismatch) are caused by airway or parenchymal lung disease and usually are associated with increased minute ventilation.
In hypoventilation as defined earlier, the alveolar PCO2 can rise to the point that the partial pressure of O2 is significantly reduced. The disorders that cause hypoventilation are called the extrapulmonary causes of respiratory failure because they do not involve abnormality of the pulmonary gas exchange mechanisms [7,12,13]. A defect leading to hypoventilation can occur anywhere in the normal physiologic linkages that affect minute ventilation; the differential diagnosis of extrapulmonary respiratory failure is listed in Table 46.1. Note that in this categorization, obstruction at or above the trachea and other large airways is classified as an extrapulmonary disorder because of the fact that the gas exchange mechanisms of the lung remain intact.
High Partial Pressure of Inspired Carbon Dioxide
The inhalation of a gas containing CO2 can cause hypercapnia although it is not usually part of the differential diagnosis in clinical medicine. It does occur occasionally in iatrogenic situations; patients on a t-piece with extended tubing attached to the expiratory port may be forced to re-breathe exhaled CO2 to the point of hypercapnia.
Table 46.1 Differential Diagnosis of Extrapulmonary Respiratory Failurea | ||||||||||||||||
---|---|---|---|---|---|---|---|---|---|---|---|---|---|---|---|---|
|
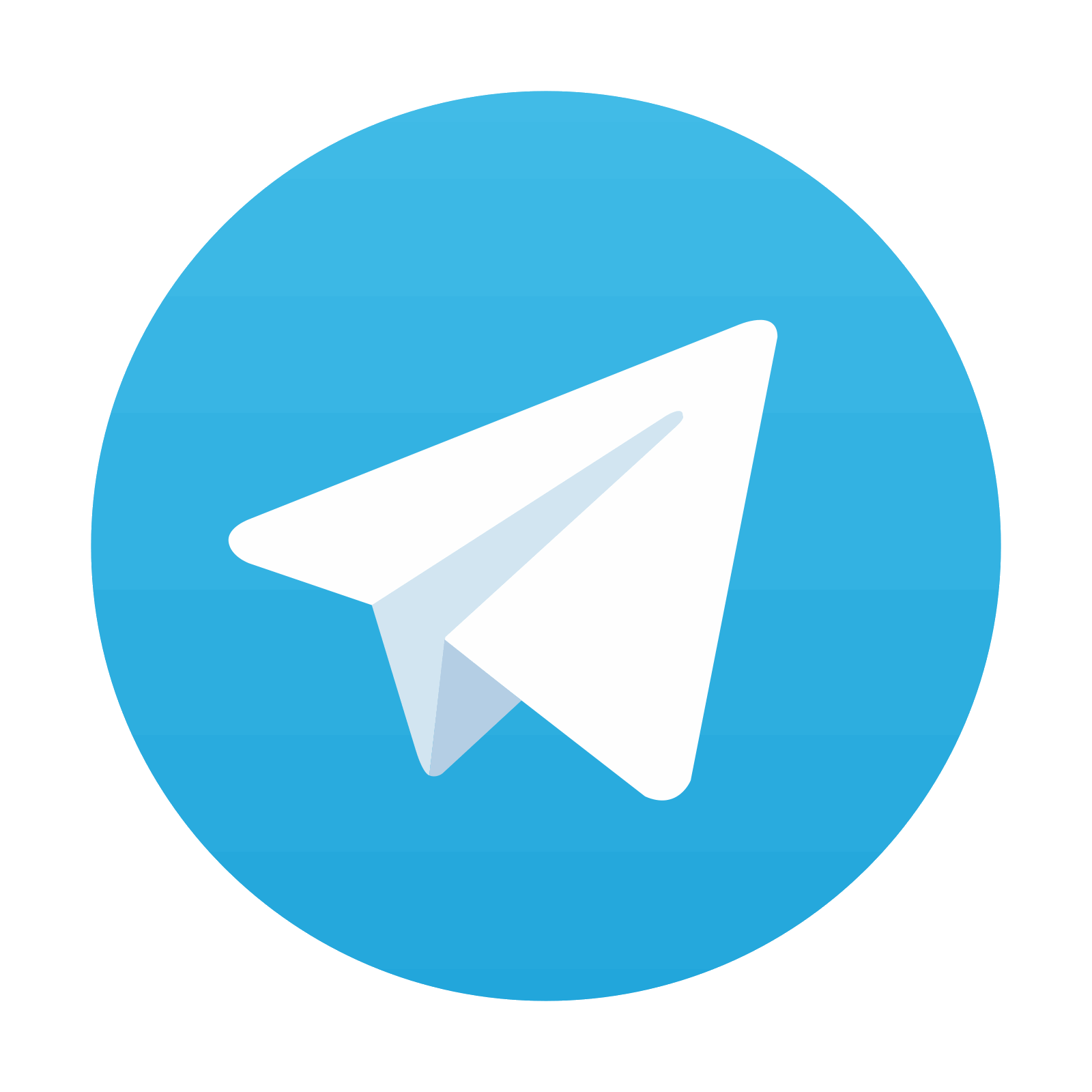
Stay updated, free articles. Join our Telegram channel
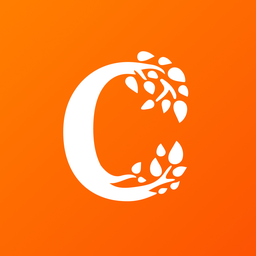
Full access? Get Clinical Tree
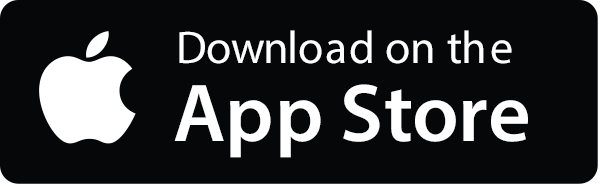
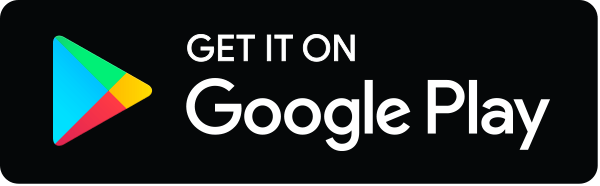