Flat-plain damage patterns from a ground-level nuclear detonation (viewed from above). The “cigar-shaped” fallout pattern is a simplification, as the fallout pattern will be determined by the direction and speed of upper-level winds at the time of the detonation.
Exposures to fallout after a nuclear detonation will vary according to distance, meteorological conditions, and available shelter. If the graphic depicted in Figure 33.1 represented a 10 kT ground detonation, fallout would arrive at a location 2.5 km downwind after approximately 1 minute. Estimated dose rates that might be measured 2.5 km downwind at different time intervals after the detonation are provided in Table 33.1.34,35
Estimated Dose Rates at Different Time Intervals at a Point 2.5 km Downwind from 10 kT Nuclear Detonation
Time Post-Detonation | Outdoor Exposure Rate from Fallout |
---|---|
1 min | 300 Gy/min |
15 min | 0.25 Gy/min |
120 min | 0.03 Gy/min |
480 min | 0.001 Gy/min |
As Table 33.1 demonstrates, dose rates decline rapidly over time as fission products with a short half-life decay, so the importance of obtaining shelter cannot be overstated. Underground shelters can substantially reduce doses from fallout. However, if basement shelters are not available, sheltering away from the ground in the upper floors of buildings (assuming windows are intact and ventilation systems are off) can also provide enhanced protection when compared with ground-level shelters. The quality of a shelter’s building materials influences the degree of protection it affords, with stone office buildings providing more shelter than a wood frame house.
Prompt Effects
Prompt effects are those occurring as a direct result (and within the first minutes) of the detonation. They encompass radiation, blast, and thermal effects, which are described briefly in the next sections.
Radiation Effects
Prompt radiation includes: 1) neutrons and gamma rays emitted as a direct result of fission; 2) alpha and beta particles and gamma radiation emitted by unstable fission products; and 3) radiation emitted by neutron-activated debris swept up into the fallout cloud from the immediate vicinity of the detonation. Prompt radiation doses decline according to the inverse square law (doubling the distance from the detonation site reduces the dose by a factor of 4), but are sufficient to produce signs and symptoms of ARS at distances up to approximately 1.5 km from a 10-kT detonation. Within this radius, terrain and building structures may provide shielding from the thermal radiation but will not greatly attenuate the neutron and gamma radiation.
Blast Effects
The blast wave accounts for approximately 50% of the energy released by a nuclear detonation. Consequently, blast effects make the greatest single contribution to the immediate damage caused by the detonation. The blast wave moves outward from the point of detonation at supersonic velocity, generating winds of up to several hundred kilometers per hour. Injury and lethality due to blast effects are very difficult to predict because of the varied and complex mechanisms whereby the shock wave can interact with structures and people. Modeling suggests that many or most casualties receiving a prompt radiation dose exceeding a few hundred centigray (cGy) will probably die from blast effects.
Thermal Effects
Thermal radiation (heat) accounts for approximately 35% of the energy released by air bursts such as those at Hiroshima and Nagasaki (this percentage will be reduced if the detonation occurs at ground level). Thermal radiation will produce primary and secondary burn injuries: primary burns are caused by direct exposure to the thermal pulse; and secondary burns result from the incendiary effects of the radiation on flammable items. In certain environments, fire storms may ensue as individual fires coalesce. Modeling suggests that burns will be severe at distances of up to 1 km for a 1-kT burst and up to 3–4 km for a 10-kT detonation. Within these zones, burn lethality will be enhanced in victims experiencing blast and radiation effects.
Fallout
Ground bursts aerosolize more radioactive debris with a wider range of particle sizes than air bursts. The particles of larger size tend to fall sooner. Ground bursts thus produce more concentrated fallout in larger amounts than air bursts. Such fallout poses an extreme radiological hazard to downwind populations. The deposition of fallout will be heavily influenced by weather patterns and may not be uniform. Some areas (“hotspots”) receive greater amounts than others as a result of local meteorological conditions.
Fallout Composition
Fission of nuclear weapons materials may generate more than 300 isotopes of thirty-six different elements, with each isotope having a unique specific activity and half-life. Precise fission yields are dependent on the involved isotope and the energy of the neutrons causing fission.34,36 In addition to these fission products, the fallout cloud produced by a ground burst will carry a substantial amount of neutron-activated debris. Table 33.2 provides estimates of fission yields for some of the more prevalent elements produced from 235U by slow neutrons (the combined yield exceeds 100% because each fission results in two products).36 Elements listed by name only are represented by multiple isotopes. Due to the differential radioactive decay as the fallout cloud travels downwind, the isotopic composition of deposited fallout will change from location to location and over time.
Yield of Fission Products
Element | Percentage Yield |
---|---|
Rubidium | 7 |
90Sr | 5.8 |
Yttrium | 24 |
Zirconium | 12.5 |
Molybdenum | 11.3 |
Iodine | 21.7 |
135Xe | 6.5 |
137Cs | 6.2 |
Barium | 12.6 |
Cerium | 9.3 |
Most of the isotopes occurring in fission products emit beta particles, with a high percentage of these also emitting gamma radiation. Beta particles emitted by fallout can produce cutaneous burns when this radioactive material remains in contact with skin for extended periods. Beta burns were the most prominent clinical manifestation in Marshall Islanders after exposure to fallout in the early 1950s, for example. Because beta particles are not penetrating, however, beta exposure will not contribute significantly to bone marrow doses and thus will not produce many of the systemic features of radiation exposure. Gamma rays, which are penetrating, make the greatest contribution by far to bone marrow irradiation from fallout. Few if any of the fission products are alpha emitters. However, alpha emitters will be represented in fallout in the form of unfissioned 235U or plutonium 239 (239Pu). That being said, the radioactivity of the fission products is far greater in aggregate than the activity of the dispersed fissile material and constitutes a much greater threat to downwind populations. Neutron radiation is not a component of fallout.
Among the isotopes in fallout of greatest concern are 90Sr, 137Cs, and the iodine isotopes. 90Sr has a long half-life, is concentrated in bones and teeth, and emits a high-energy beta particle. 137Cs also has a long half-life, emits gamma rays, and behaves biologically like potassium (and thus can be passed up the food chain). The iodine isotopes, which have moderate half-lives (8 days for 131I), are avidly taken up by the thyroid gland and produce a significant cancer risk in younger individuals.
Dynamic Dose Rates Due to Fission Product Decay
Doses and dose rates due to fallout reflect patterns of deposition and thus are heavily influenced by meteorological conditions and other factors. The behavior, and to some extent the composition, of fallout produced by a ground burst in an urban environment affected by microclimate features is difficult to model precisely. Table 33.3 gives approximate predicted radiation doses received by fully exposed individuals directly under the fallout plume at various distances from the point of detonation for the first hour following a 1- or 10-kT surface blast.34,35 It is clear from the calculated doses that the initial dose rates produced by fallout are extremely high. The low doses at large distances will increase as the major part of the fallout plume passes overhead. Therefore, early evacuation and/or sheltering are among the most important and effective strategies for reducing exposure.
Fallout Doses in First Hour
Range (km) | 1 kT detonation (Sv) | 10 kT detonation (Sv) |
---|---|---|
1 | 4,100 | 32,000 |
2 | 58 | 930 |
4 | 14 | 79 |
8 | 3.3 | 13 |
10 | 1.9 | 7.3 |
20 | .12 | .64 |
40 | minimal | minimal |
Fortunately, most of the radioactive isotopes in fallout decay rapidly. Table 33.4 shows the rate of fallout decay, expressed as a percentage of the dose rate at 1 hour, during the first 12 hours following detonation.35
Fallout Decay
Time after detonation (hours) | Percentage of dose rate at 1 hour |
---|---|
1 | 100 |
2 | 43 |
4 | 19 |
6 | 11 |
8 | 8 |
10 | 6 |
12 | 5 |
Because of the high early dose rates, the cumulative doses observed in exposed populations can still be quite large. Figure 33.2 shows estimated cumulative doses at different distances for fully and continually exposed populations for the first 24 hours following a detonation.34,35 Such dose estimates are considered merely illustrative, as individuals almost certainly will move in and out of shelters and through areas with different degrees of fallout deposition during this time period.

Estimated cumulative 24-hour fallout dose from HOTSPOT model.
Benefits of Shielding
The most effective way to reduce fallout exposure is evacuation. For a variety of reasons, this may be difficult to accomplish within the appropriate timeframe, especially in areas close to the detonation where fallout could reach the ground within minutes and the doses of radiation would be highest. Sheltering-in-place may be more feasible and can provide substantial protection against exposure. Table 33.5 shows the estimated dose reduction factors for different types of shelter.34,35
Shelter Dose Reduction Factors
Type of Shelter | Dose Reduction Factor |
---|---|
1 meter underground | 0.0002 |
Frame house | 0.3–0.6 |
Basement | 0.05–0.1 |
Upper stories of apartment | 0.01 |
Shelter with 0.6 meter earth cover | 0.005–0.02 |
Current State of the Art
Basics of Radiation Biology
Ionizing radiation exists in two forms: electromagnetic (gamma rays and X-rays) and particulate (alpha particles, beta particles, and neutrons). Both electromagnetic and particulate forms of ionizing radiation can contribute to the radiation injury sustained after accidental or deliberate exposures. Depending on the nature of the precipitating event, the radiation exposure for a given individual may be classified as localized or whole-body. In addition, when fallout or other radioactive substances are involved, internal or external deposition of these materials can result (causing radioactive contamination inside the human body or on its surface).
ARS encompasses a set of complex pathophysiological processes precipitated by exposure to high doses of radiation.39 The latency, severity, and duration of the various manifestations of ARS are a function of the organ system affected, the radiation dose and dose rate, and the “quality” (particulate or electromagnetic) of the precipitating exposure. Much of the immediate damage at the cellular level caused by radiation is nonspecific, mediated through the generation of free radicals and peroxides. Nonspecific lipid peroxidation, DNA damage, and protein oxidation lead to alterations of gene transcription and messenger RNA translation as part of the cellular stress response. This promotes inflammation and other changes in the tissue microenvironment that contribute to cell death. In patients receiving sufficiently high doses of radiation, the resulting cellular, tissue, and organ damage can trigger a cascade of events leading to multi-organ failure and death. It is clear from both animal experiments and accidental high-dose exposures in humans that the kinetics of lymphocyte, neutrophil, and platelet depletion – and the time course of ARS symptoms in general – are accelerated at higher doses.
Knowledge of ionizing radiation’s acute effects on human subjects has been derived from:
1. Animal studies;
2. Studies of Japanese populations exposed to radiation from atomic weapons;
3. Studies of normal tissue injury in patients receiving radiation therapy, typically for cancer; and
4. Accidents involving radiation workers, radiotherapy patients, and (rarely) civilian populations.
The relative value of the first data source is limited by inherent uncertainties regarding interspecies extrapolations. Studies of Japanese atomic bomb survivors have helped quantify the risk of subsequent malignancies and other delayed effects of radiation exposure. However, this population has provided less insight into ARS because little detailed clinical or laboratory data were collected immediately after the attacks. Radiation oncologists have gained extensive experience managing side effects in radiotherapy patients, but such patients are typically treated with fractionated and highly focal therapy, minimizing systemic impacts. In addition, these patients often receive cytotoxic chemotherapy in addition to radiation, so their clinical presentations may be complicated by effects from their primary disease or other concurrent therapy. Thus, it has been difficult to use this clinical experience to develop protocols for the management of ARS.
The most reliable information about ARS is derived from victims of radiation accidents. Not surprisingly, accidental exposures have been highly variable, ranging from alpha emitter inhalation in plutonium production and processing facilities to mixed gamma/neutron field exposures produced by criticality accidents. The largest and most contemporary cohort of patients with ARS consists of Chernobyl emergency workers who received exposures in the deterministic range (doses high enough to produce acute injury). These workers were subjected to the combined effect of radiation from several sources: 1) short-term external gamma/beta radiation from the gas emission cloud in the immediate area surrounding the reactor at the time of the explosion; 2) external gamma/beta radiation of decreasing intensity, from fragments of the damaged reactor core scattered over the site; 3) inhalation of gases and aerosol dust particles containing a mixture of radionuclides reflecting the isotope inventory of the reactor core at the time of the accident; and 4) deposition of these particles on the skin and mucous membranes.37 Few of these accidents have been good surrogates for the types of exposures that would occur following detonation of a nuclear device or the deposition of fallout. A notable exception was the accidental exposure of Marshall Island inhabitants to fallout from the detonation of a nuclear device in 1954. Estimates of exposure prior to evacuation were less than 2 Gy in the most severely irradiated, with beta burns and mild depression of blood counts being the predominant acute effects.38
An estimate of the gamma radiation dose that is lethal to 50% of the population within 60 days of exposure is known as the LD50/60. It ranges from approximately 350 cGy for unsupported adults to 600–700 cGy in persons receiving extensive supportive care, including antimicrobials and blood product transfusions.39 In medically austere environments, or in the presence of combined injuries, the LD50/60 will likely decrease substantially. For example, it has been estimated that the LD50/60 for victims of the atomic bombings was approximately 220 cGy.39,40
Determinants of Biological Effects
Dose Rate
The frequency and dosage fractionation (amount administered during any one exposure) with which radiation is delivered is an important determinant of the overall biological effects. This fact is exploited by radiation oncologists who seek to maximize tumor destruction while minimizing normal tissue injury by administering the prescribed radiation to target tissues in a series of small doses delivered daily over several weeks. In terms of lethality, studies in small animals have demonstrated that for continuous exposures, the gamma/x-ray LD50/30 declines as dose rates increase.43 For example, Neal found that the LD50/30 in mice declined from 1,100 cGy to 790 cGy as the dose rate increased from 2.5 cGy/min to 706 cGy/min.42 These findings are generally consistent with observations in humans, although it has not been possible to test this hypothesis systematically.
Radiation Quality
The quality of radiation received (alpha, beta, gamma, or neutrons) is an important determinant of the biological effects observed for a given dose. Neutron radiation, for example, is more penetrating than alpha or beta radiation but less penetrating than gamma radiation. Because neutrons are comparatively heavy particles with a moderate degree of penetration, neutron radiation has a high relative biological effectiveness (RBE). In other words, they cause a greater degree of injury than other types of radiation with the same energy. The RBE in canines for an exposure with a mixed radiation field having a neutron-gamma ratio of 5.4:1 is about 1.7 (i.e., the ratio of the LD50/30 with gamma radiation alone to the LD50/30 of the mixed field is 1.7:1).44,45 Consistent with the higher RBE observed for neutrons, increasing the neutron-gamma ratio at a fixed exposure (increasing the neutron component of the radiation while holding the energy level constant) has been shown to accelerate and prolong the suppression of white blood cell (WBC) counts.43 The effect of increasing fractions of fission neutrons on survival in rodents with and without combined injuries is illustrated in Figure 33.3.

Effect of fission-neutron dose fraction on LD50 in mice.
Alpha particles, which consist of two protons and two neutrons (and thus are identical to the nucleus of a helium atom), are charged and relatively heavy. They interact intensely with atoms in materials they encounter, dissipating their energy over a very short range. As such, they are not highly penetrating and adequate shielding against alpha particles can be provided by a single sheet of paper. External exposures do not represent a significant hazard. However, their RBE is substantially greater than that of gamma rays because they are highly ionizing and they can significantly damage cells and tissues if taken internally.
Beta particles are electrons emitted from the nucleus of a radionuclide by the decay of a neutron into a proton, an electron, and an antineutrino. The energy of the ejected beta particle can vary. Some energetic beta particles may penetrate tens of millimeters into the skin and thus pose both an external and an internal hazard. “Beta burns” are characteristic of exposure to fallout, which contains a high number of beta-emitting radionuclides.34,40,46
Differences in radiation quality may have implications for the development of radiation countermeasures. Neutrons, for example, are more likely to cause damage by direct effects on cellular macromolecules than gamma radiation (which mediates its effects indirectly through the generation of free radicals). Consequently, fission-spectrum neutrons appear to be significantly more mutagenic and thus potentially more carcinogenic than gamma radiation. In hybrid B6CF1 mice, a 97% neutron exposure of 150 cGy is approximately equivalent in mutagenic potential to 750 cGy of 60Co gamma rays. For this strain of mice, amifostine administered prior to neutron exposure had a dose reduction factor (DRF) of 1.4 for mutagenic endpoints, whereas for gamma exposures, the DRF was 2.4.47 Therefore the radiation-induced biological impact was reduced for a given neutron dose by 1.4 and for a given gamma dose by 2.4.
Physiological Variables
Cells are most sensitive to ionizing radiation during mitosis. One of the chief determinants of individual tissue sensitivity (and thus of organs) is the rapidity of cell division occurring at the time of irradiation. Consequently, tissues with high rates of cellular turnover, such as bone marrow and the gastrointestinal epithelium, are exquisitely sensitive to radiation while tissues with low rates of turnover (e.g., muscle, kidney) are intrinsically radioresistant.
In individuals, specific genetic defects that cause impaired DNA damage recognition and repair, such as mutations in the ATM (ataxia-telangiectasia mutated) or NBS1 (Nijmegen breakage syndrome) gene loci, are associated with profound hypersensitivity to ionizing radiation and a predisposition to malignancy.48,49 These and other genetic lesions associated with radiosensitivity are extremely rare. Most of the variability observed in radiosensitivity between individuals is not currently understood and does not appear to result directly from known single nucleotide polymorphisms or other genetic defects.
Studies (primarily in rodents) on the contribution of physiological variables to radiosensitivity demonstrate that significant differences are observed between animal strains in this regard, confirming that complex genetic factors are important determinants of radiation injury. Age and sex also appear to account for observed differences in the lethality of radiation exposures, with animals at the extremes of age and females exhibiting lower LD50/30 values.24,26,51,52 Whether such variables influence outcomes after acute exposures for large animals or humans has not been determined.
Biodosimetry and Radiological Triage
Cytogenetic Biodosimetry
The major determinants of clinical outcome following an acute radiation exposure are the dose received by the affected individual and the section of the body that is irradiated. Estimating this dose (in a process termed “biodosimetry”) thus becomes a critical part of clinical management of such individuals.53
Prior to 1960, determination of dose relied on reconstruction of the accident. This included health physics studies, time and motion simulation, and analysis of any physical dosimeters that might have been present. Medical management was reactive, heavily weighted toward clinical response to the evolution of various ARS characteristics or of acute local cutaneous injury.
Over the next 50 years, many biodosimetry techniques that have been evaluated. However, the gold standard for radiation dose estimation remains the measurement of cytogenetic changes evaluated by the lymphocyte metaphase-spread dicentric assay (Figure 33.4). This test utilizes dividing lymphocytes arrested in metaphase. The number of chromosomes with 2 centromeres (dicentrics) is counted and this yields an accurate estimate of the radiation dose. The dicentric chromosome assay has been extensively developed and harmonized to international standards. However, the assay remains both time-consuming and labor intensive. Therefore, less precise techniques have been developed that enable the treating physician to estimate the relative magnitude of a patient’s exposure relatively quickly and with some degree of confidence. The early initiation of therapy based on such techniques may offer critical benefits, as studies indicate that the likelihood of survival can be significantly increased with appropriate aggressive medical intervention and care.54,55

Cytogenetic abnormalities noted after irradiation in peripheral blood lymphocytes of a patient exposed to high-dose radiation. Dicentric chromosomes and ring abnormalities are relatively radiation-specific and are characteristic of changes observed.
For acute triage after a radiation event, some authorities have recommended that medical personnel rely heavily on clinical signs, lymphocyte kinetics, the time to emesis, and cytogenetic biodosimetry.55–58 The Biological Dosimetry Team at the U.S. Armed Forces Radiobiology Research Institute (AFRRI) has developed a multi-parameter triage scheme that provides an immediate statistical evaluation of dose.59 These techniques have been incorporated into a diagnostic computer algorithm called the Biodosimetry Assessment Tool (BAT). It and related decision support tools are available online at http://www.afrri.usuhs.mil/outreach/biodostools.htm. The algorithms developed for these tools are also the basis for the online dose estimators maintained on the U.S. National Library of Medicine’s Radiation Emergency Medical Management (REMM) website (http://www.remm.nlm.gov/ars_wbd.htm).
Harmonized international protocols have been established for the conventional lymphocyte metaphase-spread dicentric assay, which has been used over several decades to guide the management of victims with severe radiation overexposure. Another cytogenetic test, the premature chromosome condensation (PCC) assay, may offer certain advantages over conventional metaphase-spread chromosome-aberration biodosimetry techniques.60–70 The latter techniques are robust, but as mentioned previously, they are laborious and time-consuming.
For potential high-dose irradiation above the median lethal dose, it is expected that radiation-induced cell death and delay in cell cycle progression into mitosis will interfere with dose estimation. To overcome this limitation, quantitative analysis of radiation-induced damage may be performed using resting peripheral lymphocytes in lieu of metaphase spreads. Use of interphase cytological assays, such as the PCC assay, can eliminate the inherent problems associated with the use of metaphase-spread cytogenetic assays. The PCC assay requires only a small amount of blood (0.5 ml) and chromosomal damage may be visualized within a few hours after obtaining the blood sample. A modification of the PCC assay, the interphase-based rapid interphase chromosome aberration (RICA) assay, is a simple alternate to the metaphase-spread based dicentric analysis. In the RICA assay, damage involving specific chromosomes is examined in chemically induced PCC spreads after fluorescence in situ hybridization (FISH) with specific whole-chromosome DNA hybridization probes. The use of FISH greatly expands the dose range over which the PCC technique can be used and facilitates the recognition of chromosome exchange aberrations.62 In summary, PCC techniques are reliable at a wide range of doses. The assay may be used to characterize low dose exposures as well as life-threatening acute high doses of both electromagnetic (gamma rays) and particle (neutrons, alpha) radiation.60,70 In addition, PCC assays can discriminate between total and partial-body exposures.
Radiation experts have suggested that the dicentric assay could be adapted for the triage of mass casualties.64,65,66,69 Lloyd and colleagues described an ex vivo simulation of an accident with mass casualties receiving whole- or partial-body irradiation in the 0–8 Gy range. Faced with a hypothetically urgent need for rapid results, clinical triage was accomplished by scoring as few as 20 metaphase spreads per subject, compared with the typical 500 to 1,000 spreads scored in routine analyses. In such a situation, twenty cells could be scored per person initially, and a preliminary dose communicated to the treating physicians. If the patient’s clinical symptoms suggested a dose significantly higher than the preliminary estimate, the assessment could be improved by scoring up to fifty cells. Using the dicentric assay in this triage mode, a throughput of 500 or more samples per week per laboratory may be feasible.67 WHO has performed surveys of the capabilities and needs of member nations with respect to cytogenetic biodosimetry. A European network for biological dosimetry has been created to strengthen regional emergency preparedness and response capabilities for a large-scale nuclear accident or radiological emergency.66,69,70 Similar proposals have been made to create a comparable network in North America, but no such organization exists to date. Table 33.6 lists current AFRRI recommendations on the type of definitive biodosimetry to use when a preliminary estimate of a given dose has been obtained.60,70
Proposed Biodosimetry Technique as a Function of Expected Dose BM = bone marrow; GI = gastrointestinal; PCC = premature chromosome condensation. Used with permission from NATO/RTO and Prasana
Dose Range (Gy) | Proposed Validated Dosimetry Method | Prodromal Effects | Manifest Symptoms | Survival Expectancy |
---|---|---|---|---|
0.1–1 | Dicentrics/PCC | none to mild (1–48 h) | none to slight decrease in blood count | almost certain |
1.0–3.5 | Lymphocyte depletion kinetics/dicentrics/PCC | mild to moderate (1–48 h) | mild to severe bone marrow damage | 0–10% death |
3.5–7.5 | Lymphocyte depletion kinetics/PCC | severe (1–48 h) | pancytopenia, mild to moderate GI damage | 10–100% death within 2–6 weeks |
7.5–10.0 | Lymphocyte depletion kinetics/PCC | severe (<1 h–48 h) | combined BM and GI damage | 90–100% death within 1–3 weeks |
>10.0 | PCC | severe (minutes to <48 h) | GI, neurological, cardiovascular damage | 100% death (within 2–12 days) |
Several new approaches to biodosimetry are under investigation that utilize either changes in the transcriptome or proteome of peripheral blood in response to radiation. These may offer greater throughput, assuming the availability of equipment for processing large numbers of samples in parallel. For example, Meadows et al.86 developed gene expression signatures for radiation exposure in mice and demonstrated that genotype differences did not affect the accuracy of the signatures to predict radiation exposure. The signatures could completely distinguish the response to sepsis from radiation response. In addition, human peripheral blood signatures of radiation exposure and chemotherapy treatment differed and could identify patients exposed to radiation with 90% accuracy.
Historical Experience with Early Phase Acute Biodosimetry
Table 33.7 (adapted from Reference 49) lists selected radiation accidents where the dicentric and PCC biodosimetry techniques have played an important role in the clinical management of radiological casualties. More detailed summaries of some notable applications of the techniques are provided in the next section.71–79
Selected Use of Acute Phase Cytogenetic Assays in Radiation Accidents
Accident Location | Year of Accident | Number of People Exposed | Dicentrics (Chromosomal Abnormalities) | PCC |
---|---|---|---|---|
Cuidad Juarez, Mexico71 | 1984 | 7? | 7? | N/A |
Chernobyl, Russia72 | 1986 | 116,000 | 158 | N/A |
Goiânia, Brazil73 | 1987 | 250 | 129 | N/A |
Lilo, Georgia74 | 1986–1987 | multiple | 4 | N/A |
Kiisa, Estonia75 | 1994 | 4 | 4 | N/A |
Istanbul, Turkey (multiple cases)64 | 1995 | 21 | 21 | ?18 |
Tokaimura, Japan77–79 | 1999 | 3 | 1 | 3 |
unknown | 43 | |||
Meet Halfa, Egypt80 | 2000 | 7 | 5 | N/A |
Bangkok, Thailand81 | 2000 | 28? | 28 | 28 |
Ghent, Belgium82 | 2005 | 1 | 1 | 1 |
Referral Laboratory – incident summary73 | 2003–2005 | 23 | 18 | uncertain |
Referral Laboratory – incident summary83,84 | 1968–2003 | 996 | 996 | uncertain |
Cytogenetic Reference Standards68,69,83,84,85 | 2002–2014 | uncertain | ||
Acute Phase Dosimetry53,69,83,86 | 2002–2014 | uncertain |
Cytogenetic study results from the 1986 Chernobyl accident have been summarized.72 Investigators used chromosomal aberration dosimetry in the acute phase of the Chernobyl accident as a method of dose assessment. A good clinical correlation was observed between doses calculated based on chromosomal aberrations (dicentrics) and severity of ARS.
Soon after the Chernobyl accident, a radiation accident involving a 137Cs therapy source occurred in Goiânia, Brazil, in September 1987. More than fifty individuals received moderate to high doses (0.2–7 Gy) of gamma radiation. A cytogenetic technique (i.e., frequencies of dicentrics and rings in peripheral lymphocytes) was employed in the acute phase to estimate absorbed dose.73 Ramalho and Nascimento have described a follow-up study in which they found a two-log decline in the dicentric lymphocyte frequency. They reported an average disappearance half-time of lymphocytes containing dicentric and centric rings of approximately 130 days, which is significantly shorter than the value of 3 years usually cited in the literature.73
The radiation accident at Tokai-mura in 1999 is a famous and well-studied uranium criticality accident. It is an important event because it was witnessed, allowing careful reconstruction of the event, and because multi-parameter triage techniques were employed in the acute phase medical management of the victims. Despite the very high radiation doses received by two of the victims (approximately 8 and 20 Gy, respectively), the frequency of chromosome aberrations in circulating lymphocytes was found to be a reliable indicator of the absorbed radiation dose. Chromosome-painting techniques were found to be accurate in the evaluation of both dicentrics and translocations.77–79
Table 33.8 presents a comparison between various acute phase techniques for this criticality event. All table entries represent data contemporaneous with acute patient care and not from a retrospective analysis. Lymphocyte kinetics and the time to emesis were evaluated in real-time, and the results of chromosome biodosimetry were available quickly enough to impact clinical decisions when taken in the context of the patients’ evolving ARS. These different techniques provided useful and generally consistent dose estimates that allowed meaningful inferences about each patient’s prognosis. The proceedings from a Tokai-mura-related symposium is available that includes a retrospective improved analysis of the source term, power spectra, and medical treatment in this accident.87
Acute Phase Estimates of Dose (Gy) after the Tokai-mura Event (1999)
Method | Patient O | Patient S | Patient Y |
---|---|---|---|
Na-24 blood (n only) | 9.1 | 5.0 | 1.2 |
Rings + dicentrics | 21 | 6.6 | 2.8 |
PCC (γ equivalent) | >20 | 7.8 | 2.6 |
Na-24 WBC | 1.6 | ||
Lymphocyte kinetics | >10 | 6–10 | 1–4.5 |
Survival | Death 82 days post-exposure | Death 210 days post-exposure | Survival |
Electron Paramagnetic Resonance Physical Dosimetry
Electron paramagnetic resonance (EPR) or electron spin resonance spectroscopy is a technique for studying chemical species that have one or more unpaired electrons. Paramagnetic centers (molecules or atoms with unpaired electrons) are produced by the action of radiation on materials. The paramagnetic centers created by ionizing radiation are proportional to the absorbed dose and EPR can be used as a non-destructive probe of the structure and concentration of these paramagnetic centers. In the EPR measurement, irradiated materials are placed in a magnetic field of the appropriate frequency (typically in the GHz range). Electron spin transitions are induced by exposure to this field and then quantitated.
Electron paramagnetic resonance differs from nuclear magnetic resonance in that EPR measures excited electron spins rather than the spins of atomic nuclei. Most stable molecules have all their electrons paired and thus are not detected by EPR techniques, which are sensitive only to paramagnetic species. From the perspective of biological dosimetry, this limitation is actually an advantage in that ordinary chemical solvents and matrices do not give rise to EPR spectra. Thus, the EPR technique is one of great specificity. Bone and teeth have been found to provide the EPR signals of greatest stability, and so serve as natural physical dosimeters.
EPR dosimetry has been used primarily in the retrospective analysis of radiation accidents and is quite valuable in this regard. It is particularly helpful in events where an amputation has occurred and bone fragments are available from a site of severe local irradiation. These samples are often obtained after surgical amputation days to weeks post-accident. Table 33.9 presents selected cases where EPR has been useful in radiation accidents.
Selected Use of Acute Phase Electron Paramagnetic Resonance (EPR) in Radiation Accidents. A Radioactive Exposure Device (RED) is a bare radioactive source emitting external radiation
Place of Accident | Date | Type of Accident | Materials |
---|---|---|---|
United States88–92 | 1991 | Accelerator; various radiation accidents | EPR (bone; digits) |
San Salvador88 | 1991 | 60Co irradiator | EPR (bone; femur) |
Tammiku, Estonia91 | 1994 | RED | Thermoluminescence or TL (quartz pots) EPR (sugar samples) |
Georgia82 | 2001 | RED | EPR (bone; vertebra, ribs) |
Review of General and Combined Acute Phase Accident | 2005 | Overview of Acute Phase Dosimetry | |
Dosimetry53,78–81 |
EPR has received increased consideration as a health physics and medical tool for the acute phase analysis of radiation incidents. In the United States, various reports are available describing accelerator accidents and cases of severe, acute, local injury in which EPR dosimetry has been performed.88–93 A 1991 San Salvador accident involving a 60Co source was characterized by significant heterogeneity of exposure, with the highest doses being delivered to the feet and lower legs of the victims. Desrosiers presented a detailed EPR analysis of a femur available from that accident.92 Evaluation of the multi-casualty radiation accident in Lilo, Georgia, employed EPR techniques to analyze vertebra and rib samples removed from a victim for medical reasons to reconstruct the dose received by this individual.93
In the 1994 radiation accident in Tammiku, Estonia, three brothers stole a large 137Cs source from a poorly guarded radioactive waste depository and took it to their home. Various members of the family were exposed to this source in a chronic and non-uniform manner. In particular, the most severely injured patient received 1,830 Gy to the femur and thigh, and an approximately 4 Gy acute whole-body dose. He soon died of multi-organ failure. Other members of the family received a 0–4 Gy whole-body dose over 28 days and up to a 20–30 Gy acute local dose to the hands. This case is interesting because various acute phase modalities were employed in dose reconstruction: 1) chromosome aberration dicentric analysis; 2) Glycophorin A somatic mutation assays; 3) thermoluminescence dosimetry; 4) optically stimulated luminescence; 5) EPR; 6) chemiluminescence; and 7) Monte Carlo modeling of spatial effects. The use of EPR in this event was a valuable adjunct to clinical analysis of the ARS and of acute local injury.94,95
Acute Radiation Syndrome
General Considerations
ARS or “radiation sickness,” occurs when individuals are exposed over a short time period to high energy penetrating radiation with doses of ≥1 Gy to the whole body.99–101 Significant partial body exposures can also result in the development of ARS. ARS affects multiple organ systems. Symptoms associated with dysfunction of different organ systems will predominate at varying doses. The most frequently recognized components of ARS are the hematopoietic, gastrointestinal, and neurovascular syndromes, which result from cellular dysfunction or cell death within each of these tissue compartments. Cutaneous injury from trauma, radiation and/or thermal burns is also frequently encountered in radiation accidents. The so-called cutaneous radiation syndrome, where cutaneous injury is solely attributable to radiation exposure, represents a clinical entity distinct and separate from systemic ARS. In other cases, radiation exposure in conjunction with trauma or thermal injury will directly impact multiple organs, resulting in a “radiation combined injury.” This extremely complicated physiological state is associated with high mortality and the multi-organ failure syndrome. The complexities presented by individual patients notwithstanding, categorizing the syndromes is still useful, both for discussion purposes and because such categorizations enable the clinical team to identify the most life-threatening injuries and make better triage and management decisions. A recent review is available of ARS and irradiated victim triage in a military environment.100,101
In the absence of cutaneous or non-radiation related injuries, ARS follows a relatively predictable (or deterministic) course for each of its constituent syndromes. The hematopoietic syndrome is associated with the lowest threshold dose and the neurovascular syndrome is seen at the highest dose (Table 33.10). In general, the severity of ARS is directly proportional to dose, while the timing of symptom onset is inversely proportional. For example, ARS has a hematological threshold dose of about 0.7 Gy with severe reductions in blood counts occurring above 3 Gy.9 As previously noted, the LD50/60 for persons receiving no supportive care is about 3.5 Gy. This is primarily due to infection in the setting of neutropenia or hemorrhage in the setting of thrombocytopenia. The LD50/60 increases to 6–7 Gy with optimal supportive care (e.g., antibiotics, hematopoietic growth factors, and transfusions). Human mortality resulting from hematological insult has a peak incidence at about 30 days but continues through day 60. Since humans recover from hematological damage more slowly than other mammals, an LD50/60 (lethal to 50% of the population within 60 days of exposure) is used, in contrast to the LD50/30 for animals.39 A marked reduction in both the LD50/60 and the time from radiation exposure to death would likely occur following a nuclear detonation. This is due to the complex patterns of ARS, radiation combined injury, and cutaneous radiation syndrome that would occur.
Phases of Radiation Injury (Adapted from Reference 86)
Dose (Gy) | Prodromal Phase | Manifest Phase | Prognosis without Supportive Care |
---|---|---|---|
0.5–1.0 | Mild | Modest decline in blood counts | Survival |
1.0–2.0 | Mild to Moderate | Some bone marrow damage | Survival > 90% |
2.0–3.5 | Moderate | Moderate to severe bone marrow damage | Probable survival |
3.5–5.5 | Severe | Severe bone marrow damage; modest GI damage | Death within 3.5–6 weeks (50% of victims) |
5.5–7.5 | Severe | Pancytopenia and moderate GI damage | Death probable within 2–3 weeks |
7.5–10.0 | Severe | Severe GI and bone marrow damage | Death probable within 2 weeks |
>10.0 | Severe | Severe GI damage, radiation-induced lung injury, altered mental status; at higher doses (>20.0 Gy), cardiovascular collapse, fever, shock | Death within 2 weeks |
Clinical Progression
Prodromal Phase
In terms of its temporal progression, ARS is divided into four sequential phases: prodromal, latent, manifest (illness), and recovery or death. These stages are described in detail. As listed in Table 33.11, a variety of symptoms and signs that characterize the prodromal phase may result within minutes to hours after radiation exposure, depending on the dose received. These symptoms and signs can be divided into two main groups: gastrointestinal and neuromuscular. The gastrointestinal symptoms include vomiting, diarrhea, intestinal cramps, dehydration, and anorexia, while the neuromuscular symptoms include fever, sweating, headache, hypotension, apathy, and easy fatigability.100 The prodromal symptoms indicative of doses fatal to 50% of the population are nausea, vomiting, anorexia, and easy fatigability. The presence of initial fever, headache, immediate vomiting and diarrhea, hypotension, and/or disorientation after exposure portends a fatal outcome. As a guideline, persons who vomit within 2 hours of irradiation are at risk for at least moderate ARS. However, one should be cautious as it is difficult to distinguish radiation-induced vomiting from emesis due to psychological factors resulting from environmental stressors.
Prodromal Phase: Severity/Dose and Medical Response
Signs/Symptoms after Exposure | Mild (1–2 Gy) | Moderate (2–4 Gy) | Severe (4–6 Gy) | Very severe (6–8 Gy) | Lethal* (>8 Gy) |
---|---|---|---|---|---|
Vomiting | |||||
Onset | ≥2 h | 1–2 h | <1 h | <30 min | <10 min |
Incidence (%) | 10–50 | 70–90 | 100 | 100 | 100 |
Diarrhea | None | None | Mild | Heavy | Heavy |
Onset | – | – | 3–8 h | 1–3 h | <1 h |
Incidence (%) | – | – | <10 | >10 | ∼100 |
Headache | Slight | Mild | Moderate | Severe | Severe |
Onset | – | – | 4–24 h | 3–4 h | 1–2 h |
Incidence (%) | – | – | 50 | 80 | 80–90 |
Temperature | Normal | Increased | Fever | High fever | High fever |
Onset | – | 1–3 h | 1–2 h | <1 h | <1 h |
Incidence (%) | – | 10–80 | 80–100 | 100 | 100 |
Consciousness | Normal | Normal | Normal | Possibly altered | Unconscious |
Onset | – | – | – | Sec to min | |
Incidence (%) | – | – | – | 100 (>50 Gy) | |
Medical Response | Outpatient | Observation or treatment at a specialized hospital if needed | Treatment at a specialized hospital | Treatment at a specialized hospital | If dose <10–12 Gy consider treatment ≥12 Gy palliative |
* Individuals may survive with exposures as high as 12 Gy with appropriate medical management for greater than 6 months. Modified from the International Atomic Energy Agency, Diagnosis and Treatment of Radiation Injuries, Safety Report Series No. 2, Vienna; 1998.
Latent Phase
The latent stages of ARS (Table 33.12) are characterized by a relatively asymptomatic period. With a 2–3 Gy exposure, the prodromal symptoms abate after a few days and the latent period ensues for 2–3 weeks with continued declines in lymphocytes, neutrophils, and platelets. When the dose is high enough to induce the gastrointestinal and neurovascular forms of ARS, the latent phase is shortened or frequently eliminated, respectively.
Latent Phase
Signs/Symptoms after Exposure | Mild (1–2 Gy) | Moderate (2–4 Gy) | Severe (4–6 Gy) | Very severe (6–8 Gy) | Lethal* (>8 Gy) |
---|---|---|---|---|---|
Latency period (d) | 21–35 | 18–28 | 8–18 | ≤7 | None |
Lymphocytes | |||||
(109 cells/L), days 3–6 | 0.8–1.5 | 0.5–0.8 | 0.3–0.5 | 0.1–0.3 | 0.0–0.1 |
Granulocytes | >2.0 | 1.5–2.0 | 1.0–1.5 | ≤0.5 | ≤0.1 |
Diarrhea | None | None | Uncommon | Days 6–9 | Days 4–5 |
Epilation (d) | None | Moderate, ≥15 | Moderate, 11–21 | Complete, <10 | Complete, <10 |
Medical response | Outpatient | Hospitalization recommended | Hospitalization required | Hospitalization required | Hospitalization required, palliative treatment if ≥12 Gy |
* Individuals may survive with exposures as high as 12 Gy with appropriate medical management for greater than 6 months. Modified from the International Atomic Energy Agency, Diagnosis and Treatment of Radiation Injuries, Safety Report Series No. 2, Vienna, 1998.
Manifest (Illness) Phase
During this stage (Table 33.13) the tissue compartments that are damaged become dysfunctional, thereby dictating the form of ARS. At very high doses (e.g., 100 Gy) all organ systems are severely compromised and death quickly ensues from neurovascular dysfunction.
Manifest (Illness) Phase
Signs/Symptoms after Exposure | Mild (1–2 Gy) | Moderate (2–4 Gy) | Severe (4–6 Gy) | Very severe (6–8 Gy) | Lethal* (>8 Gy) |
---|---|---|---|---|---|
Onset (d) | 21–35 | 18–28 | 8–18 | ≤7 | 0 |
Lethality (%) | 0 | 0–50 | 20–70 | 50–100 | ∼100 |
Onset (weeks) | – | 6–8 | 4–8 | 1–2 | ≥1 day to 2 weeks |
Clinical Manifestations | |||||
Fatigue | Yes | Yes | Yes | Yes | Yes |
Epilation | – | Yes | Yes | Yes | Yes |
Infection | – | Yes | Yes | Yes | Yes |
Bleeding | – | Yes | Yes | Yes | Yes |
Shock | – | – | – | – | Yes |
Coma | – | – | – | – | Yes |
Lymphocytes (109 cells/L) | 0.8–1.5 | 0.5–0.8 | 0.3–0.5 | 0.1–0.3 | 0.0–0.1 |
Platelets (109 cells/L) | 60–100 | 30–60 | 25–35 | 15–25 | <20 |
Medical Response | Outpatient | Hospitalization recommended | Hospitalization required | Hospitalization required | Hospitalization required, palliative treatment if ≥12 Gy |
* Individuals may survive with exposures as high as 12 Gy with appropriate medical management for greater than 6 months. Modified from the International Atomic Energy Agency, Diagnosis and Treatment of Radiation Injuries, Safety Report Series No. 2, Vienna, 1998.
Recovery or Death
Recovery or death follows the manifest (illness) phase. At higher doses, the time to recovery can be prolonged, with substantial residual deficits due to late fibrosis and other complications. Patients receiving high doses may experience other delayed effects of acute radiation exposure, such as radiation pneumonitis, radiation nephropathy, cataracts, and cognitive decline.39,99,100
Acute Hematopoietic Syndrome
The hematopoietic syndrome is usually encountered with doses that exceed 2 Gy, although the dose thresholds may be lower under compromising conditions, such as significant cutaneous damage.105,108 This syndrome follows the four well-characterized sequential phases described previously. The prodromal symptoms are nonspecific and include nausea, vomiting, and anorexia. Rapid declines in lymphocytes herald the onset of full-blown hematopoietic syndrome. A latent period follows over 1–2 weeks with continued declines in the peripheral blood cell counts, possibly resulting in infection and hemorrhage during the manifest phase.
Hematopoietic cells are among the most radiosensitive cells in the body. Mitotically active precursor cells are substantially reduced after a 2–3 Gy dose, resulting in a decreased supply of red blood cells, white blood cells, and platelets. At these doses, the supply of mature cells from the diminished precursor pools may be insufficient to maintain an adequate number for proper physiologic function, thereby resulting in the cytopenias characteristic of the hematopoietic syndrome. Certain subpopulations of the precursor cells are more radioresistant, presumably because the cells are in the non-cycling (G0) or radioresistant stage (late S) of the cell cycle.109 This population may play a vital role in hematological reconstitution with exposures as high as 7–8 Gy. Fortunately, most individuals involved in radiation accidents receive inhomogeneous exposures due to: 1) the radiation mixture (e.g., photons, beta or alpha particles); 2) the energy of the radiation (i.e., penetrating or not); 3) the individual’s distance from the source; 4) the physical environment; and/or 5) the degree of internal or external contamination that occurs. As a consequence, persons receiving potentially lethal doses of radiation may still survive due to sparing of small areas of bone marrow, especially if located in the central axial skeleton, that can serve as a reservoir for the rapid reestablishment of hematopoiesis.110
The rates of decline for the various circulating cells depend on the sensitivity of the cell type (i.e., stem, precursor, and fully differentiated cells) and their turnover time. Lymphocytes decline the most rapidly, while platelets and other leukocytes are depressed less quickly. Having the longest circulating half-life and being resistant to apoptosis, erythrocytes demonstrate the slowest decline. Thus, the acute hematopoietic syndrome predisposes an individual to infection, hemorrhage, and anemia that follow the decline in leukocytes, platelets, and erythrocytes from as early as 5–10 days to several weeks after a high-dose exposure.111 Due to the long circulating half-life of erythrocytes, the body’s compensation mechanisms, and the general availability of transfusions, anemia is seldom life threatening unless other trauma or bleeding results secondary to thrombocytopenia.
Lymphocytes demonstrate a somewhat unusual response to radiation. Terminally differentiated cells (e.g., rhabdomyocytes) are usually more radioresistant than intermitotic cells (e.g., intestinal crypt cells, erythroblasts). However, lymphocytes, which are long-lived and highly differentiated, are nonetheless significantly radiosensitive and undergo rapid apoptosis when exposed to comparatively low doses of radiation. Therefore, lymphopenia occurs more rapidly than the other cytopenias, and assuming no other insult, a predictable dose-dependent decline is expected after radiation. For example, a potentially lethal radiation dose is characterized by a 50% drop in lymphocyte count within the first 24 hours followed by a more severe decline over the next day.108,110,112
Neutrophils are part of the innate immune system and are the first responders to infection. Thus, they are the most critical blood cell type in combating an acute threat from microorganisms. Circulating neutrophils have a half-life of about 7 hours before entering the tissue pools where they survive for an additional 1–2 days. In cases of infection, these cells are recruited from the circulation into the tissue and consumed, resulting in a marked reduction in their half-life. Maturation of neutrophil precursors to mature neutrophils in the bone marrow normally takes about 2 weeks. Following irradiation, declines in circulating neutrophils result from depletion of the marrow reserves of mature cells and death of rapidly dividing, early progenitor cells. Thus, the loss of progenitor cells and the unusual kinetics of neutrophil production and release account for the delayed onset of the hematopoietic syndrome.39,108 To complicate matters, a transient increase in the granulocyte count may occur due to remobilization from the venous, splenic, and bone marrow pools with exposures of less than 5 Gy. A second abortive rise or stabilization in the granulocytes counts may occur in days 5–10 post-exposure, reflecting the production and release of granulocytes from residual hematopoietic tissue. This abortive rise in the peripheral granulocyte counts may suggest a survivable exposure.
The loss of progenitor cells with radiation exposure also results in a decrease in platelets, which have a mean survival time of 8–11 days. The resultant thrombocytopenia contributes to hemorrhage that occurs with the hematopoietic syndrome. Most authorities recommend platelet transfusion: 1) to reduce the risk of spontaneous hemorrhage when platelet counts fall below 10 × 109 cells/L in asymptomatic patients; and 2) for counts in the 10–50 × 109 cells/L range if there is clinical bleeding or if invasive procedures are anticipated. The final component of the hematopoietic syndrome, anemia, is characterized by a hemoglobin mass of less than 10 g/dl. The long mean lifespan of red cells (which approaches 120 days) makes anemia less of an immediate problem in the hematopoietic syndrome than the other cytopenias.
Acute Gastrointestinal Syndrome
The gastrointestinal (GI) syndrome also has four sequential stages but occurs at higher radiation doses than the hematopoietic syndrome (typically becoming manifest at total body irradiation doses of ≥ 6 Gy). The full syndrome will usually occur with a dose greater than approximately 10 Gy although some symptoms may occur as low as 6 Gy. The GI mucosa is a self-renewing tissue; the morbidity and mortality observed in the GI syndrome reflect the destruction of this mucosal lining in the setting of concurrent myelosuppression. The prodromal stage is characterized by prompt nausea, vomiting, and diarrhea, which are typically more severe than the symptoms observed with the hematopoietic syndrome due to the higher initiating radiation doses. In some cases, this may be followed by a latent period lasting several days, although the duration of latency declines as the exposure dose increases. The manifest stage then follows with severe diarrhea, nausea, vomiting, and fevers. Other systemic effects may include dehydration, ileus, malabsorption, electrolyte derangements, gastrointestinal bleeding, renal impairment, and eventual cardiovascular collapse. As with the hematopoietic system, the dividing precursor cells are more radiosensitive than the differentiated cells. The radiosensitive epithelial stem cells are confined to the crypts and provide a continuous supply of new cells. These new cells differentiate as they move up the villi or luminal surface to become functionally mature cells, which are then extruded. Hence, sufficient radiation sterilizes the dividing crypt cells, eventually disrupting the mucosal barrier resulting in septicemia and usually death. Kolesnick, Fuks, and colleagues have argued that endothelial damage is the primary lesion regulating crypt cell survival and intestinal injury, but this hypothesis remains controversial.113
Acute Neurovascular Syndrome
Neurovascular syndrome may be observed at acute doses of greater than 10–20 Gy and is thought to reflect cerebral edema and cardiovascular collapse, although hypotension may also be seen at lower doses. The full syndrome will usually occur with a dose greater than approximately 50 Gy although some symptoms may occur as low as 10 Gy. Death occurs within 3 days and is due to collapse of the circulatory system as well as increased intracranial pressure from edema due to vasculitis and meningitis. As with the hematopoietic and gastrointestinal syndromes, the prodromal phase is characterized by nausea, vomiting, and diarrhea, but this typically occurs within minutes of exposure in persons suffering acute neurovascular syndrome. Disorientation, confusion, and prostration are characteristic of the prodromal phase. Loss of balance and seizures may also occur. Papilledema, ataxia, and reduced or absent deep tendon and corneal reflexes may be noted during the physical examination. This phase is followed, possibly without a latent period, by a severe manifest phase of fever, respiratory distress, disorientation, ataxia, persistent diarrhea, seizures, cardiovascular collapse, and coma. The course is inexorable and death invariably follows within a few days. The clinical course of rapid deterioration mimics that of acute sepsis and septic shock, both of which must also be considered in the differential diagnosis.
Acute Cutaneous Radiation Injury (≤ 90 days)
The skin is composed of the epidermis and dermis. The epidermis provides a durable waterproof protective barrier of stratified squamous epithelium between the body and external environment. The stratum germinativum (or basal layer), containing the basal stem cells, is the innermost layer of the epidermis. Cells produced in the basal layer differentiate and migrate toward the surface, where they maintain some proliferating potential in the stratum spinosum (squamous layer). The cells then pass through additional layers where they finally make their way to the stratum corneum, where they eventually slough off. The turnover time ranges from 4–7 weeks. The dermis, connected to the epidermis by a basement membrane, contains a dense network of connective tissue, hair follicles, capillaries, lymphatics, sweat glands, nerve endings, sebaceous glands, and apocrine glands. The highly radiosensitive epithelial stem cells, follicular, sebaceous, and sweat germinal cells provide a continuous supply of new cells to their respective structures. These new cells differentiate as they become functionally mature cells and are eventually lost. With irradiation, a large proportion of these cells may die. Unless replenished, the loss of these cells will result in disruption of skin integrity, epilation, dry skin, and if the dose is high enough, failure of thermal regulation. The late effects of radiation are mainly attributable to endothelial cell death within the papillary vasculature and are discussed in detail elsewhere.116–118
Significant cutaneous radiation injury independently predicts lethality, thus increasing the risk of death when combined with other trauma.119,120 Cutaneous radiation injury will usually be combined with other aspects of ARS but it may occur in isolation if the exposure is restricted to low-energy X-rays or beta radiation. Mechanical, chemical, and thermal injuries may accelerate and exacerbate the cutaneous radiation injury. In cases where multiple mechanisms have contributed to the skin damage, a more appropriate term for the clinical syndrome encountered might be “combined skin injury.” Moreover, the damage to the skin is almost invariably inhomogeneous due to factors such as the position of the individual in relationship to the radiation source and/or the presence of physical barriers providing partial body protection.
The effects of radiation on the skin are dose, depth, and volume dependent. Most individuals receiving a radiation dose to the skin of 5 Gy or higher will experience a transient skin reaction of erythema, edema, itching, and/or tingling within 24 hours of exposure. This prodromal period is followed by a latent period of 2–3 weeks. Next, an orderly progression occurs of erythema, hyperpigmentation, and finally dry followed by wet desquamation if the focal dose to skin is approximately 15–24 Gy. In the case of extremely high doses (≥ 50 Gy), the period of latency may not occur and the injury may progress from erythema to necrosis within days (Table 33.14).117 Figure 33.5 demonstrates the progressive stages of cutaneous injury in a patient receiving a focal high-dose exposure to the right hand. Clinicians should remember to include radiation injury as part of the differential diagnosis of desquamation or ulceration of unclear etiology, particularly when the patient lacks a history of a burn injury.
Skin Injury and Time of Onset
Stage/symptoms* | Dose range (Gy) | Time of Onset (d)* |
---|---|---|
Erythema | 3–10 | 14–21 |
Epilation | >3 | 14–18 |
Dry desquamation | 8–12 | 25–30 |
Moist desquamation | 15–20 | 20–28 |
Blister formation | 15–25 | 15–25 |
Ulceration (without skin) | >20 | 14–21 |
Necrosis | >25 | >21 |
* The time to progression of each stage (erythema, epilation, dry desquamation, etc.) is shortened with increasing dose (not shown). Modified from the International Atomic Energy Agency, Diagnosis and Treatment of Radiation Injuries, Safety Report Series No. 2, Vienna, 1998.

Progression of skin lesions in a patient receiving a focal high-dose exposure to the right hand. (a) Minutes after exposure, (b) approximately 12 hours post-exposure, (c) day 25 post-exposure, (d) day 34 post-exposure, and (e) day 46 post-exposure.
Radiation Combined Injury
In many potential scenarios, burns and/or wounds in combination with radiation are highly likely. It is estimated that 60–70% of persons exposed to significant radiation doses from the atomic bombings of Hiroshima and Nagasaki also sustained traumatic injury. Similarly, in the Chernobyl accident, approximately 10% of the 237 acutely exposed first responders received both significant radiation doses and burns.119 The combination of radiation exposure with other injuries results in high lethality, whether the etiology is blast, burn, trauma, or infectious. The LD50/30 and the time from exposure to death for irradiated animals at a given dose both decrease significantly in the setting of combined injury.120 This effect has been observed for the combination of radiation injury with burns, wounds, and experimentally induced infections across multiple species. Data derived from studies involving the combination of sublethal radiation exposure and thermal injury are summarized in Table 33.15 and are representative of findings with other permutations of radiation combined injury.
Data Summarized from the Literature by the Armed Forces Radiobiology Research Institute (AFRRI)
Model | Injury | Mortality |
---|---|---|
20% burn | 12% | |
Dog | 100 cGy total body irradiation | 0% |
combined burn + total body irradiation | 73% | |
10–15% burn | 0% | |
Pig | 400 cGy total body irradiation | 20% |
combined burn + total body irradiation | 90% | |
31–35% burn | 50% | |
Rat | 250 cGy total body irradiation | 0% |
combined burn + total body irradiation | 95% | |
1.5% burn | 9% | |
Guinea pig | 250 cGy total body irradiation | 11% |
combined burn + total body irradiation | 38% |
Delayed Effects of Acute Radiation Exposure
In persons surviving the initial phases of ARS, or receiving substantial partial-body exposures, tissue and organs other than those mentioned previously can manifest late radiation effects, referred to collectively as the delayed effects of acute radiation exposure (DEARE). For example, persons exposed to high doses of radiation (typically in excess of 6–8 Gy) often develop impairment of lung or kidney function, starting approximately 3 months after the exposure event. Acute, subacute, and chronic radiation syndromes may present as a clinical continuum or there can be a prolonged latency between exposure and the manifestation of radiation-induced organ dysfunction. Although the hematopoietic and gastrointestinal syndromes are largely attributable to the direct cytotoxic effects of radiation on rapidly dividing tissues, DEARE are thought to reflect chronic inflammation induced by vascular damage or other injury to the connective tissue. The interested reader may refer to several reviews on this topic for additional information.121–124
Radiation-Induced Malignancy
Individuals exposed to doses of radiation that do not produce immediate acute effects are still likely to be concerned about their risk of developing radiation-induced cancer. In general, the risk of secondary cancer increases with increasing dose, although outcomes for specific individuals cannot be predicted with any certainty. This is in contrast to organ injury, where the severity of dysfunction increases with increasing dose once the threshold dose for injury is surpassed. Among atomic bomb survivors, the observed excess relative risk was greatest for the development of hematologic malignancies (primarily acute myeloid leukemia), with incidence peaking approximately 10 years after exposure.124,125 Long-term follow-up of participants in the lifespan study cohort of Hiroshima and Nagasaki atomic bomb survivors has shown that excess risk for solid cancers exhibits significant variation with gender, attained age, and age at the time of exposure. According to the latest summary report, it was estimated that solid cancer rates increase by about 35%/Gy for men and 58%/Gy for women, at age 70 after exposure at age 30. In addition, statistically significant radiation-associated increases in risk are seen for cancers of the oral cavity, esophagus, stomach, colon, liver, lung, skin (non-melanoma), breast, ovary, bladder, nervous system, and thyroid.125
In the future, it may be possible to estimate an individual’s risk of radiation-induced cancer more precisely by assessing the radiation exposure in light of other pertinent factors, such as the volume of tissue irradiated, the individual’s history of exposure to other carcinogens (e.g., smoking), and the victim’s age and family history. At present, however, there is no way to reduce an individual’s risk of radiation-induced cancer after the radiation exposure has occurred.
Treatment of Acute Radiation Syndrome
Medical Management of Acute External Radiation Syndrome
Treatment for ARS in the absence of combined injury is usually required only in those who have received radiation doses ≥ 2 Gy.99 Patients who present with combined injuries due to mechanical, thermal, and/or chemical causes should be triaged as described elsewhere in the chapter and managed using relevant burn or trauma protocols. Since radiation is not immediately life-threatening, the non-radiation-related injuries should be addressed first. The management of acute radiation injury depends on multiple factors including the location of exposure (external or internal), the extent of exposure (partial or whole body), the dose and type of radiation, concurrent injuries or illnesses, age and weight of the patient, pregnancy status, and the particular radionuclides involved in the case of internal contamination.
Infection is a major cause of death in patients with ARS. Therefore, supportive care including wound care, antimicrobial treatment and prophylaxis for infection, and mitigation of neutropenia with myeloid cytokines and possibly stem cell transplantation all play vital roles in proper management.130–137 Several cases of high-dose exposure illustrate that restoration of bone marrow function may be possible even after exposures as high as 10–12 Gy with aggressive supportive care. Hematopoietic recovery has not translated into long-term survival, however, due to progressive gastrointestinal and pulmonary dysfunction.11 As a result, it is appropriate to withhold aggressive treatment for victims who have received a supralethal dose of radiation, especially in a setting of mass casualties, where resource allocation constraints may be significant.99,101,131–137 Exactly what constitutes a supralethal dose remains contentious, but whole body doses over 12 Gy have universally been fatal.
Radiation injury management can be divided into three categories: acute (≤ 72 hours), intermediate (3–30 days), and late (> 30 days). A discussion follows highlighting the management of radiation injury during the acute and intermediate periods.
Acute Management
Both external and internal decontamination should be performed along with medical and surgical stabilization as soon as possible (methods of internal decontamination are discussed later). External decontamination is achieved for most victims by removal of clothing and showering with soap and water. Achieving a reading of no more than two times background levels of radiation on a survey instrument indicates effective decontamination. The presence of persistent radioactive material should not delay critical medical interventions. Additional information on decontamination can be found in Chapter 16.
Prodromal symptoms such as nausea, vomiting, diarrhea, and headache may be controlled with conventional agents such as 5-hydroxytryptamine (5-HT3) receptor antagonists (ondansetron) and fluids, loperamide, and acetaminophen (paracetamol), respectively. All blood products should be leukoreduced and irradiated to minimize transfusion-associated graft-versus-host disease (GVHD). Not only can GVHD cause significant morbidity and mortality, it can also pose a clinical dilemma as some of the signs and symptoms are similar to those of severe ARS (e.g., diarrhea, fever, hyperbilirubinemia, and pancytopenia).
Table 33.16 outlines general recommendations for pharmacologic management of hematologic ARS. Similar guidelines have been published elsewhere and reflect differences in appropriate care based on the size of the incident.126–130,132 Patients susceptible to severe life-threatening infections should receive prompt antibiotic treatment on an empirical basis if they show clinical evidence of infection. In casualties who have become neutropenic (peripheral blood absolute neutrophil count less than 0.5 × 109 cells/L) but do not have evidence of active infection, it is appropriate to administer acyclovir for prophylaxis against herpes simplex virus. Based on guidelines for patients with cancer receiving chemotherapy from the National Comprehensive Cancer Network (www.nccn.org) and Infectious Disease Society of America, patients who are expected to have neutropenia lasting longer than 3–5 days may also benefit from prophylaxis against bacteria and Candida.132 This can be accomplished with the combination of a fluoroquinolone such as levofloxacin and a triazole such as fluconazole. These agents can all be discontinued upon the resolution of neutropenia.
Treatment Guidelines for Radiation Exposure Based on Estimates of Whole-Body Dose
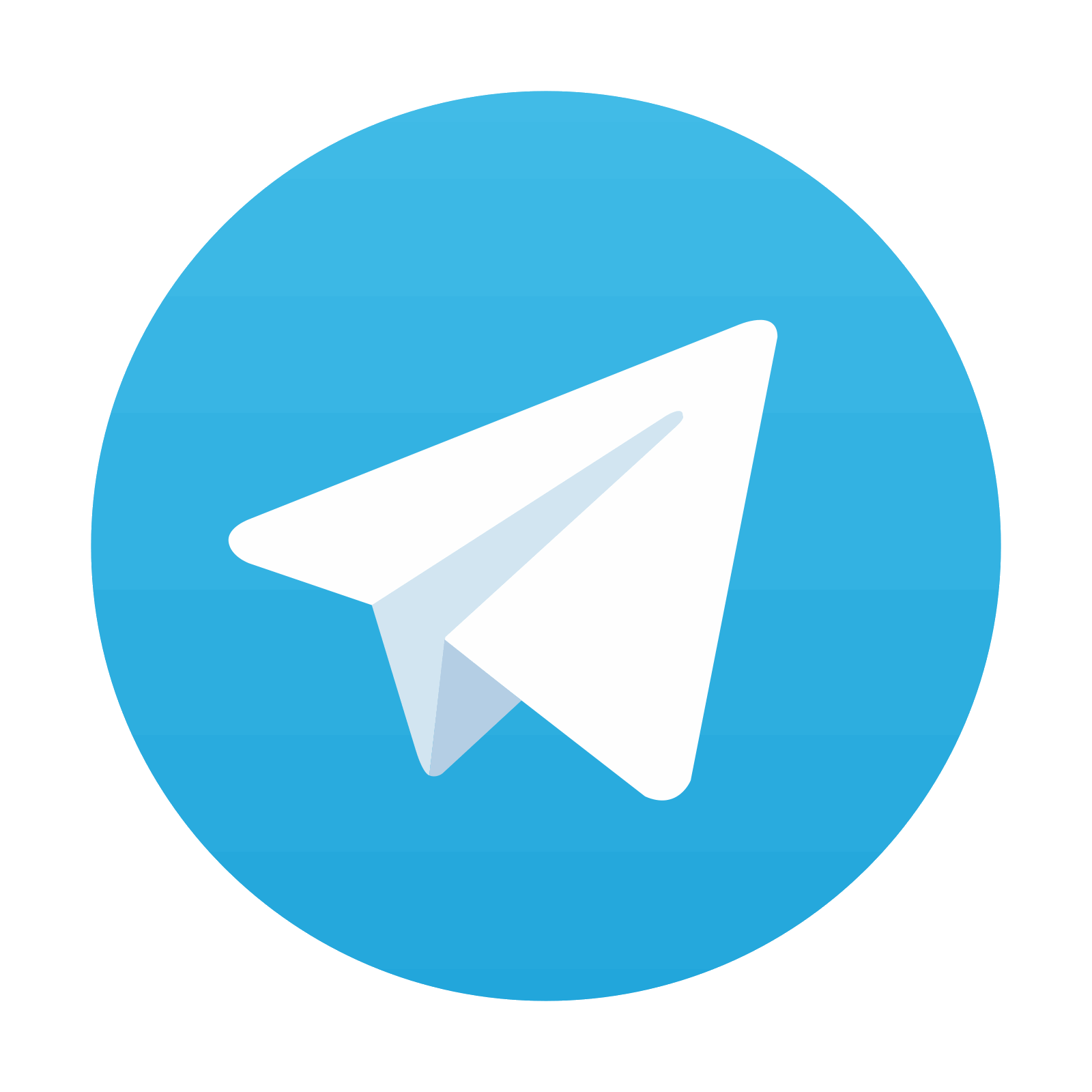
Stay updated, free articles. Join our Telegram channel
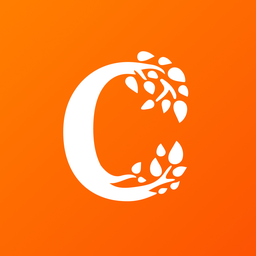
Full access? Get Clinical Tree
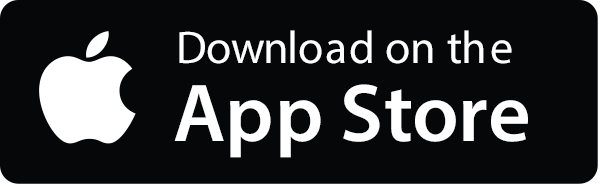
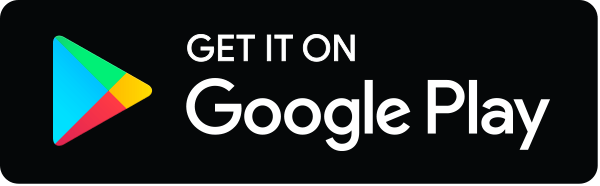
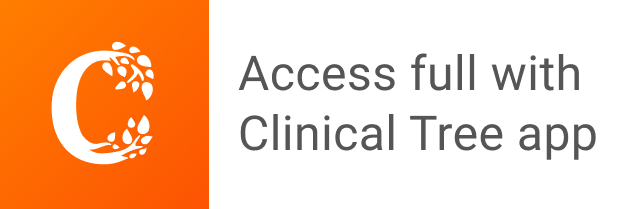