Doppler color flow imaging has evolved as the method of noninvasively imaging blood flow through the heart by displaying flow data superimposed on the two-dimensional (2D) echocardiography image. Although 2D echocardiography provides structural or anatomical details, Doppler imaging (spectral, color flow, and tissue Doppler) is extensively utilized for physiological and hemodynamic information, thus allowing identification of valvular, congenital, and other forms of heart disease.
Doppler echocardiography provides an accurate assessment of speed and direction of motion of blood and myocardial tissue utilizing the Doppler effect. Therefore, it allows assessment of valves; quantification of stenosis or regurgitation; and calculation of filling pressures, cardiac output, and intracardiac shunts. It has largely replaced conventional cardiac catheterization for many such hemodynamic assessments.
The Doppler effect is characterized by a change in the apparent frequency of a wave as a result of relative motion between the observer and the source. It is attributed to Johann Christian Doppler, an Austrian mathematician and scientist, who in his 1842 paper titled, “On the Colored Light of the Binary Stars and Some Other Stars of the Heavens,” was the first to notice the colored appearance of stars, which emitted blue light while moving toward the earth and red light while moving away from earth. Thus, he postulated that the observed frequency of a wave depends on the relative speed of the source and the observer. Although Doppler himself never extended the principle to other natural phenomena, the Doppler effect can be observed for any type of wave, including water waves, sound waves, light waves, and electromagnetic waves.
The Doppler effect can be illustrated by a common phenomenon: when a vehicle sounding a siren or horn approaches, passes, and recedes from an observer, the pitch of the siren sound (a measure of the siren’s frequency) varies as high pitch when approaching, actual pitch at the point of passing, and low pitch after the car passes the observer. The relative changes in frequency can be explained as follows: when the source of the sound waves is moving toward the observer, each successive wave is emitted from a position closer to the observer than the previous wave, reducing the time taken to reach the observer. As a consequence, the distance between successive wave fronts is reduced leading to clustering, or “bunching together,” and resulting in an increase in the frequency. Conversely, if the source of waves is moving away from the observer, each wave is emitted from a position farther from the observer than the previous wave, increasing the arrival time between successive waves, thus “spreading them out” and reducing the frequency. Similarly, when evaluating blood flow, the frequency (cycles/second) of the reflected sound waves increases when the red blood cells are moving toward the transducer and decreases when red blood cells are moving away from the transducer (Fig. 5-1).
For waves that propagate in a medium, such as sound waves, the velocity of the observer and of the source is relative to the medium in which the waves are transmitted. The total Doppler effect may therefore result from motion of the source, motion of the observer, or motion of the medium. Each of these effects is analyzed separately. For waves, which do not require a medium, such as light, only the relative difference in velocity between the observer and the source needs to be considered.
If the sound waves emitted by the transducer at the frequency f(t) strike a stationary object in their path, they are reflected back to the receiving transducer at a frequency f(r) that remains unaltered and no Doppler shift will be observed, i.e., f(t) = f(r). If the object is moving toward the transducer, f(r) is increased [i.e., f(t) < f(r)], but if the object is moving away from the transducer, the f(r) is reduced [i.e., f(t) > f(r)]. In terms of actual quantification, the difference in frequency between the transmitted frequency f(t) and received frequency f(r) is known as the Doppler shift = Δf = f(r) – f(t). The magnitude of the frequency shift is proportional to the frequency of the emitted sound waves. The higher the transmitted frequency, the larger the frequency shift. Doppler shift is directly related to the velocity of the object (i.e., red blood cell) encountered by the ultrasound beam. The relationship of the Doppler shift with velocity is described by the Doppler equation1:
V = Velocity (of red blood cells)
θ = Angle between the Doppler beam and the direction of blood flow
C = Speed of ultrasound in tissue/blood (1540 m/s)
Modern ultrasound systems measure the Doppler shift and calculate the velocity information using the Doppler equation.
The angle between the ultrasound beam and the direction of blood flow (angle of insonation) can introduce error in estimating velocities. For θ angles of 0 and 180 degrees (parallel with blood flow), cosine θ = 1 and the Doppler shift is most accurately measured. But with increases in θ there is a progressive decrease in the measured Doppler shift. For θ of 60 degrees, cosine θ = 0.50. When the Doppler beam is perpendicular to the direction of flow, the Doppler shift is recorded as zero because the cosine of 90 degrees is zero and no blood flow is detected. Misalignment of interrogating beam leads to underestimation, but never an overestimation, of the true velocity. However, underestimation of Doppler shift remains clinically insignificant until θ exceeds 20 degrees and the associated percentage error exceeds 7% (Fig. 5-2).2 It is therefore essential to align the Doppler beam as parallel to the direction of blood flow as possible for an accurate measurement of speed and direction of flow. The value of θ is particularly important for accurate assessment of high-velocity jets.
After obtaining the required 2D echocardiographic view of where the Doppler shift has to be measured, the cursor of the Doppler beam is oriented on the 2D image parallel to the direction of blood flow to obtain accurate estimates of velocity (Fig. 5-3). When the reflected wave (backscattered signal) is received by the transducer, the difference in frequency between the transmitted and backscattered signal is determined using fast Fourier transformation (FFT).1 The Doppler information is then displayed as distinct envelopes known as the “spectral display” on the monitor. Time is displayed over the x-axis and velocity over the y-axis. If the returning frequency is higher than the transmitted frequency, it is referred to as a “positive Doppler shift” (blood moving toward the transducer) and is displayed above the baseline. If the returning frequency is lower than the transmitted frequency, it is called a “negative Doppler shift” (blood moving away from the transducer) and is displayed below the baseline.
All ultrasound systems are equipped to create both a spectral display and an audio component of the beat-to-beat Doppler data during acquisition. The loudness and pitch of this audio signal varies with the strength and quality of the Doppler data, and experienced echocardiographers have been known to use the audio component alone to acquire and record the maximal velocity. The loudness/pitch of the audio signal can also be used to qualitatively diagnose the presence of stenosis; however, the information obtained from audio analysis is qualitative and subjective and should not be used as the sole means of quantification.1
It is important to remember that a simultaneous display of the two-dimensional (2D) image and Doppler information requires a time-share arrangement between two independent functions of the transducer. In the case of a continuous spectral display, the 2D image display is rapidly switched on and off, giving the impression of a continuous image alongside the spectral display. Thus, there is always some reduction in the quality of 2D and Doppler data when a simultaneous imaging mode is used.1
Doppler can be used either in “pulse” or “continuous” mode to interrogate intracardiac flow patterns. Both these modalities have strengths and weaknesses that are suited for specific situations.
Pulsed-wave Doppler (PWD) utilizes a single ultrasound crystal that alternates between transmission and reception. In PWD repetitive short bursts of ultrasound at a specific frequency (pulse repetition frequency or PRF) are emitted. It can provide Doppler data from a specific site along the ultrasound beam, referred to as the “sample volume” or “sample gate.” The operator determines the location of the sample volume. The signals returning to the transducer are interpreted on the basis of the time taken for them to return to the transducer, a process referred to as “time-gating” and described by the following mathematical relationship: Time Delay = 2d/C (d = Depth, C = Speed of sound in blood). Sufficient time must be allowed for the signal to travel twice the distance (d), i.e., from the transducer to the sample volume and back. After transmitting a sound pulse, the transducer waits the exact time required for the signal to travel to and from the sample volume before briefly listening for a reflection. If a reflection is received, it has been created at the location of the sample volume. All other returning ultrasound information is ignored. PWD thus has the advantage of being “site specific” (Fig. 5-4). Simultaneous display of the location of the sample volume and the spectral Doppler data is also possible with some sacrifice of the quality of the 2D image as well as the Doppler signal. The distance from the transducer determines the size and three-dimensional shape of the sample volume. The farther the sample volume is placed from the transducer, the larger it becomes due to progressive divergence of the ultrasound beam from the transducer.
FIGURE 5–4.
PWD allows the operator to measure a specific site by manually positioning the sample volume. CWD measures the highest velocity in the path of the ultrasound beam, thus suffering from “range ambiguity,” or the inability to discriminate the precise location of the highest recorded velocity. The velocity envelopes obtained with CWD are typically shaded, representing the multiple velocities measured in the course of the ultrasound beam.

However, PWD has a major disadvantage in that it cannot accurately assess velocities greater than 1.5 to 1.7 m/s, generally encountered in clinically important valvular lesions. To understand the reasons, we have to first address the “aliasing” phenomenon. Because PWD utilizes a single transducer, there is a maximum limit to the frequency shift (and thus maximum velocity) that can be measured unambiguously. This limit is referred to as the Nyquist limit and is equal to one-half the pulse repetition frequency. When this maximal frequency shift is exceeded, aliasing or a wrapping around of the velocities (i.e., the top of the waveform appearing in the reverse part of the scale) is seen on the spectral Doppler display. “Aliasing” is analogous to the sudden apparent reversal of direction of the spokes of a wheel, when the velocity of the wheel spokes exceeds the sampling rate of the observer (e.g., human eye) (Fig. 5-5). Both frequency of the transducer and depth determine the velocities at which aliasing occurs. Lower frequency transducers result in lower Doppler shifts, which are less likely to exceed the Nyquist limit. The PRF and therefore the Nyquist limit or maximum velocity that can be accurately measured are directly related to the depth of placement of the sample volume. The greater the depth of the sample volume, the longer the time it takes for the sound to travel back to the transducer; therefore, the lower the PRF and the lower the maximum velocity that can be measured at that location. In order to measure higher velocities, the baseline can be shifted to display the entire spectral display waveform in one direction. However, for velocities greater than 2 m/s continuous wave Doppler has to be used.
PWD is particularly useful for assessing relatively low-velocity flows associated with transmitral or transtricuspid blood flow, left ventricular outflow tract flow, pulmonary venous flow, or left atrial appendage flow.
High pulse repetition frequency (HPRF) Doppler enables measurement of high velocities with PWD by employing special transducers with the ability to emit multiple pulses. Because any emitted pulse does continue beyond the primary sampling depth (albeit weaker), the net effect is increased sampling at locations beyond the primary depth. Depending on the number of pulses, the Nyquist limit can be increased by a factor of 2 or more. The increase in sampling frequency is, however, at the expense of range ambiguity. The primary application of HPRF Doppler is in resolving the velocity of multiple high-velocity lesions that are in series (Fig. 5-6).
In the continuous-wave Doppler (CWD) mode, the ultrasound transducer utilizes two crystals—one to continuously transmit ultrasound beams and another to constantly receive the reflected waves (Fig. 5-4). Because there is one crystal dedicated to receiving the reflection, the maximal frequency shift that can be detected is not limited by the pulse repetition frequency, and very high velocities can be recorded without aliasing. Blood flow velocities are thus measured accurately when velocities are greater than 1.5 m/s. However, the CWD signal is not time-gated and measures the highest velocity in the path of the ultrasound beam, thus suffering from “range ambiguity,” or the inability to discriminate the precise location of the highest recorded velocity, unlike PWD. As a result, Doppler shift data are analyzed along the entire course of the ultrasound beam. This leads to two disadvantages:
There may be overlap of velocity envelopes seen as differently shaded, multiple velocities measured in the course of the ultrasound beam (Fig. 5-7).
Because a large range of blood velocities is interrogated, there is “spectral broadening” of the velocity envelope and loss of spatial specificity, compromising its sharpness and homogeneity as compared to PWD (Fig. 5-8).
Color flow Doppler (CFD) is a technique by which the Doppler shifts within the heart are displayed in the form of colors superimposed on 2D images giving an impression of real time blood flow. CFD is based upon pulsed-wave Doppler methods. However, whereas PWD provides information on peak velocities, CFD reports on the direction and mean velocities of flow (velocity mode), as well as differentiation of laminar versus turbulent flow (variance mode). Blood flow directed toward the transducer is commonly (but not exclusively) color-coded in shades of red, whereas blood flowing away from the transducer is coded in shades of blue. Lighter and darker shades within each of these colors typically represent faster and slower velocities, respectively. The color-coding is ultimately dependent on the color map being used (displayed on the top-right corner of the image). Velocity maps display colors below the baseline representing flow away from the transducer and colors above the baseline representing flow toward the transducer. In addition to colors above and below the baseline, variance maps display colors side by side when moving from left to right on the color map. The colors on the right side of a variance map (usually yellow and green) represent turbulent flow (Fig. 5-9). It is therefore important to always refer to the color map and not simply rely on conventions. Color Doppler has the characteristics of pulsed-wave Doppler, range discrimination, and aliasing. Aliasing is observed as reversal of color into the opposite direction when the mean velocities measured exceed the Nyquist limit and can be present in the absence of turbulent flow (Fig. 5-9).
Tissue Doppler imaging (TDI) is an echocardiographic technique that uses Doppler principles to measure the velocity of myocardial motion. Conventional Doppler modalities are designed to calculate the intracardiac or intravascular blood flow velocities (i.e., high-velocity and low-amplitude signals). TDI is geared to detect velocities of the myocardial tissue (i.e., low-velocity, high-amplitude signals).3–5 Current echocardiography systems come equipped with presets for measuring TDI signals and are generally set to measure myocardial velocities up to 20 cm/s and detect amplitudes greater than 20 dB. In contrast, the amplitude of the movement of red blood cells is generally less than 15 dB.6 TDI can be performed in pulsed-wave and color modes. Pulsed-wave TDI is used to measure peak myocardial velocities at specific locations determined by the position of the sample volume. It has high temporal resolution but analyzes a single myocardial segment at one time. Pulsed-wave TDI signals can be displayed as spectral tracing. This TDI modality is particularly useful for the measurement of long-axis ventricular motion in the mid-esophageal views by transesophageal echocardiography because the longitudinally oriented fibers are most parallel to the ultrasound beam. In the color mode, TDI measures mean myocardial velocities represented as color codes superimposed on the 2D image. Color-TDI provides qualitative information regarding myocardial velocities; however, it can evaluate multiple segments simultaneously. The digitally stored color TDI loops can be processed offline, and the mean velocities can be extracted by using an M-mode cursor or a sample volume and displayed in a spectral format.
Pulsed-wave TDI allows the measurement of several parameters with clinical and prognostic significance. To measure longitudinal myocardial velocities, the sample volume is placed in the ventricular myocardium immediately adjacent to the mitral annulus.
The superscripted prime symbol (e′) is used to differentiate tissue Doppler velocities from conventional mitral inflow. The mitral annular tissue Doppler velocity display (Fig. 5-10) shows:
s′ = systolic myocardial velocity below the baseline as the annulus descends toward the apex;
e′ = early diastolic myocardial relaxation velocity above the baseline as the annulus ascends away from the apex during early filling; and
a′ = myocardial velocity above the baseline associated with atrial contraction.
Clinical applications of TDI include assessment of left ventricular diastolic function and cardiomyopathies, estimation of LV filling pressures, and differentiating between restrictive cardiomyopathy and constrictive pericarditis.
Two-dimensional and Doppler imaging can be used to obtain comprehensive hemodynamic data, but it should be remembered that these data, although providing a more objective measure of cardiac performance, should always be interpreted within the context of the patient’s clinical condition.
In circulation, the blood flow and velocity are phasic (i.e., change throughout the cardiac cycle). A Doppler spectrum of the velocity of blood flowing through a conduit will yield a curve during systole that has velocity (cm/s) on the y-axis and time (s) on the x-axis. When this curve is integrated, it yields a velocity-time integral (VTI) in units of centimeters (velocity [cm/s] time [s] = VTI [cm]). The VTI is a manifestation of the stroke distance (i.e., the distance the stroke volume travels over time during a single systolic ejection period). The product of VTI (cm) and cross-sectional area (CSA; cm2) will yield stroke volume (SV; cm3) (Fig. 5-11):
Cardiac output can then be calculated as:
Cardiac index can be calculated as:
The calculation of SV through a conduit assumes laminar flow, a flat-flow profile in which velocities are uniform, the diameter of the conduit is constant, velocity is measured at the same point as the diameter, velocity measured represents the mean velocity during the entire ejection period, and the measurement of the conduit diameter is accurate (a common source of error). Stroke volume can be measured at any conduit in the heart; however, the preferred site for such calculation is the left ventricular outflow tract (LVOT) (Fig. 5-12). Other intracardiac sites such as the pulmonic and mitral valves have a dynamic conduit diameter during the cardiac cycle, and at times the velocity profile is parabolic, where velocities vary across the parabola.
FIGURE 5–12.
Imaging windows used to calculate stroke volume. The mid-esophageal long-axis view is used to measure left ventricular outflow tract (LVOT) diameter, and the deep transgastric five-chamber view is used to measure the LVOT velocity-time integral (VTI). CSA, cross-sectional area; PWD, pulsed-wave Doppler.

In echocardiography, it is assumed that a conduit or orifice, such as the LVOT, is circular. The CSA is calculated from the diameter measurements by using the equation for the area of a circle:
The diameter of the LVOT is calculated from the mid-esophageal long-axis window, and LVOT VTI is calculated from the deep transgastric window (see Fig. 5-12).
The ratio of pulmonary (Qp) to systemic (Qs) flow is a useful parameter to assess the magnitude of shunting, with a ratio greater than 1.5 generally considered significant. Qp/Qs measurement requires calculation of the stroke volume at the right ventricular outflow tract (RVOT) and the left ventricular outflow tract (LVOT). Thus, for atrial or ventricular shunts:
For shunts occurring through a patent ductus arteriosus:
Assuming a constant flow of fluid through a conduit at a certain velocity, if there is a stenosis in the conduit, the velocity of fluid will increase at the site of stenosis to conserve flow. This concept is known as the continuity of flow and sometimes as the conservation of flow:
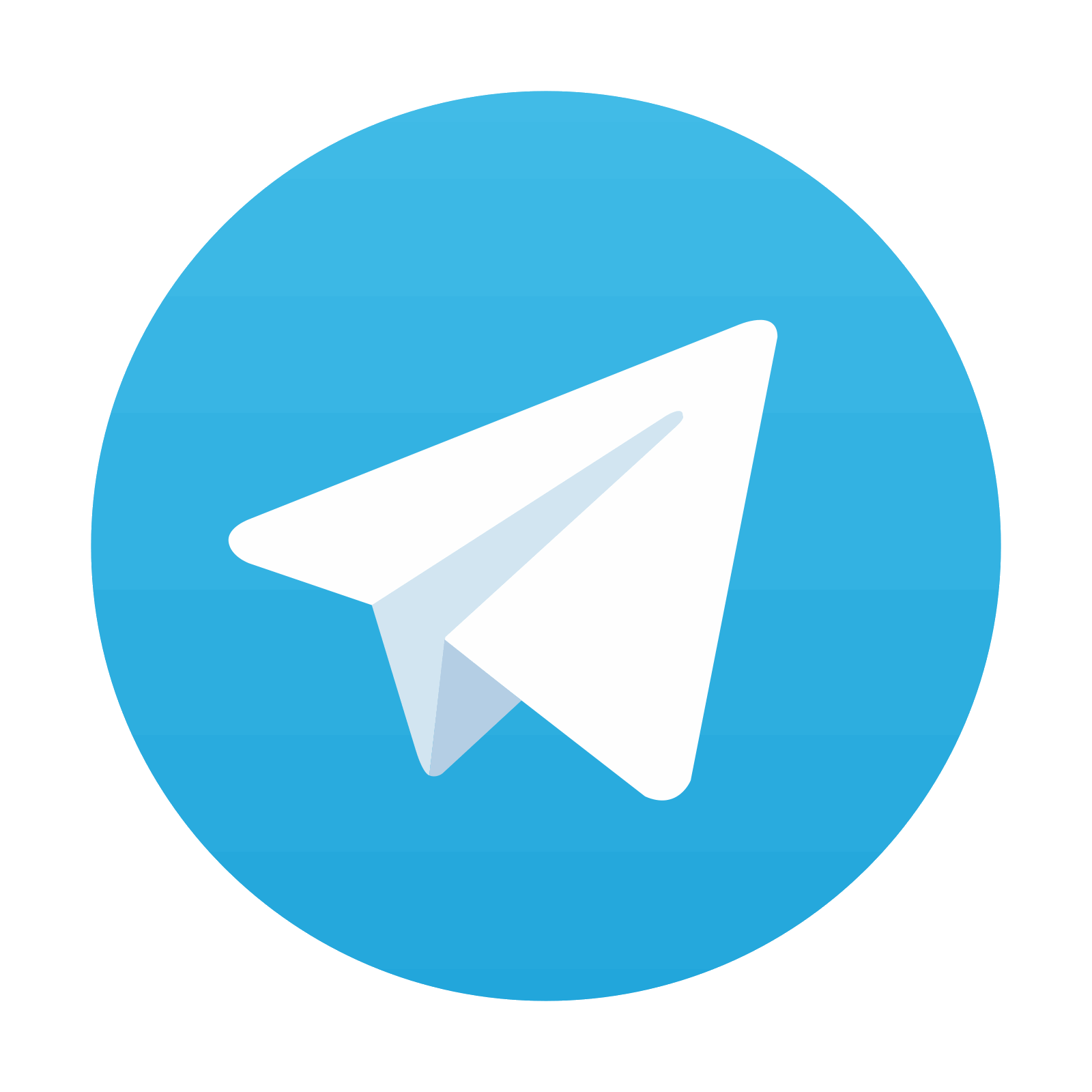
Stay updated, free articles. Join our Telegram channel
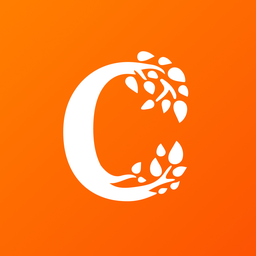
Full access? Get Clinical Tree
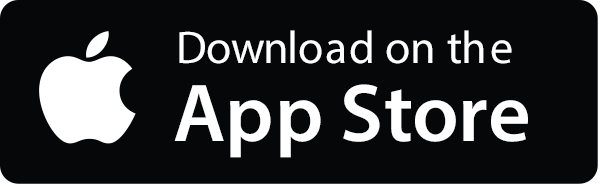
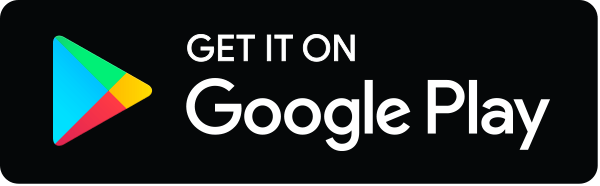
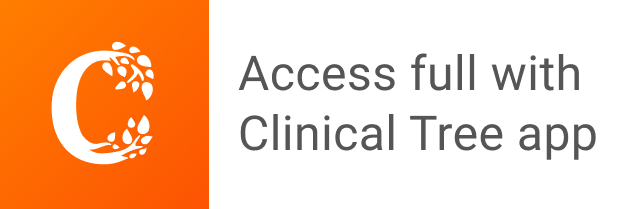