Christian Doppler, in his 1842 paper titled, “On the Coloured Light of the Binary Stars and Some Other Stars of the Heavens,” was the first to note that stars moving towards the earth emitted blue light while stars moving away from earth radiated red light. Thus, he postulated that the observed frequency of a wave depends on the relative speed of the source and the observer. Although Doppler himself never extended the principle to other natural phenomena, the common observation that the pitch of sound is different for a locomotive approaching the listener than one moving away led to a more widespread application of Doppler’s initial observation.
In clinical echocardiography, the Doppler technique depends upon an analysis of the frequency and wavelength of an emitted ultrasound beam. Frequency is defined as the number of waves passing though a certain point in 1 second, and is a fundamental property of the sound waves. It is expressed in units of hertz (Hz) and determines the resolution and the depth of penetration of the medium. However, the Doppler assessment of ultrasound waves depends upon not just the absolute emitted frequency, but the relative change in frequency as the sound waves are reflected back (by red blood cells) towards the transducer. The frequency of the reflected sound waves increases when the red blood cells are moving towards the transducer and decreases when red blood cells are moving away from the transducer (Figure 4–1). This relative change in the frequency, known as the Doppler shift, enables the echocardiographer to assess the direction, speed, and turbulence of blood flow, which in turn helps to objectively quantify intracardiac pressures and the severity of valvular stenosis and regurgitation.
The Doppler shift is defined as the change in frequency of the reflected ultrasound waves and is described by the following mathematical relationship1:
- Δf = 2ft × (v × Cosθ)/c
- Δf = Difference between the transmitted frequency (ft) and received frequency
- v = Velocity of red blood cells
- θ = Angle between the Doppler beam and the direction of blood flow
- c = Speed of ultrasound in blood (1540 m/s)
When the Doppler beam is parallel to the direction of blood flow, the cosine of θ is 1 and the Doppler shift is most accurately calculated, but with increases in θ there is a progressive decrease in the measured Doppler shift. This underestimation of Doppler shift remains clinically insignificant until θ exceeds 20° and the associated percentage error exceeds 7% (Figure 4–2).2 When the Doppler beam is perpendicular to the direction of flow, the Doppler shift is recorded as zero since the cosine of 90 is zero. It is therefore essential to align the Doppler beam as parallel to the direction of blood flow as possible for an accurate measurement of speed and direction of flow.
All ultrasound systems are equipped to create both a spectral display and an audio component of the beat-to-beat Doppler data during acquisition. The loudness and pitch of this audio signal varies with the strength and quality of the Doppler data, and experienced echocardiographers have been known to use the audio component alone to acquire and record the maximal velocity. The loudness/pitch of the audio signal can also be used to qualitatively diagnose the presence of stenosis; however, the information obtained from audio analysis is qualitative and subjective and should not be used as the sole means of quantification.1
On the other hand, quantitative Doppler data on a spectral display provides a high degree of spatial and temporal resolution. Before the Doppler information can be displayed as distinct envelopes (velocity profiles over time) on the spectral display, the raw Doppler data undergo significant post-acquisition manipulation (demodulation, fast Fourier transformation, and chirp-Z transformation) to isolate the required frequencies.1 If the returning frequency is higher than the transmitted frequency, it is referred to as a “positive Doppler shift” (blood moving towards the transducer) and is displayed above the baseline. If the returning frequency is less than the transmitted frequency, it is called a “negative Doppler shift” (blood moving away from transducer) and is displayed below the baseline.
It is important to remember that a simultaneous display of the two-dimensional (2D) image and Doppler information requires a time-share arrangement between two independent functions of the transducer. In the case of a continuous spectral display, the 2D image display is rapidly switched on and off, giving the impression of a continuous image alongside the spectral display. Thus, there is always some reduction in the quality of 2D and Doppler data when a simultaneous imaging mode is used.1
Doppler can be used either in “pulse” or “continuous” mode to interrogate intracardiac flow patterns. Both these modalities have strengths and weaknesses that are suited for specific situations.
PWD utilizes a single ultrasound crystal that alternates between transmission and reception. As a consequence, the maximal frequency shift (and thus maximum velocity) that can be measured is limited to one-half the pulse repetition frequency (also known as the Nyquist limit). When this maximal frequency shift is exceeded, aliasing or a wrapping around of the velocities is seen on the spectral Doppler display. In general, the maximum measurable velocity without aliasing with PWD is less than 2 m/s. The pulse repetition frequency (PRF) is directly related to the depth of placement of the sample volume, ie, the longer the time it takes for the sound to travel back to the transducer, the lower the PRF (Figure 4–3). An advantage of PWD is that the operator can select the specific site of measurement by manually positioning the sample volume during conventional 2D echocardiography, thus providing “range resolution” or the ability to identify the exact location of the recorded velocity (Figure 4–4). Simultaneous display of the location of the sample volume and the spectral Doppler data is also possible with some sacrifice of the quality of the 2D image as well as the Doppler signal. The distance from the transducer determines the size and three-dimensional shape of the sample volume. The farther the sample volume is placed from the transducer, the larger it becomes due to progressive divergence of the ultrasound beam from the transducer.
Figure 4-4.

PWD allows the operator to measure a specific site by manually positioning the sample volume. CWD measures the highest velocity in the path of the ultrasound beam, thus suffering from “range ambiguity” or the inability to discriminate the precise location of the highest recorded velocity. The velocity envelopes obtained with CWD are typically shaded, representing the multiple velocities measured in the course of the ultrasound beam.
During transmission of a PWD beam, the signals returning to the transducer are interpreted on the basis of the time it takes for them to return to the transducer, a process referred to as “time-gating.” During time-gating, signals returning after a specific time from a preselected depth are chosen for interpretation, and all other returning signals are selectively ignored by the ultrasound system. Time-gating is described by the following mathematical relationship:
Time Delay = 2d/C
- d = Depth
- C = Speed of sound in blood
A technique to measure high velocities with PWD employs special transducers with the ability to emit multiple pulses. Since any emitted pulse does continue beyond the primary sampling depth (albeit weaker), the net effect is increased sampling at locations beyond the primary depth. Depending on the number of pulses, the Nyquist limit can be increased by a factor of 2 or more. The increase in sampling frequency is, however, at the expense of range ambiguity. The primary application of HPRF Doppler is in resolving the velocity of multiple high-velocity lesions that are in series (Figure 4–5).
In the continuous-wave mode, the ultrasound transducer utilizes two crystals—one to continuously send an ultrasound beam and another to constantly receive the reflected wave (see Figure 4–4). Since there is one crystal dedicated to receiving the reflection, the maximal frequency shift that can be detected is not limited by the pulse repetition frequency, and very high velocities can be recorded without aliasing. However, the CWD signal is not time-gated and measures the highest velocity in the path of the ultrasound beam, thus suffering from “range ambiguity” or the inability to discriminate the precise location of the highest recorded velocity. The velocity envelopes obtained with CWD are typically shaded, representing the multiple velocities measured in the course of the ultrasound beam.
Color-flow Doppler is a pulsed ultrasound technique that color codes Doppler information and superimposes it on a 2D image, displaying information on the direction and the mean velocities of flow as color maps. Blood flow directed towards the transducer is commonly (but not exclusively) color-coded in shades of red, while blood flowing away from the transducer is color-coded in shades of blue. Lighter shades within each of these colors typically represent higher velocities. Color-flow Doppler uses packets of multiple pulses (3 to 20 per scan line), and therefore has a low temporal resolution. It has the characteristics of pulsed-wave Doppler (range discrimination and aliasing), but color-flow Doppler aliases at a lower velocity than traditional PWD because of the reduction in PRF associated with the simultaneous generation of a gray-scale and a color image.
Conventional Doppler modalities are designed to calculate the intracardiac or intravascular blood flow velocities (ie, high-velocity and low-amplitude signals). Doppler tissue imaging (DTI) is geared to detect velocities of the actual myocardial tissue (ie, low-velocity, high-amplitude signals).3–5 Current echocardiography systems come equipped with presets for measuring DTI signals, and are generally set to measure myocardial velocities in the range of 0.2 to 0.4 cm/s and detect amplitudes greater than 20 dB. In contrast, the amplitude of the movement of red blood cells is generally less than 15 dB.6 The DTI signals can be displayed as a spectral tracing, in association with color-flow Doppler signals, or as color-encoded 2D signals.
Two-dimensional and Doppler imaging can be used to obtain comprehensive hemodynamic data, but it should be remembered that these data, while providing a more objective measure of cardiac performance, should always be interpreted within the context of the patient’s clinical condition.
In circulation, the blood flow and velocity are phasic, ie, change throughout the cardiac cycle. A Doppler spectrum of the velocity of blood flowing through a conduit will yield a curve during systole that has velocity (cm/s) on the y-axis and time (s) on the x-axis. When this curve is integrated, it yields a velocity-time integral (VTI) in units of centimeters (velocity [cm/s] time [s] = VTI [cm]). The VTI is a manifestation of the stroke distance (ie, the distance the stroke volume travels over time during a single systolic ejection period). The product of VTI (cm) and cross-sectional area (CSA; cm2) will yield stroke volume (SV; cm3):
Stroke Volume (SV) = Stroke Distance (VTI) × Cross-Sectional Area (CSA)
Cardiac output can then be calculated as:
Cardiac Output = Stroke Volume × Heart Rate
Cardiac index can be calculated as:
Cardiac Index = Cardiac Output/Body Surface Area
The calculation of SV through a conduit assumes laminar flow, a flat flow profile in which velocities are uniform, constant diameter of the conduit, velocity measured at the same point as the diameter, velocity measured represents the mean velocity during the entire ejection period, and accurate measurement of the conduit diameter (a common source of error). Stroke volume can be measured at any conduit in the heart; however, the preferred site for such calculation is the left ventricular outflow tract (LVOT) (Figure 4–6). Other intracardiac sites such as the pulmonic and mitral valves have a dynamic conduit diameter during the cardiac cycle, and at times the velocity profile is parabolic, where velocities vary across the parabola.
In echocardiography, it is assumed that a conduit or orifice, such as the LVOT, is circular. The CSA is calculated from the diameter measurements by using the equation for area of a circle:
The diameter of the LVOT is calculated from the midesophageal long-axis window, and LVOT VTI is calculated from the deep transgastric window (Figure 4–7).
Figure 4-7.

Imaging windows used to calculate stroke volume. The midesophageal long-axis view is used to measure left ventricular outflow tract (LVOT) diameter, and the deep transgastric long-axis view is used to measure the LVOT velocity-time integral (VTI). (CSA, cross-sectional area; PWD, pulsed-wave Doppler.)
The ratio of pulmonary (Qp) to systemic (Qs) flow is a useful parameter to assess the magnitude of shunting, with a ratio greater than 1.5 generally considered significant. Qp/Qs calculation requires calculation of the stroke volume at the right ventricular outflow tract (RVOT) and the left ventricular outflow tract (LVOT). Thus, for atrial or ventricular shunts:
Qp/Qs = (CSARVOT × TVIRVOT)/(CSALVOT × TVILVOT)
For shunts occurring through a patent ductus arteriosus:
Qp/Qs = (CSALVOT × TVILVOT)/(CSARVOT × TVIRVOT)
Assuming a constant flow of fluid through a conduit at a certain velocity, if there is a stenosis in the conduit, the velocity of fluid will increase at the site of stenosis to conserve flow. This concept is known as the continuity of flow and sometimes as the conservation of flow:
FlowLarger Conduit = FlowStenosis
As described above, constant flow (cm3/s) in a conduit is the product of cross-sectional area (CSA) of the conduit (cm2) and the average velocity of the fluid (cm/s). Thus,
CSALarger Conduit × VelocityLarger Conduit = CSAStenosis × VelocityStenosis
When three variables are known, the fourth is easily determined with this equation, commonly known as the continuity equation.
In aortic stenosis, flow across the aortic valve is equal to the flow across the LVOT (Figure 4–8) and in order to determine the stenotic area, the continuity equation can be reordered as:
Thus:
Mitral valve area can also be similarly calculated, but it must be remembered that the transmitral flow must be the same as left ventricular SV, a condition that is met only in the absence of ventricular shunts and mitral and aortic regurgitation.
The principles of flow conservation can also be applied to assess regurgitant volumes and fractions. For this calculation, one valve is considered the “reference valve,” but it should be remembered that the assumption that the mitral valve orifice is circular may not always be valid. In the absence of aortic stenosis or regurgitation (see Chapters 7 and 9), SVLVOT can be substituted for SVAortic Valve
When molecules move within a large cavity toward a small orifice, as in stenotic and regurgitant lesions, the velocity increases over a large area and the velocity profile is hemispherical, with the cavity of the hemisphere facing the orifice. The velocity over the surface of the hemisphere is the same (isovelocity), and because it is proximal to the orifice, the surface area is known as proximal isovelocity surface area (PISA). The product of the (iso)velocity (cm/s) and the surface area of the hemispherical velocity profile (cm2) yields the flow (cm3).
If the flow approaching the orifice is examined with color-flow Doppler, with the color scale set so that the accelerated velocity exceeds the Nyquist limit, aliasing will take place and a semicircular shell of a contrasting color will appear to cap the orifice (Figure 4–9). To obtain this shell, the baseline for the color scale should be shifted in the direction of the jet of interest (eg, towards the transesophageal echocardiography [TEE] transducer for mitral regurgitation). The semicircular shell is in fact a hemisphere in three dimensions, and its surface area can be calculated as that of a sphere:
Figure 4-9.

Color-flow Doppler examination of transmitral flow in mitral stenosis. A: Velocity acceleration as flow approaches the stenotic orifice is demonstrated by aliasing of color flow, which shows a shell of proximal isovelocity surface area. The edge of the proximal isovelocity surface area is defined by the transition from blue to red color. In this case, the aliasing velocity is 26 cm/s and the radius of the hemisphere is 1.3 cm. B: The angle (α) subtended by the mitral leaflets is shown.
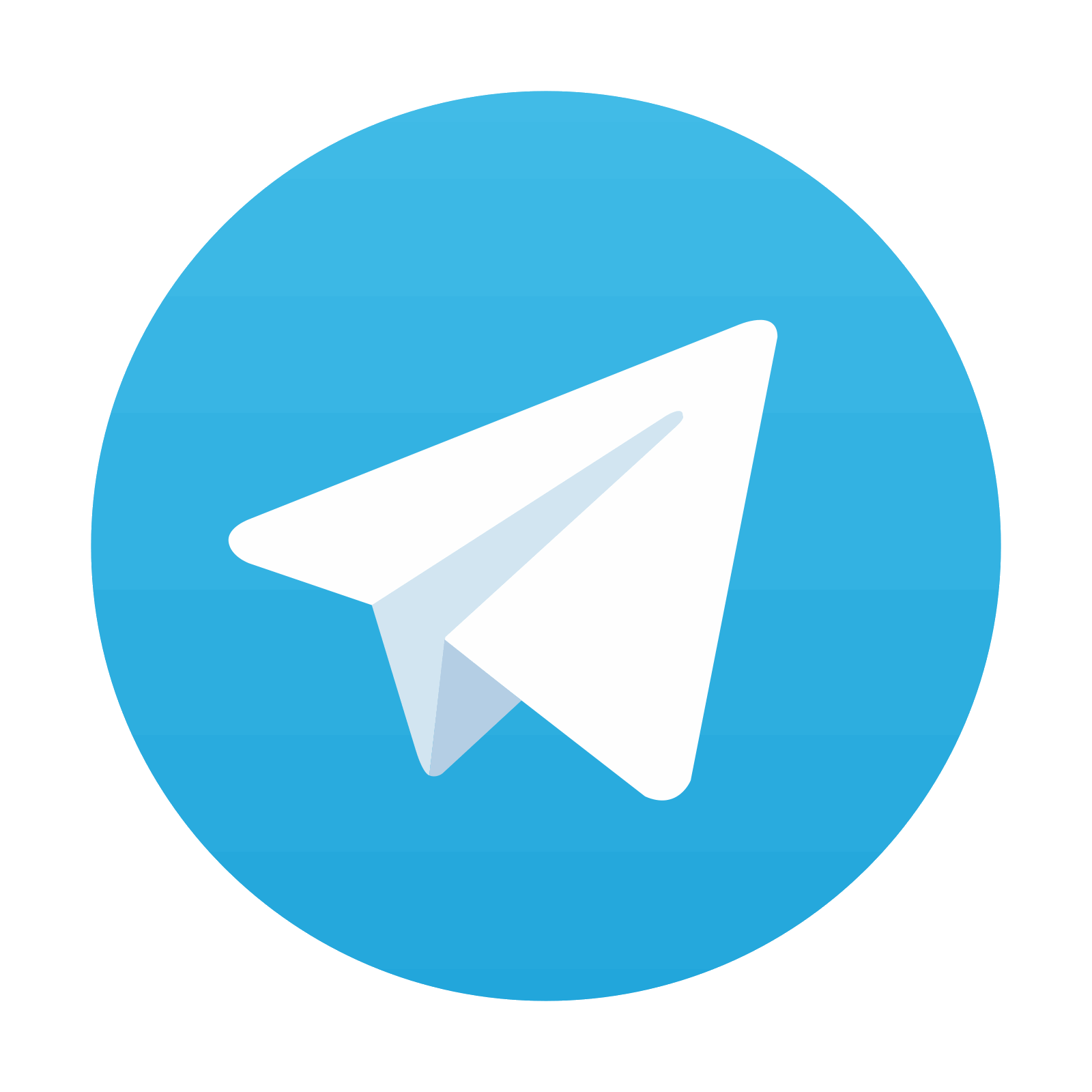
Stay updated, free articles. Join our Telegram channel
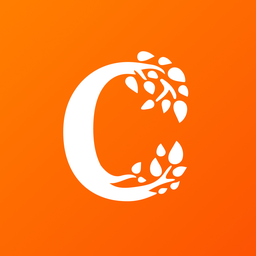
Full access? Get Clinical Tree
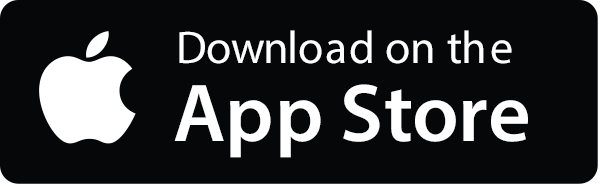
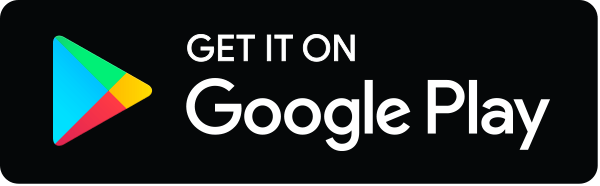
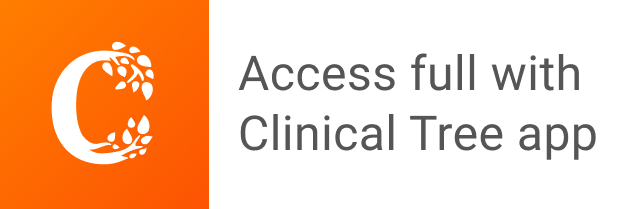