FIGURE 114.1 Outcomes in pulmonary embolism. RV, right ventricle.
Risk Factors
The Virchow triad of stasis, vessel trauma, and hypercoagulability remain fundamental risk factors for VTE. Furthermore, risk factors can be considered clinical (i.e., multiple trauma or major abdominal surgery, acute myocardial infarction or stroke, need for mechanical ventilation) or patient-related (prior history of VTE, malignancy, inherited coagulopathy).
With respect to lower extremity deep venous thrombosis (DVT), observational studies of medical–surgical ICU patients have identified mechanical ventilation, treatment with neuromuscular blockers, and presence of a central venous catheter (CVC) as risk factors for DVT (4). Central venous catheterization, in particular, has been reported to confer an increased relative risk (RR) of 1.04 for each day the catheter was in place (5). Among 261 medical–surgical ICU patients, multivariate regression analysis defined exposure to platelet transfusions and the use of vasopressors to be independent risk factors for ICU-acquired DVT (6).
TABLE 114.1 Venous Thromboembolism Prophylaxis of Critical Care Patient | ||
![]() |
The two most powerful patient-related risk factors are a prior history of VTE and malignancy. Malignancy is perhaps the most common acquired hypercoagulable state encountered in the ICU and likely will become more prevalent as the general population continues to age. Additionally, end-stage renal disease has been identified as an independent risk factor for ICU-acquired DVT (6). Activated protein C resistance from factor V Leiden (FVL) is the most common hereditary defect predisposition for DVT. In order of descending prevalence among the general population, prothrombin gene mutation 20210A, antithrombin, protein C and S deficiency, elevations in homocysteine, and coagulation factors VIII, IX, and XI may predispose the patient to developing DVT (4). Although uncommon, heparin-induced thrombocytopenia (HIT), an acquired platelet disorder, results in increased risk of both venous and arterial thrombosis (7).
Cancer and the presence of a CVC are the two most powerful risk factors for upper extremity DVT (UEDVT). In the report of Mustafa et al., a CVC at the site of the UEDVT was present in 60% of patients and 46% were diagnosed with cancer. Underscoring the possible additive nature of these two risk factors, 76% of the patients with cancer also had an indwelling CVC (8). In a review of cancer patients who had an indwelling CVC, the reported prevalence of UEDVT, either symptomatic or asymptomatic, ranged from 6.7% to 48% (9). In a prospective registry of 592 patients with UEDVT, the presence of an indwelling CVC was the strongest independent predictor of occurrence (odds ratio [OR], 7.3) (10). It is important to note that nearly 30% of patients will develop an UEDVT with no apparent cause. In these patients, inherited thrombophilia, particularly FVL, prothrombin G20210A mutation, and anticoagulant protein deficiencies may be causative. Evaluated prospectively for a median follow-up of 5.1 years, recurrence of primary UEDVT was reported to be 4.4% in those with inherited thrombophilia compared to 1.6% in those without (11).
Prevalence/Incidence
Critically ill patients are at substantial risk of developing DVT and accompanying PE as frequently all three components of Virchow triad of stasis, hypercoagulability, and intimal vessel damage are impacted by disease states and treatments. In the absence of prophylaxis, it is reported that the incidence of VTE ranges from 13% to 30% (12). As a consequence, virtually all patients admitted to an ICU are considered high risk and some form of prophylaxis is indicated. Omission of DVT prophylaxis within 24 hours of admission is reported to be associated with increased mortality in critically ill patients (13) and although unfractionated heparin (UFH) has proven to be effective in diminishing the rate of DVT, significant failure rates of up to 15% have been reported (6,14,15).
Patients undergoing major general surgery have an event rate of 25% without DVT prophylaxis; 9% of patients will have clinically detectable DVT, and 7% will have proximal DVT. In a study of trauma patients who did not receive prophylaxis, 58% of patients developed a DVT, one-third of which were proximal. Similarly, in patients undergoing elective hip surgery and not receiving prophylaxis, the incidence of DVT is 50%, with 23% being clinically detectable and 20% proximal (16). The pooled incidence of detectable DVT in neurosurgical patients is 22%, and the incidence in acute spinal cord injury patients is as high as 90% when prophylaxis is not used (4). The incidence of DVT in critically ill medical patients is reported to be 1% to 15% depending on which screening technique was used. No studies specific to the critically ill have been performed regarding UEDVT, and no prospective studies using systematic screening techniques are available to assess the prevalence or incidence. Nonetheless, there has been an increase over the last several decades attributed to the greater use of transvenous pacemakers and CVCs. Symptomatic PE is reported to occur in 7% to 9% of these patients (17,18), and studies using systematic ventilation/perfusion scanning in those previously diagnosed with UEDVT have reported high-probability scans in 13% (19).
Pharmacologic Prophylaxis
Four randomized controlled trials have evaluated the efficacy of UFH and/or low–molecular-weight heparin (LMWH) compared to placebo in critically ill patients (20–23). A systematic review that included these trials reported that UFH- or LMWH-prophylaxed patients had a significantly decreased relative risk for DVT (RR) 0.51 and PE (RR 0.52), with similar risk of bleeding compared to placebo (24). Three RCTs compared LMWH to UFH (25–27) and did not report significant differences in the incidence of DVT. Meta-analysis of similar data reported that LMWH decreased the incidence of PE compared to UFH (24).
Questions have been raised, whether subcutaneous (SQ) administration of LMWH has sufficient bioavailability to achieve therapeutic plasma levels (≥0.3 IU/mL) in the critically ill. A prospective, controlled, open-labeled study of enoxaparin, 40 mg once daily, in critically ill patients with normal renal function demonstrated significantly lower anti-Xa levels when compared with medical patients in the normal ward (28). This difference does not appear to be associated with vasopressor administration. These findings call into question whether once-daily dosing is appropriate for the critically ill.
Fondaparinux, a synthetic factor Xa inhibitor, in doses of 2.5 mg SQ daily has been reported to decrease VTE rates by half as compared to placebo in older, acutely ill medical patients (ARTEMIS) (29); to be more effective than enoxaparin, 30 mg twice daily, for VTE prophylaxis after elective major knee surgery (30); and equivalent to dalteparin, 5,000 IU daily, for the prevention of VTE in high-risk abdominal surgery (PEGASUS) (31). Of note, patients requiring mechanical ventilation and those with severe sepsis and septic shock were excluded from these trials, and most were not in the ICU. Nevertheless, the notion that these drugs are superior to placebo in the prevention of VTE is indeed supported. Although meta-analysis of DVT prophylaxis in critically ill patients did not report evidence of increased bleeding with pharmacologic prophylaxis compared to placebo, the risk benefit ratio should be reviewed for all patients; when bleeding risks are high, mechanical prophylaxis should be instituted.
Mechanical Prophylaxis
Graded compression stockings, intermittent pneumatic compression (IPC) devices, and venous foot pumps (VFPs) are attractive insofar as they are without bleeding risk. Compression stockings are reported to be less effective than pharmacoprophylaxis, and their utility as an independent prophylactic agent is uncertain (32). Two RCTs of mechanical prophylaxis reported IPCs combined with pharmacoprophylaxis; one reported a synergistic effect in decreasing DVT (33) and one reported no differences between IPC and Compression stockings (34).
In summary, all critically ill patients should receive DVT prophylaxis. Pharmacoprophylaxis is recommended in the absence of a bleeding contraindication and, when precluded, mechanical prophylaxis should be instituted (35). In an unblended study of 422 trauma patients, more DVT occurred in patients in whom IPC devices were used than with LMWH (2.7% vs. 0.5%) (36). Only a trend toward significance was found among 2,551 consecutive patients undergoing cardiac surgery treated with either UFH, 5,000 U SQ twice daily, or a combined prophylactic regimen of IPC and UFH; the incidence of objectively confirmed PE decreased from 4% to 1.5% (37). Use of IPC did not appear to have any additional benefit when used with either UFH or LMWH in a randomized pilot trial for VTE prophylaxis in patients undergoing craniotomy (38). IPC was less effective in preventing PE when used in addition to UFH versus LMWH alone in a prospective, randomized, multicenter trial involving acute spinal cord injury patients (39).
IVC Filters
The idea of interrupting the inferior vena cava (IVC) to prevent transit of lower extremity thromboses to the pulmonary circulation is attributed to Trousseau in 1868 (40). Today, IVC interruption is most often carried out by percutaneous insertion of a filter or “umbrella” via the femoral or jugular vein. As a result of technical refinements and ever-increasing expertise in performing the procedure, this one-time surgical technique is performed nearly 50,000 times a year (41). Categorical indications for IVC filter (IVCF) placement include contraindications to anticoagulation (absolute or relative), complications of previously instituted anticoagulation (failure, bleeding, thrombocytopenia, drug reactions), as a prophylactic adjunct to anticoagulation in patients thought to be unable to withstand another embolic event, failure of a previous IVCF, or in association with another procedure (thrombectomy, embolectomy, or thrombolysis). Unfortunately, methodologically sound literature in support of specific indications for filter placement is lacking.
Decousus et al. (42) reported one of the first RCTs of IVCFs for the prevention of PE in patients with documented proximal LEDVT. Patients were followed for 2 years in the initial report, and results of a longer-term follow-up in the same patients were reported after 8 years (43). In the initial report, 400 patients were randomized to receive a filter or no filter in addition to anticoagulation with UFH or LMWH. At 12 days, fewer patients suffered symptomatic or asymptomatic PE in the filter group while bleeding and mortality were unaffected. At 2 years, the number of patients suffering symptomatic PE was no longer significantly different (as a result of more symptomatic PE between years 1 and 2 in the filter group), and the recurrence of DVT was significantly higher in the filter group; placement of an IVCF had no effect on survival. At 8-year follow-up, patients with filters still had higher DVT rates, but symptomatic PE was lower than in patients without a filter; mortality was still unaffected. These reports suggest that DVT patients with or without PE may derive limited benefit from an IVCF in addition to anticoagulation alone. Placement of a filter in PE patients who have failed anticoagulation are at higher risk for a decrease in IVC patency or frank occlusion over time when compared to those with other indications for filter placement (44). However, no differences in edema formation, occurrence of varicose veins, trophic disorders, ulcer formation, or postthrombotic syndrome have been shown between those with and without a filter (43). Percutaneous filter placement in the superior vena cava may also be considered for prevention of symptomatic PE due to acute UEDVT in patients in whom therapeutic anticoagulation has failed or is contraindicated. Limited observational data support its safety and efficacy in this setting (45,46).
IVCF use has not been systematically studied in critical care outside the setting of major trauma, where the deployment of retrievable IVCFs (R-IVCF) has been favored. Allen et al. (47) reported that retrievable filters are safe and effective in the prevention of PE in high-risk trauma patients with contraindications to anticoagulation. Interpretation of their findings is hampered by several factors: lack of anticoagulated patients as a comparator, small numbers (53 devices placed in 2,426 patients), and a low overall incidence of thromboembolic events (2.1% with DVT, 0.2% with nonfatal PE, no fatal PE). Others have cautioned that liberal application of these filters in the trauma population does not alter rates of VTE and may lead to a greater incidence of filter and retrieval-related complications (48). Furthermore, two studies reported that only about one in five of these devices is, in fact, retrieved, suggesting that they have simply become permanent filters (48,49). Although there is limited data specifically referable to IVCs in critical care, a large retrospective analysis of IVC filters in patients showed that placement of IVCs in unstable PE patients decreased in-hospital all-cause mortality (24.5% vs. 42.0%). In unstable PE patients receiving thrombolytic therapy and an IVC, the mortality was 7.6% compared to 51.5% in those that received neither (50,51). The PREPIC 2 study evaluated the efficacy and safety of R-IVCFs versus anticoagulation in patients with the severity criterion (age >75 years, active cancer, chronic cardiac or respiratory disease, ischemic stroke with paralysis, DVT with iliocaval involvement) or evidence of right ventricular dysfunction and/or myocardial injury. At 3 and 6 months, there was no difference between the groups in recurrent PE, symptomatic DVT, major bleeding, or death (52). The preceding studies suggest that there is benefit in unstable PE patients but little support for filter placement in stable PE patients that can tolerate full anticoagulation.
DVT
Diagnosis
Validated prediction rules have been published and are useful in patients able to communicate their symptoms. However, many ICU patients will be incapable of effectively communicating any symptoms due to altered mental status, requirement for mechanical ventilation, and/or infusions of sedatives, analgesics, or neuromuscular blocking drugs; physical examination is equally unhelpful.
The gold standard for DVT is lower limb venography (53). When adequately performed, it is able to detect all clinically important forms of DVT, including calf thrombosis, thrombosis of the pelvis, and the IVC. Because of the technical nature of the test, risk of radiocontrast-induced nephrotoxicity, and need to transport the patient from the ICU, it is rarely performed outside research settings. Consequently, compression ultrasound (CUS) is the most commonly reported method of detecting DVT in the ICU setting (54). For symptomatic patients, the reported pooled sensitivity for CUS in excluding a proximal DVT is 97%, but only 62% in asymptomatic patients. Furthermore, CUS lacks sensitivity in the detection of distal DVT, yielding pooled sensitivities of 73% and 53% for symptomatic and asymptomatic patients, respectively (55). Negative serial CUS over a 7- to 10-day period may effectively rule out clinically important DVT, but thus far has only been validated in symptomatic outpatients (53). An alternative to both venography and CUS is computed tomography venography (CTV) of the lower extremities and the pelvic veins as a continuation of CT angiography of the pulmonary arteries. In the setting of diagnostic workup for PE, CTV has a reported sensitivity and specificity of 70% and 96%, respectively, for all DVT, comparable to CUS in one study (56) and was superior to CUS for detection of iliofemoral DVT in a second report, yielding 100% sensitivity and specificity (57). The PIOPED investigators reported that CTV and CUS are diagnostically equivalent, reporting a 95.5% concordance between CTV and CUS for diagnosis or exclusion of LEDVT (58). Thus the choice of imaging technique can be made on the basis of safety, expense, and time constraints. Limitations of the test are the same as for those previously mentioned for CT angiography of the pulmonary arteries.
In the case of UEDVT, the first-line diagnostic test is color duplex ultrasound with a three-step protocol involving compression, color, and color Doppler with reported sensitivity and specificity ranging from 78% to 100% and 82% to 100%, respectively (9). In the event of vessel incompressibility but in the presence of isolated flow abnormalities in combination with persistent clinical likelihood, contrast venography should be considered. Magnetic resonance venography (MRV) has been studied but with disappointing results. Reported sensitivities are 50% and 71% for MRV with and without gadolinium enhancement, respectively (57).
Treatment
The mainstay of therapy for all forms of VTE is anticoagulation. The reader is referred to the section for treatment of PE for further details. For larger clot burden involving the iliofemoral system, some suggest administration to thrombolytics. Indeed, a Cochrane review concluded that thrombolysis reduces postthrombotic syndrome and maintains venous patency after DVT when compared to traditional anticoagulation (59). However, the optimum drug, dose, and route of administration have yet to be determined. Endovascular catheter-directed thrombolysis is another promising treatment for acute iliofemoral thrombosis.
PULMONARY EMBOLISM
Contemporary risk stratification for the diagnosis, resuscitation, and treatment of PE is predicated on an implicit understanding of the pathophysiology of PE. The vicious pathophysiologic sequence of events related to the impaction of the embolic material on the pulmonary outflow is depicted in Figure 114.2. The combination of mechanical obstruction and neurohumoral factors, combined with the patients underlying cardiopulmonary status, results in an increase in pulmonary vascular impedance and the induction of pressure load on the right ventricle. Although the impact of the mechanical obstruction is well appreciated, the effect of neurohumoral influence is significantly underappreciated. The release of factors from platelets in the imbedded clot, which include serotonin, adenosine diphosphate (ADP), and thrombin, all precipitate vasoconstriction in the pulmonary artery system (60). The development of a pressure load will precipitate right ventricular decompensation, which decreases right ventricular output. Because the heart is two hydraulic pumps linked in series, diminished output of the right ventricle will result in diminished left ventricular preload. The consequence of diminished left ventricular preload is a decrease in cardiac output (CO) and a resultant loss of mean arterial pressure. The perfusion pressure gradient for the right ventricular subendocardium is the difference between the mean arterial pressure and the right ventricular end-diastolic pressure. PE precipitates an increase in right ventricular end-diastolic pressure through the induction of a right ventricular pressure load and the development of right ventricular decompensation. This increases right ventricular myocardial oxygen demands, which, because of the diminished gradient between the mean arterial pressure and the right ventricular subendocardium, induces further right ventricular decompensation and resultant right ventricular ischemia. The right ventricle compensates through the use of the Starling mechanism and increases right ventricular volume. This results in leftward shift of the intraventricular septum, further jeopardizing of left ventricular filling. Pericardial restraint further limits of left ventricular filling, and impairments of left ventricular distensibility additionally decreasing left ventricular preload. This pathophysiologic sequence results in a vicious cycle of ventricular decompensation that manifests as hemodynamic instability and shock.

FIGURE 114.2 Pathophysiology of pulmonary embolism. CO/MAP, cardiac output/mean arterial pressure; CPP, cardiopulmonary pressure; LV, left ventricular; RV, right ventricular.
Recent autopsy and laboratory investigations suggest that the pathophysiology of PE is strongly linked to an inflammatory state induced by the preceding pressure-volume overload phenomena. An autopsy study of patients with fatal PE and no underlying cardiopulmonary disease reported inflammatory infiltrates composed of predominantly macrophages, T cells, and frequent neutrophilic inflammation with myocyte necrosis identified in association with the necrosis in 64% of cases; this was associated with a 6.1-fold increase in right ventricular fibrosis compared to controls (61). These inflammatory changes differed from the traditionally proposed ischemic mechanisms of RV dysfunction as they were multifocal rather than zonal, suggesting direct cellular injury resulting in fibrosis that may be a significant contributor to the persistent RV dysfunction observed in some patients. Animal laboratory investigations report that RV injury is related to both mechanical stress forces and RV ischemia that combine to promote a cytokine and chemokine-mediated inflammatory injury (62). Metalloproteinases (MMPs), important for remodeling of the cardiac extracellular matrix in both the developing and damaged heart, are reported to be activated by the pressure overload, and have been implicated as a cause of contractile dysfunction and troponin leaks (63). In animal models, inhibition of MMPs activity by doxycycline, tetracycline, and atorvastatin has been shown to attenuate MMP effects resulting in improvements in hemodynamics and CO, and decreases in pulmonary vascular resistance (PVR), RV dilatation troponin levels, and animal mortality (63,64). It is important to recognize that PE is a spectrum of presentations and that the most extreme form of PE will result in gross hemodynamic instability and cardiac arrest. Figure 114.3 illustrates a diagrammatic overview of the sequence of events that occur in PE.

FIGURE 114.3 Pathophysiology and differential diagnosis of pulmonary embolism. CO, cardiac output; CPP, cardiopulmonary pressure; LV, left ventricular; MAP, mean arterial pressure; PMS, PAP, pulmonary artery pressure; RAP, right atrial pressure; RV, right ventricular; RVEDP, RV end-diastolic pressure.
The care of the critically ill patient often proceeds along two parallel pathways: physiologic resuscitation and generation of a differential diagnosis that eventually leads to a definitive diagnosis and treatment. Consequently, the use of a universally applicable model of the circulatory system is of substantial utility in characterizing the physiologic elements for resuscitation and assisting in the differential diagnosis generation. Figure 114.4 represents a three-compartmental model of the circulatory system that is characterized by two hydraulic pumps linked in series; each hydraulic pump has its own capacitance (volume reservoir) and impedance (resistive element) system. Insofar as the pumps are aligned in series, the output of one pump can never exceed the output of the other; consequently, hydraulic pumps may be conceptualized as a single hydraulic unit. Using this model, the circulatory system can be viewed as a venous capacitance reservoir that provides volume to a hydraulic pump that generates flow into an impedance bed. Any hemodynamic abnormality, such as hypovolemia, ventricular failure, sepsis, or major PE, may be characterized by defining one or more of the variables in this hydraulic pump. The surrogates for venous capacitance pressure, hydraulic pump function, and impedance are right atrial pressure (RAP), CO, and systemic venous resistance (SVR), respectively. Oftentimes invasive monitoring or echocardiographic assessment is not immediately available on patient presentation in the ICU. Consequently, it is frequently necessary to assess the model elements from physical examination; the venous capacitance reservoir may be estimated from examination the right internal jugular vein, and the pulse character and temperature of extremities maybe relied on to approximate the arterial impedance. Characteristically, a reciprocal relationship between flow and impedance is present in most disease states. Warm flushed extremities with a very wide pulse pressure indicate low vascular impedance and a correspondingly high flow state, whereas cool constricted extremities with a thready narrow pulse pressure suggest a state of high vascular impedance resulting from the compensatory increase in catecholamines to maintain perfusion pressure gradients. Recognizing that flow and impedance are almost uniformly reciprocal, one can exploit this relationship to assist with the differential diagnosis of shock patients. Therefore, the initial assessment of impedance (resistance) allows for the inferential derivation of flow. Cool, clamped hypoperfused extremities reflect a catecholamine surge and low flow state. Given the hydraulic pump alignment in series, the presence of an elevated jugular venous pressure against the background of clinical and radiographically clear lungs isolates the hemodynamic lesion to the right ventricle. The differential diagnosis of increased impedance, increased capacitance pressure, and decreased flow against the background of clear lungs is illustrated in Figure 114.3 and includes PE, right ventricular infarct, and pericardial tamponade. Impaired gas exchange in conjunction with the preceding is strongly suggestive of PE. Given the potential likelihood of anticoagulation and thrombolytic therapy in this patient population, invasive monitoring should be selectively used when the circulatory model variables cannot be well characterized from physical examination. A characterization of model variables for various shock states are depicted in Figure 114.4.

FIGURE 114.4 Three-compartment circulatory shock model.
The gas exchange abnormalities in PE are exceedingly complex and a function of the size and character of the embolic material, the magnitude of the occlusion against the background of the patients underlying cardiopulmonary status, and the interval time since the embolic event (65). The multiple causes of hypoxia have been attributed to an increase in alveolar dead space, ventilation–perfusion (V/Q) abnormalities, right-to-left shunting and, in the case of cardiogenic shock, a low mixed venous O2. Although seemingly counterintuitive, the multiple inert gas technique suggests that a low V/Q relationship develops and precipitates hypoxia in PE consequent to the redistribution of blood flow away from the embolized area, resulting in excessive perfusion in the unembolized lung regions and subsequent reperfusion through the atelectatic area of the previous clot.
It is especially instructive to examine the clinical manifestations of PE patients without underlying cardiac pulmonary disease because it permits the assessment of the effects of the embolic event and specific compensatory responses. In this particular population, the clinical and physiologic implications of PE are directly correlated to the size of embolism (66,67). In these studies, there is significant correlation observed between the magnitude of the angiographic obstruction and the mean pulmonary artery pressure (mPAP), RAP, PaO2, and pulse. It has been suggested that a PVR of greater than 500 dyne-s-cm−5 is correlative with a degree of obstruction exceeding 50% (68). It is interesting to note that depression in oxygen saturation is common and may occur with as little as a 13% angiographic obstruction and, commonly, is the only clinical manifestation when the obstruction is less than 25% (69). When the extent of pulmonary vascular obstruction is 25% to 30%, pulmonary hypertension begins to develop (normal mPAP, 20 mmHg). It is important to recognize that this represents an increase in excess of similarly described nonembolic experimental obstruction, which further illustrates the relative contribution of the neurohumoral mechanism to pulmonary vascular impedance. Patients without underlying cardiopulmonary disease are unable to generate an mPAP in excess of 40 mmHg, which is reported to be the maximal pressure that a healthy ventricle can generate. In patients without underlying cardiopulmonary disease, either a large single embolus or the cumulative incremental effects of multiple recurrent emboli generating obstructions over 50% are needed to precipitate right ventricular failure. Consequently, mPAP in excess of 40 mmHg represents either significant underlying cardiopulmonary disease or the effects of multiple embolic events that have occurred over a prolonged time period, enabling the development of the right ventricular hypertrophy. It is important to recognize that the relationship between PVR and the extent of anatomic obstruction is hyperbolic and not linear. Direct increases in PVR occur when anatomic obstruction exceeds 60% (70). In the population with no underlying cardiopulmonary disease, an increase in RAP in the setting of PE is almost uniformly indicative of severe pulmonary vascular obstruction. The RAP is characteristically related to the mPAP, but is generally not elevated until the latter exceeds 30 mmHg and anatomic obstruction exceeds 35% to 40%. RAP can be elevated without a decrease in CO in patients with PE. However, a decrease in CO without an increase in RAP should suggest an alternative non–PE-related diagnosis.
In contrast to patients without an underlying cardiopulmonary disease, patients with previous cardiopulmonary disease characteristically will manifest a significantly greater degree of cardiovascular impairment with less anatomic vascular obstruction (71). This is perhaps best exemplified in the European Pulmonary Embolism Trial where 90% of the patients who presented in shock had prior cardiopulmonary disease and 56% of those with prior cardiopulmonary disease presented in shock compared to only 2% of patients without cardiopulmonary disease (72). In this population of patients with prior cardiac disease, the level of mPAP is disproportionately elevated compared to that of anatomic obstruction, which strongly suggests that underlying cardiopulmonary hemodynamics dominates the presentation process. With a mean angiographic obstruction of only 23%, significant elevations in mPAP were reported in a population with previous cardiopulmonary disease, and the increment in the mPAP was directly related to the pulmonary capillary wedge pressure (PCWP) (71). This level of anatomic obstruction would be below the threshold to elicit an increase in mPAP in patients without cardiopulmonary disease. In patients with prior cardiopulmonary disease, the RAP was reported to be an unreliable indicator of the magnitude of the embolic event and limited its usefulness in the assessment of extensive vascular obstruction and life-threatening disease. Therefore, it appears that there is no consistent relationship between the extent of embolic obstruction and right ventricular impairment in patients with previous cardiopulmonary disease. Hemodynamic and right ventricular function can be misleading as measurements of the effect of the embolic event, which clearly underscores that the assessment of the severity is predicated on the pre-embolic status of the patient as illustrated in Figure 114.1.
Readily Available Diagnostic Studies
The development of a differential diagnosis in the case of the undifferentiated shock patient or existing patients in the ICU is usually predicated on elements derived from the history, physical findings, and readily available diagnostic studies to include a chest x-ray (CXR) study, arterial blood gas (ABG) analysis, and electrocardiogram (ECG). It is important to recognize the physiologic footprint that PE makes on these readily available studies to ensure that PE is hierarchically incorporated into the differential diagnosis of the unstable patient. Generating this differential diagnosis is often difficult in the critical care environment, given the inability to obtain a current history from the patient, multiple comorbidities masking physical findings, and coexistent disease that already compromises existing laboratory variables. Although multiple risk factors are additive, admission to an ICU by itself denotes a significant risk factor for VTE. In patients presenting to the ICU with undifferentiated shock or respiratory failure, it is imperative to review each specific case for risk factors that may contribute to the development of PE. The previously defined hydraulic model of the circulation allows for a physiologic characterization of the differential diagnosis. The constellation of elevated RAP with cool clamped extremities, indicative of low flow, against the background of relatively clear lungs and CXR isolates the hydraulic lesion to the right ventricle with a very limited differential diagnosis as illustrated in Figure 114.3. Occasionally, invasive hemodynamic measurements will be available, which should reflect an increase in the RAP, a low CO state in the shock population, associated with a low PCWP, and a high SVR.
Electrocardiogram
Since the sentinel description in 1935 by McGinn and White (73) of the S1Q3T3 pattern in a limited number of patients with PE-induced cor pulmonale, a plethora of ECG manifestation have been reported. However, several important points from large series regarding the ECG findings for PE may be helpful: First, a normal ECG is distinctly unusual, being reported in only a minority of patients in the UPET Trial without cardiopulmonary disease (14%) (74); a normal ECG was similarly appreciated in only 30% of patients in the PIOPED Trial (75). Rhythm disturbances are uncommon, and the incidence of atrial fibrillation and flutter as a presenting component of PE is exceedingly small as are first-, second-, or third-degree heart blocks. The most common ECG findings are related to abnormalities in the ST–T-wave segment. In UPET (74) and PIOPED (75), these changes occurred in 42% and 49% of patients, respectively. Recently, it has been shown that anterior T-wave inversion pattern is the most common abnormality in PE, occurring in 68% of patients. It was the ECG sign that was most correlative with the severity of the underlying embolic event, as 90% of the patients with anterior T-wave inversion had a Miller Score exceeding 50% (mean 60%), and 81% of those had an mPAP elevation exceeding 30 mmHg. The early appearance of the T-wave inversion was reported to be an even stronger marker of the severity of the event and similarly correlative with the efficacy of thrombolytic therapy given the T-wave normalization that occurred in patients who had successful thrombolytic therapy (76).
Chest Radiograph
Although the chest radiograph (CXR) cannot be effectively used to include and exclude PE, it is helpful in contributing to the diagnostic assessment by excluding other diseases that may mimic PE and defining abnormalities that necessitate further evaluation; further, it may provide a crude assessment of severity (77). Similar to the ECG, a normal CXR in patients with angiographic-proven PE is unusual, occurring in only 16% and 34% of patients in the PIOPED (75) and UPET (78), respectively, who did not have cardiopulmonary disease. In PIOPED, there appeared to be an association between severity of the thromboembolic event and the radiographic findings defined by the relationships between PAP, oxygen saturation, and CXR findings when normal CXR views were compared to those with parenchymal and vascular abnormalities (77). Vascular abnormalities, including relative oligemia in the area of embolic event, were correlative with the severity of PE. Other findings on CXR suggestive of PE include abrupt cutoff of the pulmonary artery, relative or focal oligemia, and distention of the proximal portion of the pulmonary artery.
Arterial Blood Gas
It is important to recognize that hypoxia in PE is not uniform, as PaO2 readings greater than 80 mmHg were seen in approximately 12% of UPET patients and 19% of PIOPED patients (78,79). It is similarly important to recognize that a normal PaO2 does not exclude PE, occurring in approximately 14% of patients in the PIOPED Trial (80). In patients without underlying cardiopulmonary disease, it is likely that these small changes in oxygen saturation reflect low levels of severity in PE. In contrast to the almost linear relationship between PE severity and arterial oxygen saturation levels in patients without cardiopulmonary disease, there appears to be no correlation between the arterial oxygen saturation or PaO2 and magnitude of the embolic event in patients with cardiopulmonary disease. Given that many patients in ICUs have significant gas impairment and are maintained on mechanical ventilation, it is the change in the oxygen saturation or the requirement of escalating FiO2 that should prompt the consideration toward evaluation for PE. In ICUs that are capable of measuring dead space (Vd/Vt) or end-tidal CO2, these should similarly be used given the physiologic imprint of increased dead space with PE.
DIAGNOSTIC/THERAPEUTIC APPROACH
Risk Stratification
The contemporary approach to physiologic risk stratification is depicted in Figure 114.1. The combination of underlying cardiopulmonary status and embolic size that precipitates cardiac arrest is surprisingly associated with a mortality of only 70% in reported series. This underscores the necessity of aggressively pursuing patients with suspected underlying PE presenting with cardiac arrest, because approximately 30% of those patients with PE and cardiac arrest will survive. At the other extreme, it is equally underappreciated that the predicted mortality of patients with hemodynamically stable PE and normal right ventricle is very low when treated with appropriate anticoagulation. The combination of embolic size and cardiopulmonary status that precipitates decompensation resulting in shock is associated with 30% mortality. Although not well reported, syncope and emboli in transit have predicted mortalities just below that of a shock patient. Echocardiography is frequently used to risk-stratify patients with PE who are hemodynamically stable. However, it is important to recognize that the overwhelming majority of patients with right ventricular dysfunction and hemodynamic stability will do well with anticoagulation alone. As is evident in Figure 114.1, there is a spectrum of presentations related to hemodynamically stable patients with PE that may include patients with incipient shock and those with insignificant dilatation of the right heart. It is important to recognize that an anatomic obstruction of approximately 30% is necessary to provoke elevations in PAP. Similarly, literature related to echocardiography reveals that an obstruction of 30% is needed to precipitate right ventricular dilatation.
The presence of hemodynamic deterioration or shock in a patient with PE represents the failure of both compensatory mechanisms to maintain forward flow and is associated with significant increases in mortality. Consequently, the presence of shock has traditionally been used as a discriminator to define the likelihood of survivorship from PE. The presence of shock in patients with PE is associated with a threefold to sevenfold increase in mortality (81,82). It is underappreciated that the vast majority of patients with anatomically massive PE do not present in shock. Case series have reported a majority of patients without underlying cardiopulmonary disease and associated anatomically massive PE present with a normal CO (83). Similarly, it is important to recognize that hemodynamically stable patients who are not in shock, who have experienced submassive or massive PE, have similar mortality rates (81,82). An anatomically massive PE, unless accompanied by physiologic decompensation resulting in shock and hemodynamic instability, does not appear to be associated with increased mortality.
Figure 114.5 represents a diagnostic/therapeutic algorithm based on the presence or absent of shock. In all patients presenting with PE, therapeutic anticoagulation should be undertaken on presentation provided there are no contradictions to anticoagulation. The therapeutic effect of heparin is related to the ability to prevent further clot propagation and the prevention of recurrent of PE. Insofar as the risk of recurrent thromboembolic event is highest in the period immediately after PE, and because recurrent thromboembolic events are the most common cause of death in hemodynamic stable patients, it is pivotal to adequately and appropriately achieve a therapeutic level of anticoagulation as soon as feasible. Given the risks of bleeding associated with critical illness, unfractionated intravenous heparin is recommended because of the short half-life and the ability to reverse the therapeutic effect of heparin with protamine. Low–molecular-weight heparin, although appealing in the outpatient treatment of DVT and stable PE patients cannot be readily reversed.
In patients without evidence of hemodynamic stability, spiral CT scanning has supplanted V/Q scanning as a diagnostic modality of choice in PE. In patients with elevated creatinine or inability to tolerate a CT scan, V/Q scanning represents a reasonable alternative given previous experience using this technique. Pretest probability characterizations are pivotal in the diagnosis of PE whether CT or V/Q scanning are used. Pretest probability can be characterized by the use of scoring systems or based on clinical judgment. The combination of a high pretest probability with a high-probability V/Q scan confirms the diagnosis of PE. Similarly, a low-probability V/Q scan in conjunction with a low clinical pretest probability effectively excludes PE. Any other combination of pretest probability and scan probability usually requires further testing to include and exclude PE. Although spiral CT scanning has been used to either exclude or confirm PE, there is an evolving literature that suggests spiral CT scanning can be used to define the severity of PE. Recent reports have been able to characterize the extent of pulmonary artery obstruction (84,85). Similar to the Miller and Walsh scores, which defined the extent of anatomic obstruction, there has been variability in reports of outcome related to the extent of obstruction. Measurements that are available from the CT scan include the calculation of the RV/LV axis ratio, the diameter of the right ventricular chamber, assessment of pulmonary artery diameter, and reflux of contrast material into the IVC (86). The predictive value of CT for acute PE was recently evaluated in a systematic review and meta-analysis of 14 studies with 13,612 patients undergoing diagnostic CT imaging for PE. An abnormally increased RV/LV diameter ratio measured on the transverse section was associated with a 2.5 OR for increased all-cause mortality, 5.0 OR for PE-related mortality, and 2.3 OR for adverse outcome. Thrombus load (OR 1.6) and central location (OR 1.7) were not predictive of all-cause mortality, although associated with adverse clinical outcomes (87). Similar to RV dilatation on echocardiography, it would appear that RV dilatation on CT is very sensitive but not specific for mortality outcome, as the vast majority of patients with RV dilatation will survive with appropriate anticoagulation. With spiral CT scanning as the modality of choice for the diagnosis of PE, CT severity stratification will more frequently be used to define risk stratification.

FIGURE 114.5 Diagnostic therapeutic approach to pulmonary embolism. AMI, acute mesenteric ischemia; CT, computed tomography; DVT, deep vein thrombus; LV, left ventricle; RV, right ventricle; TEE, transesophageal echocardiography; TTE, transthoracic echocardiography; US, ultrasound; V/Q, ventilation/perfusion.
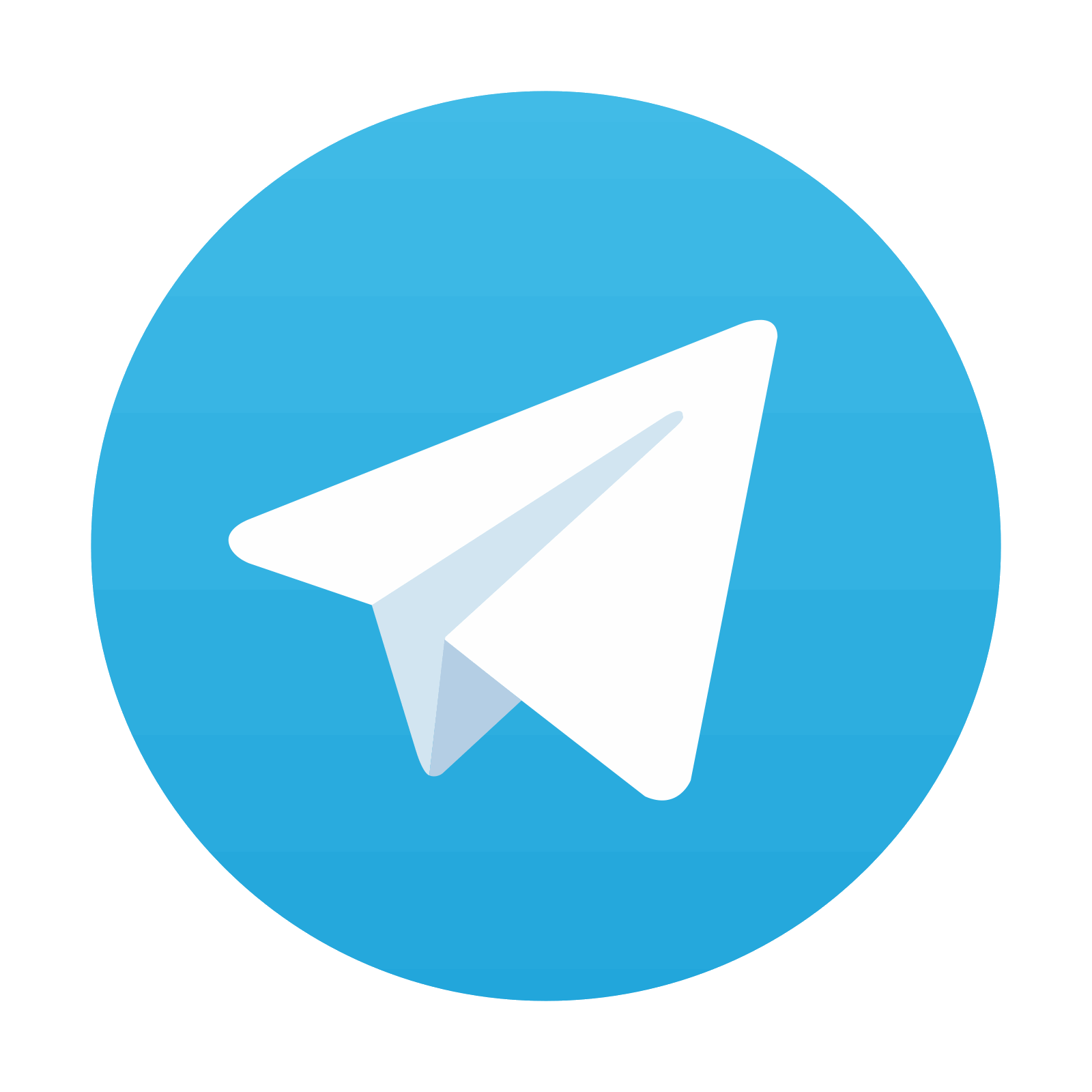
Stay updated, free articles. Join our Telegram channel
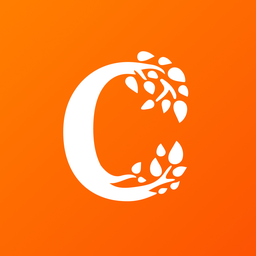
Full access? Get Clinical Tree
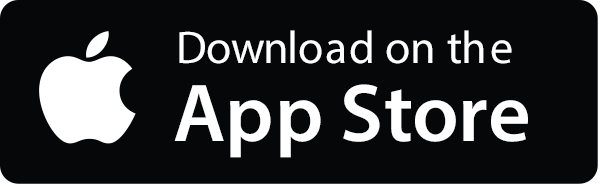
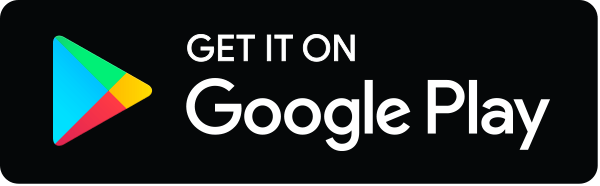