KEY POINTS
The altered pharmacokinetics of critically ill patients can greatly impact antimicrobial exposures. Recognizing these changes and optimizing antimicrobial administration to make certain appropriate pharmacodynamic targets are reached is crucial in ensuring successful outcomes.
Understanding the impact of the MIC of the pathogen on overall free-drug exposures is important to be able to reach required pharmacodynamic targets of efficacy. Ultimately, organisms with high MICs will require a larger free-drug exposure compared with organisms with lower MICs.
Patients with augmented renal function will exhibit enhanced clearance of antimicrobials, particularly β-lactams, and are at risk for subtherapeutic exposures. Therefore, these patients often require higher doses and more frequent administration of the antimicrobial.
Antimicrobial stewardship focused on disease state management and selecting the appropriate antimicrobial therapy for the infecting pathogen is essential in preventing poor outcomes. In addition to poor outcomes, failure to treat infections appropriately can lead to the emergence of resistant organisms that become increasingly difficult to treat.
Given the vast majority of antimicrobials are renally cleared, concentrations of antimicrobials are affected by continuous renal replacement therapy and therefore dosing should be modified accordingly to obtain adequate target exposures.
INTRODUCTION
Ensuring adequate antimicrobial treatment to critically ill patients remains a significant challenge. Physiological changes within the patient and resistant pathogens make it increasingly difficult to successfully treat infections. Optimizing the way antimicrobials are administered and understanding the principles of pharmacokinetics and pharmacodynamics can significantly change the outcomes of a patient’s clinical course. In addition, optimizing regimens can help minimize the development of resistance. Herein the various classes of antimicrobials used within the ICU and optimization strategies are described.
PHARMACOKINETICS AND PHARMACODYNAMICS IN CRITICALLY ILL PATIENTS
The major changes in pharmacokinetic parameters of critically ill patients include alterations in volume of distribution (Vd) and clearance (Cl).1,2 Subsequently, these alterations affect the concentrations of antimicrobials in the body and the extent to which they are cleared. The Vd is the volume in which the total amount of drug would have to be evenly distributed in to equal the same concentration as in the plasma. The toxins produced by various bacteria often lead to endothelial damage and result in increased capillary permeability. This leads to the phenomenon of “third spacing” where fluid shifts into the interstitial space from the intravascular space. These fluid shifts will increase the Vd of hydrophilic antimicrobials. Generally speaking, hydrophilic antimicrobials have a low Vd and therefore are greatly affected by these fluid shifts. Since lipophilic antimicrobials have a larger Vd, they typically distribute further into tissues and are less affected by these fluid shifts. Patients in the ICU often have hypotension as a result of septic shock, which requires the administration of fluid boluses. Additionally, heart failure and renal failure lead to more edematous states where patients can retain large amounts of fluid. These situations also lead to increases in Vd of hydrophilic drugs. Changes in protein binding can also have a substantial effect on the Vd, especially for drugs that are highly protein bound. Only unbound or free drug is microbiologically active. Hypoalbuminemia in critically ill patients can result in decreased binding of drugs and subsequently higher free concentrations of drugs. While free drug will distribute into tissues, critically ill patients often have greater amounts of fluid in the interstitial space causing the antimicrobial concentrations in the tissues to remain low.3
The administration of large volumes of fluid and use of vasopressors leads to a hypermetabolic state in which cardiac output and glomerular filtration rate are increased. It has been shown in animal studies that increases in cardiac output result in increases in renal blood flow. The term often used to describe this enhanced elimination is augmented renal clearance.4 These physiological changes affect the clearance of drugs and can lead to subtherapeutic levels of antimicrobials that are typically cleared renally. In contrast, decreased organ perfusion in the presence of end organ damage can lead to kidney and/or liver failure in which concentrations of these antimicrobials would be increased. Inadequate clearance or metabolism of these drugs would lead to accumulation and potential toxicity. Typically, equations such as Cockroft-Gault are used to estimate renal function; however, these are often not good predictors of renal function in critically ill patients due to the acute and rapid changes such patients often experience. Since many antimicrobials are dosed based on renal function it is even more challenging to ensure adequate doses are being administered. The most accurate way to calculate renal function is the use of 8- or 12-hour creatinine collections.2 In situations where renal replacement therapy is utilized, careful consideration of timing and supplemental dosing post-dialysis would be needed depending on the antimicrobial agent.
Understanding the pharmacokinetics factors that affect drug concentrations is essential in ensuring drugs are delivered to the target areas (Fig. 61-1). However, understanding the pharmacodynamics is just as important to ensure clinical successes. Ideally, dosing regimens that maximize the rate of response and minimize the development of resistance should be employed. Different classes of antimicrobials have different target pharmacodynamic (ie, relationship between drug concentration and antimicrobial killing effects) predictors and depending on the infecting organism different levels of these pharmacodynamic predictors would be necessary for adequate eradication of the bacteria. These pharmacodynamic predictors include (1) the time that drug concentrations remain above the MIC (T>MIC), (2) the ratio of the area under the plasma concentration-time curve (AUC) to the MIC (AUC:MIC), (3) the ratio of the Cmax (peak concentration) to MIC (Cmax:MIC).5 Because the free-drug concentration is considered the biologically active component these parameters are often displayed as fT > MIC, fAUC:MIC, or fCmax:MIC.
The percentage of the dosing interval that drug concentrations remain above the MIC is the driver of efficacy for a commonly administered class of antibiotics known as β-lactams that is represented by the cephalosporins, penicillins, and carbapenems. In situations where patients have a large volume of distribution or when the MIC of the organism is high, larger doses may be necessary to achieve adequate concentrations. While achieving the appropriate concentration is important, simply administering larger doses is not adequate. The extent of bacterial eradication when using these drugs is time dependent and therefore maintaining that concentration is essential. Since many of these antimicrobials have short half-lives, administering the dose more frequently is often necessary. Additionally, in the context of augmented renal function, patients may be clearing the drug much faster and therefore will require the use of higher doses and more frequent dosing. Another strategy is to administer the dose over a longer duration of time, commonly known as prolonged (administering each dose over 3-4 hours) or continuous infusion (administering the entire daily dose as a 24-hour infusion). This dosing strategy has been shown to increase the fT>MIC and therefore improve patient outcomes by increasing microbiological and clinical success rates.6,7 It is important to note that these dosing strategies can be challenging especially in critically ill patients receiving multiple medications where drug compatibility and limited venous access are present. Moreover, when considering these techniques, the stability profile of the antimicrobial in the chosen intravenous solution should also be taken into account to ensure minimal loss of potency during the preparation and administration of therapy.
AUC:MIC ratio has been shown to be the pharmacodynamic driver of antimicrobials such as fluoroquinolones, vancomycin, azithromycin, linezolid, and daptomycin.5 The rate of killing by these antimicrobials is considered to be a hybrid of concentration and time; thus, this pharmacodynamic parameter integrates the entire exposure profile. Depending on the antimicrobial and the organism being treated a specific AUC:MIC ratio is required to optimize antimicrobial killing. Unlike β-lactams, these antimicrobials may be dosed less frequently because time of exposure in and of itself is less critical, however the selection of the most appropriate dose is still paramount to ensure an adequate AUC (exposure) is being achieved. The goal of therapy is to maximize the exposure of antimicrobial therapy. Aminoglycosides display concentration-dependent killing and since this is the predominant driver of efficacy they are dosed to achieve a targeted peak or maximum concentration, based on the relationship of the MIC of the infecting organism (Cmax:MIC). Similarly to β-lactams, since many of these antimicrobials are renally eliminated the overall exposures achieved is partly dependent on the patient’s renal function. Unlike the β-lactams, many of these antimicrobials (ie, vancomycin, aminoglycosides, daptomycin) are associated with more significant toxicities such as nephrotoxicity, ototoxicity, and rhabdomyolysis with supratherapeutic concentrations. Thus optimizing the toxicodynamic profile as well as the pharmacodynamic is a challenging aspect to dose optimization in the critically ill patient.
ANTIMICROBIAL CLASSES
β-Lactams are the most commonly prescribed class of antimicrobials in the ICU.8 Typically, these drugs are hydrophilic and therefore have a relatively low volume of distribution. Clearance of most antimicrobials in this class depends on the patient’s renal function. The extent of bacterial killing is time dependent and therefore the pharmacodynamic parameter of interest is fT>MIC as described above.5 The changes in volume of distribution and clearance in critically ill patients can significantly affect the extent of fT>MIC for β-lactams.2,8 Volume of distribution is often increased as a result of capillary leak, positive-pressure ventilation, transfusions, and other interventions critically ill patients may receive. With an increase in volume of distribution drug concentrations will be reduced. Since renal blood flow is often increased in patients with septic shock, renal clearance may also be elevated, contributing to subtherapeutic levels of β-lactams. Although creatinine clearance is often calculated using equations such as Cockcroft-Gault, these may underestimate the clearance, in which case a creatinine clearance collection may be more accurate. On the other hand, patients with renal dysfunction will not clear the drug and therefore require fewer doses of antimicrobials due to an extended half-life. Overtly high concentrations of β-lactams can potentially lead to toxicities such as renal failure and seizures. Inadequate dosing as a result of these pharmacokinetic changes can lead to untreated infections, poor outcomes, a rise in resistant pathogens, and potential toxicities.
Traditional dosing of β-lactams usually involves doses administered over 30 minutes up to four times a day depending on the patient’s renal function. However, this dosing strategy may not achieve the adequate fT>MIC targets necessary for bacterial killing. Since β-lactams display time-dependent killing administering the antimicrobial over an extended period of time increases the fT>MIC and therefore the potential to optimize clinical and microbiological outcomes.
Penicillins: Piperacillin-tazobactam is an extended spectrum penicillin with activity against Enterobacteriaceae, Pseudomonas aeruginosa, and many anaerobes. With broad gram-negative coverage it is often used empirically in patients with sepsis, ventilator-associated pneumonia, and other serious infections. While dosing can vary based on indication and renal function, ICU patients typically receive 4.5 g every 6 hours due to the severity of their infections. Depending on the decline in renal function, the dosing interval should be further extended to every 8 or 12 hours. The volume of distribution and clearance of piperacillin in ICU patients have been shown to be increased in previous pharmacokinetic studies and therefore aggressive dosing is necessary.9,10 Given these changes in pharmacokinetic parameters, fT>MIC can potentially be reduced, resulting in unsuccessful outcomes, especially for pathogens with high MICs. Penicillins typically require at least 50% fT>MIC to reach maximal bactericidal activity and this may not always be achieved with conventional intermittent dosing. Administering larger doses as previously studied with piperacillin-tazobactam will provide higher overall exposures.11 Additionally, continuous infusion or extended infusion administration are two ways to better optimize time-dependent antibiotics such as piperacillin-tazobactam. Continuous infusion dosing of piperacillin-tazobactam can range from 9 to 18 g daily depending on the type of infection, with higher doses used for bacteremia and pneumonia, while lower doses are typically used for skin and skin structure infections and community-acquired intra-abdominal infections. Extended infusion dosing is usually administered as standard 3.375 or 4.5 g doses; however, the duration of the infusion is extended to 3 to 4 hours. Monte Carlo simulations have shown that using extended infusion dosing (ie, 4-hour infusions every 8 hours) helps achieve the pharmacodynamic target at higher MICs versus intermittent dosing.12 This extended infusion dosing strategy has been shown to decrease mortality and median length of stay in patients with APACHE II scores ≥17 in a retrospective cohort study.12 Another study with continuous infusion piperacillin-tazobactam showed that fT>MIC was higher with continuous infusions versus intermittent dosing (100% vs 62%, respectively).13 Other studies have shown favorable clinical outcomes especially in the critically ill population, including higher rate of clinical cure and lower mortality with continuous or extended infusion piperacillin-tazobactam.14-16
Carbapenems: With activity against a number of clinically significant organisms such as P aeruginosa, Acinetobacter spp, and β-lactamase-producing bacteria, carbapenems are often used in the ICU.17,18 Carbapenems provide the broadest gram-negative coverage of all β-lactams and unlike other β-lactams, carbapenems are stable against extended-spectrum β-lactamases and AmpC β-lactamases. Resistance mechanisms such as ESBLs are of particular concern because they are increasing worldwide, including in the United States, and current treatment options are very limited. Similar to other β-lactams, carbapenems exhibit time-dependent bactericidal activity and require approximately 40% fT>MIC, and therefore administering these agents as prolonged infusions can help increase fT>MIC and ultimately efficacy. The pharmacokinetics of carbapenems in critically ill patients are likely to change in a similar pattern as other β-lactams with increases in volume of distribution and clearance.2 Currently, there are four carbapenems available in the United States: imipenem, meropenem, ertapenem, and doripenem. Each of these differs slightly in their spectrum of activity and pharmacologic properties.17 While carbapenems typically are well tolerated, the potential for seizures have been reported with imipenem/cilastatin. Impaired renal function, high doses, increased age, history of seizures, or preexisting CNS diseases/infections are the most common risk factors for seizures. The documented incidence from phase III trials and post-marketing surveillance is 1.5% to 2%. Meropenem, doripenem, and ertapenem have a lower risk of seizures compared with imipenem.
Imipenem was the first carbapenem approved in the United States in the 1980s. Imipenem can be hydrolyzed and inactivated by dehydropeptidase I (DHP-1), an enzyme found at the renal brush border cells, therefore it must be coadministered in a 1:1 with a DHP-1 inhibitor, cilastatin. Depending on the severity of infection and renal function typical dosing of imipenem is 250 to 1000 mg every 6 to 8 hours administered as a 30- to 60-minute infusion. At room temperature imipenem is stable for only 4 hours, making it very difficult to administer as a prolonged infusion. Additionally, the potential for seizures at higher doses may prevent the use of imipenem for infections that require more aggressive dosing, especially in patient populations at greater risk (ie, CNS infections, concomitant medications that lower seizure threshold, renal dysfunction) for seizures. Previous imipenem therapy is an independent risk factor for the presence of imipenem-resistant P aeruginosa.19,20 Another study in febrile neutropenic patients showed that relapses with Pseudomonas were more common with imipenem (2 g/d) compared with ceftazidime.21 Similar to other carbapenems, imipenem is not active against methicillin-resistant staphylococci or vancomycin-resistant enterococci.17 Among clinically relevant gram-negative bacteria, imipenem is not active against Burkholderia cepacia and Stenotrophomonas maltophilia.
Unlike imipenem, meropenem stability at room temperature is enhanced and therefore prolonged infusions are a more viable option.22 Meropenem dosing ranges from 500 to 2000 mg every 8 hours as a 15- to 30-minute infusion for patients with normal renal function. Due to the overall stability, prolonged infusions of meropenem are generally limited to 3 hours. Monte Carlo simulations have been used to help optimize dosing of meropenem in critically ill patients based on renal function.23 Simulations comparing a 30-minute infusion with a 3-hour prolonged infusion showed that prolonging the infusion increases the probability of achieving 40% fT>MIC and required less total daily dose. Depending on the MIC of the pathogen, this can have substantial effects on clinical outcomes. Prolonged infusion meropenem at a dose of 2 g every 8 hours given as a 3-hour infusion in patients with normal renal function has also been utilized in a clinical pathway for patients with ventilator-associated pneumonia.24 Based on the organizations’ unit-specific ICU data and pharmacodynamic modeling approaches, prolonged infusion meropenem was incorporated in the empiric treatment of VAP. The implementation of this pathway significantly reduced infection-related length of stay and mortality. Meropenem has slightly better gram-negative coverage than imipenem as it is active against Burkholderia cepacia.17 Additionally, the chemical structure of meropenem makes it more difficult for P aeruginosa to develop resistance.
In contrast to meropenem and imipenem, doripenem is stable for 12 hours at room temperature, making it a more suitable option for prolonged infusions. Another advantage of doripenem is enhanced in vitro activity against P aeruginosa compared with imipenem and meropenem. Similar to meropenem, prolonging the infusion can help optimize outcomes, especially in situations where the infecting pathogen is likely to have a high MIC. For most infections, doses of 500 to 1000 mg every 8 hours are used for patients with normal renal function. However, for cystic fibrosis patients, it has been shown that higher than recommended doses of doripenem and meropenem are likely needed as these patients are often infected with multidrug-resistant organisms and have a vastly different pharmacokinetic profile.25 Doripenem doses such as 2 g every 8 hours are not uncommon in this patient population.
Common indications for use include nosocomial pneumonia and intra-abdominal infections. Of note, a recent study comparing a 10-day course of imipenem-cilastatin versus a 7-day course of doripenem for the treatment of ventilator-associated pneumonia showed lower clinical cure and higher mortality in the doripenem arm.26 This was attributed to the short course of doripenem administered and therefore careful consideration is recommended when determining both the adequacy of dosing regimen as well as the duration of therapy to optimize outcomes.
Ertapenem is the most unique among the four carbapenems. Pharmacokinetically it has a longer half-life and is significantly more protein bound allowing for once daily administration. The most commonly utilized dose of ertapenem is 1 g every 24 hours as a 30-minute infusion. While 1 g doses are generally sufficient for efficacy, in the context of augmented renal function, dosing every 12 hours may be required to produce adequately high exposures.27 While this agent has good activity against Enterobacteriaceae and has shown good outcomes relative to the Group 2 carbapenems (imipenem, meropenem) against ESBL-producing organisms, this compound has no appreciable activity against P aeruginosa and Acinetobacter.28 Due to its limited spectrum of activity against these prominent ICU pathogens ertapenem is not typically used as empiric therapy in the critical care setting. However, use of this agent for de-escalated therapy against enzyme-producing bacteria may reduce the antipseudomonal pressure exerted by the use of the other Group 2 carbapenems.28 This strategy should be considered when possible as the increasing use of these Group 2 carbapenems (imipenem, meropenem, doripenem) has resulted in escalating levels of resistance across the globe for P aeruginosa and Acinetobacter.
Cephalosporins: Cephalosporins as a class cover a broad range of organisms and are fairly well tolerated. They are typically classified as first, second, third, fourth, and fifth generation with varying spectrum activity among the generations. First-generation cephalosporins, such as cefazolin, have activity against most gram-positive cocci except enterococci and methicillin-resistant S aureus. Gram-negative coverage includes most strains of Escherichia coli, Proteus mirabilis, and Klebsiella pneumoniae, but there is no activity against organisms such as Acinetobacter spp and Pseudomonas aeruginosa. Because of its narrow spectrum of activity, cefazolin is not typically used as empiric therapy in the critical care setting. Second-generation cephalosporins (cefoxitin and cefuroxime) have slightly better gram-negative bacilli coverage compared with the first generation. Similar to cefazolin, these agents are not often used as empiric therapy due to their limited spectrum of activity. Third-generation cephalosporins (cefotaxime, ceftriaxone, and ceftazidime) are much more active against gram-negative bacilli such as Enterobacteriaceae, Neisseria, and Haemophilus influenzae. Compared with first-generation cephalosporins, these agents have less activity against gram-positive organisms. Of the three parenteral third-generation cephalosporins, ceftazidime is slightly different in that it has activity against P aeruginosa. Similar to ceftazidime, cefepime, a fourth-generation cephalosporin, also has activity against P aeruginosa. Cefepime also has considerably more gram-negative coverage against enzyme producing Enterobacteriaceae. Lastly, ceftaroline is the newest antimicrobial in the cephalosporin class and is considered an anti-MRSA cephalosporin. Ceftaroline is the only cephalosporin with activity against MRSA, including strains such as vancomycin intermediate S aureus (VISA) and heteroresistant VISA. Its gram-negative activity is similar to that of ceftriaxone and it does not have activity against P aeruginosa. The most commonly used cephalosporins in the ICU are ceftazidime and cefepime due to their broad spectrum of gram-negative activity, which is inclusive of P aeruginosa. Both ceftazidime and cefepime are dosed aggressively because patients are likely to have altered pharmacokinetics, which puts them at risk for inadequate drug exposure. Both agents are typically dosed at 2 g every 8 hours for patients with normal renal function. While they can be administered as intermittent infusions, similar to other β-lactams, there is substantial benefit in administering these agents as extended infusions.
Similar to penicillins and carbapenems, fT>MIC is considered the predictive pharmacodynamic parameter for cephalosporins. Most studies have associated an fT>MIC of 50% to 70% with successful clinical outcomes for gram-negative infections.29,30 Prolonged infusions of cephalosporins, particularly with ceftazidime and cefepime, have been shown to produce beneficial clinical outcomes. Three-hour infusions of cefepime 2 g every 8 hours in the VAP clinical pathway mentioned above reduced infection-related mortality and infection-related length of stay. Most notably, some of these patients achieved successful outcomes despite being infected with P aeruginosa isolates with MICs at or above the breakpoint.
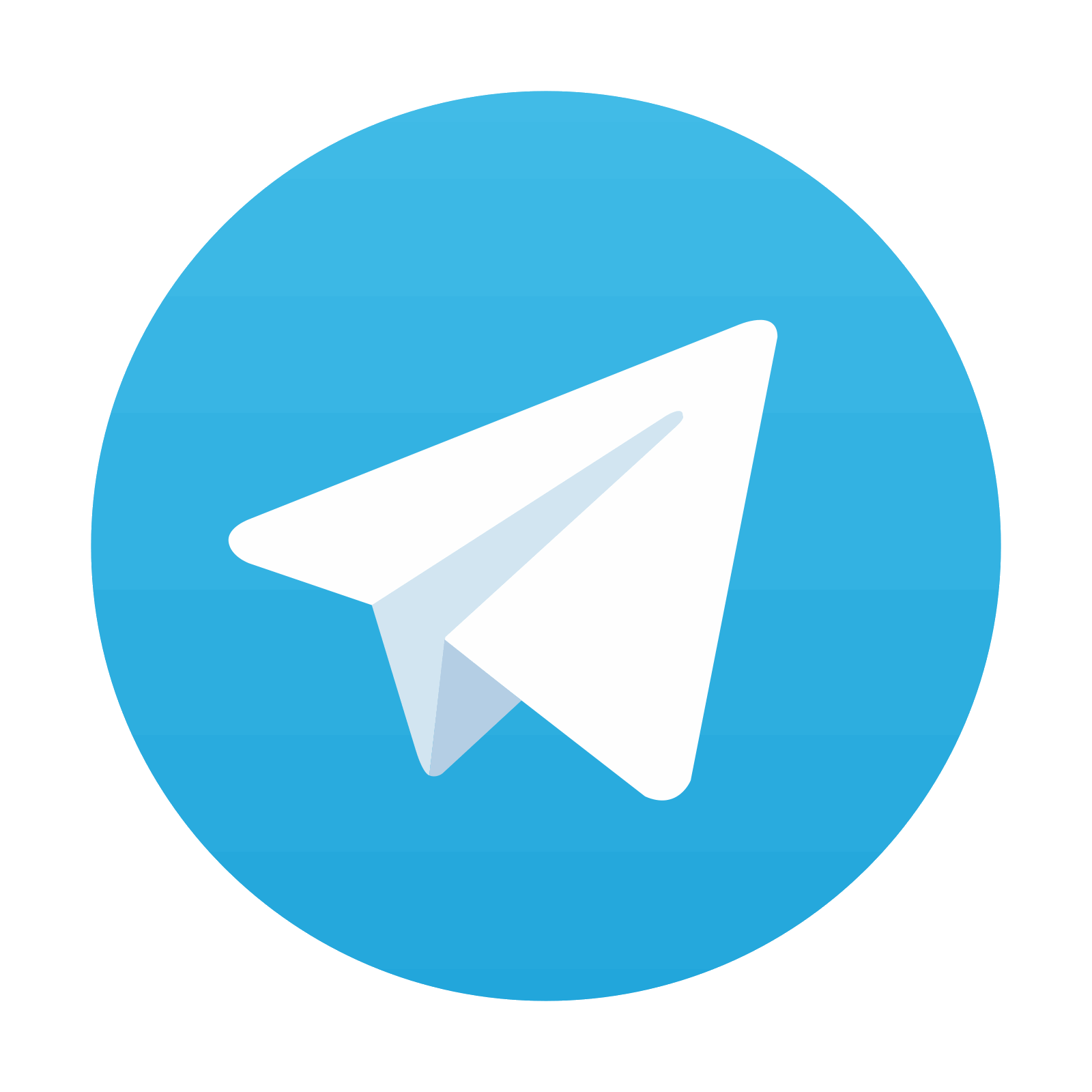
Stay updated, free articles. Join our Telegram channel
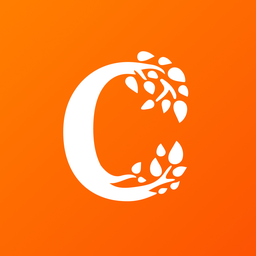
Full access? Get Clinical Tree
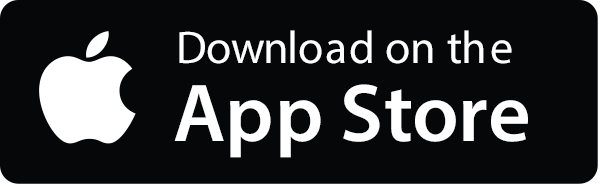
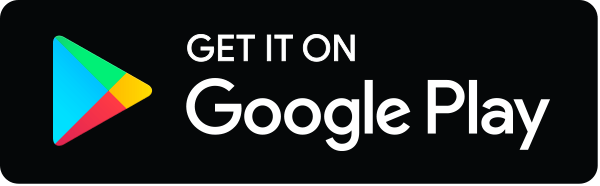