The discovery of inhalation anesthetics has a colorful history and began as early as the late 1700s with nitrous oxide. One initial use was in the treatment of dental pain and was later in combination with oxygen as an anesthetic. Chloroform was discovered in 1831 by an obstetrician, James Simpson, who used it to relieve labor pain for Queen Victoria for her eighth and ninth deliveries in the mid-1800s. Although diethyl ether was discovered in the 1600s, it was not used as an anesthetic until the mid-1800s in the United States, most notably by William Morton at Massachusetts General Hospital in Boston, to successfully anesthetize a patient for a mandibular tumor resection. In search of better anesthetics, development of additional agents continued. Ethyl chloride, an agent used as a topical anesthetic to freeze painful tissue, was later found to render patients unconscious. Additional agents developed during the late 1800s were ethylene and cyclopropane, and during the early 1900s, divinyl ether was developed.
Major drawbacks to these early inhaled agents were they were pungent, flammable, and with selected drugs, associated with substantial hepatoxicity and/or cardiotoxicity, making them less desirable to use. In the 1950s, fluorinated hydrocarbons were introduced as nonflammable alternatives and have remained the mainstay of potent inhaled agents. Since then, numerous fluorinated hydrocarbon compounds have been evaluated, with several introduced into clinical use (enflurane, methoxyflurane, and halothane). Those with the best kinetic profile and minimal toxic side effects remain in use today (isoflurane, sevoflurane, and desflurane).
The mechanism of action for potent inhaled agents is not well understood and has been the subject of debate since the agents were discovered to be anesthetics. Unlike intravenous anesthetics, where pharmacologic action is associated with a drug binding to a receptor and triggering an effect, potent inhaled agents do not appear to have specific receptor targets. There are several theories. Researchers have suggested that potent inhaled agents deform lipid membranes such that they alter function of lipid proteins that play a role in neurotransmitter function within synaptic clefts. Inhaled anesthetics may act at multiple sites, making it difficult to pin down their exact mechanism, but it may be best described by physical chemistry (ie, swelling of nerve cell walls) and not chemical bonding between a drug and receptor.1
Important elements of inhaled agent pharmacokinetics are presented in Chapter 2. Inhaled agent kinetics (time course of drug concentration in response to dose) are a function of drug delivery as well as pulmonary ventilation and perfusion. A detailed description of factors that influence inhaled agent pharmacokinetics is presented in Figure 8–1.
Figure 8–1
Schematic representation of factors that influence inhalation agent pharmacokinetics. The inspired (inhalation agent delivered is a function of vaporizer setting, fresh gas flow, and circuit volume. The alveolar drug concentration is a function of anesthetic uptake, pulmonary ventilation, and the second gas effect and concentration effect (Chapter 2). Uptake is determined by the blood–gas partition gas coefficient, the difference in drug partial pressure between the alveolar gas and venous blood, and the cardiac output (specifically the rate of blood perfusion of ventilated alveoli). Arterial drug concentration is a function of lung ventilation and perfusion. Drug delivery to the central nervous system is a function of arterial drug concentration, tissue perfusion, and blood–tissue partition coefficients. Drug uptake at tissues of interest (ie, brain and spinal cord) is dependent on the partial pressure difference between arterial blood and tissue. (Reproduced with permission from Butterworth JF, Mackey DC, Wasnick JD: Morgan, Mikhail, & Murray, Clinical Anesthesiology, 5th edition. McGraw-Hill, 2013.)

Factors that influence drug uptake include alveolar ventilation, blood–gas partition coefficients (Table 8–1), and cardiac output. Alveolar ventilation is a function of the tidal volume, dead space volume, and respiratory rate. Figure 8–2 presents a set of simulations that plot the predicted effect-site concentration of isoflurane for 3 different alveolar minute volumes (3, 6, and 12 L/min). The time required to reach 90% of the maximal effect-site concentration was 36, 19, and 12 minutes, respectively, for each alveolar minute volume. As will be described below, the effect-site concentration is not to be confused with the end-tidal concentration, especially during non–steady-state conditions.
The blood–gas partition coefficient is a reflection of drug solubility. The coefficient represents the ratio of gas amount (drug) in air versus blood. For example, the blood–gas coefficient for desflurane is 0.42, indicating that at equilibrium at 37°C, a certain volume of blood (eg, 5 mL) will hold 42% of the desflurane of an equivalent volume of air (eg, 5 mL).1
Anesthetics with a low blood–gas partition coefficient (low solubility) quickly reach maximal content in blood and have a rapid onset and offset time (ie, nitrous oxide) compared to those with a high blood–gas coefficients (ie, isoflurane).
Inhalation agent elimination is a function of primarily by exhalation once delivery is terminated. Other factors include biotransformation and loss from skin tissue to air directly. Both of these contribute little to the rate of partial pressure drop once drug is discontinued. Biotransformation is minimal for modern inhalation agents but was more pronounced with highly metabolized drugs previously used, such as methoxyflurane and to a lesser extent halothane.1
Like delivery, elimination from the brain via the lungs is largely dependent on central nervous system perfusion, cardiac output, tissue–blood and blood–gas partition coefficients, lung perfusion and ventilation, and the removal of drug from the anesthesia circuit. Anesthesiologists can influence this process using a few techniques to enhance drug removal. Some of these include increasing fresh gas flow to wash out exhaled drug via the drug scavenging system and ensuring adequate ventilation and lung perfusion (typically using blood pressure and heart rate as a surrogate measure of cardiovascular function).
Researchers have devised a tool to hasten volatile anesthetic removal involving rebreathing carbon dioxide to increase cerebral blood flow and double minute volume while avoiding hypocarbia.2,3 Using this technique, elevating the partial pressure of carbon dioxide, via a rebreathing circuit, to 55 mm Hg and hyperventilating decreased emergence times for isoflurane; this worked to a lesser extent for sevoflurane and desflurane. For example, in 20 patients undergoing anterior cruciate ligament repair, wake-up times were 50% faster (7 minutes earlier) with hyperventilation hypercarbia. This device may be useful for rapid emergence following anesthetics with a long duration; procedures that require high-dose inhaled agents; or abrupt, perhaps unanticipated, end to surgery.
Given that volatile anesthetics are lipid soluble, one concern is the accumulation of drug with prolonged drug delivery. Figure 8–3 presents a simulation of sevoflurane with the vaporizer set to 2% for 1, 4, and 8 hours. Once the delivery was terminated and the vaporizer set to 0%, predictions from this simulation suggest that all of the concentrations will decrease by 50% in 5 to 6 minutes for drug delivery ranging from 1 to 8 hours (the 50% decrement time). However, it is important to point out that at the end of a 1-, 4-, and 8-hour anesthetic, the peak concentrations are progressively higher (1.6 vol%, 1.9 vol%, and 1.95 vol%).
Figure 8–2
Simulation of the influence of alveolar ventilation on isoflurane pharmacokinetics. Isoflurane is delivered with the vaporizer set to 1.2% for 1 hour. The vertical axis is normalized to 1.0. Effect-site concentrations (vol%) are presented for an alveolar minute volume (MV) of 3, 6, and 12 L/min. The first 20 minutes after starting drug delivery and 20 minutes following termination of drug delivery are presented in the left and right columns, respectively. Simulations assume a 30-year-old, 183-cm, 100-kg male; a fresh gas flow of 2 L/min; a MV of 6 L/min; and a normal cardiac output. The vaporizer is set at 1.2% for isoflurane, 2% for sevoflurane, and 6% for desflurane. Simulations used the following published pharmacokinetic and pharmacodynamic parameters to predict end-tidal and effect-site concentrations and drug effects: Johnson KB, Syroid ND, Gupta DK, et al. Evaluation of remifentanil sevoflurane response surface models in patients emerging from anesthesia: model improvement using effect-site sevoflurane concentrations. Anesth Analg, 2010;111(2):387-394; Lerou JG, Dirksen R, Beneken Kolmer HH, et al. A system model for closed-circuit inhalation anesthesia. I. Computer study. Anesthesiology. 1991;75(2):345-355; Lerou JG, Dirksen R, Beneken Kolmer HH, et al. A system model for closed-circuit inhalation anesthesia. II. Clinical validation. Anesthesiology. 1991;75(2):230-237; Lerou JG, Booij LH. Model-based administration of inhalation anaesthesia. 1. Developing a system model. Br J Anaesth. 2001;86(1):12-28; Lerou JG, Booij LH. Model-based administration of inhalation anaesthesia. 2. Exploring the system model. Br J Anaesth. 2001;86(1):29-37; Lerou JG, Booij LH. Model-based administration of inhalation anaesthesia. 3. Validating the system model. Br J Anaesth. 2002;88(1):24-37; Lerou JG, Verheijen R, Booij LH. Model-based administration of inhalation anaesthesia. 4. Applying the system model. Br J Anaesth. 2002;88(2):175-183; and Wissing H. Volatile anesthetic pharmacokinetics. Br J Anaesth. 2000;84:443-493.

Figure 8–3
Simulation of non–steady-state conditions for volatile agent levels delivered over 1 hour. End-tidal and effect-site vol % are presented as solid and dashed lines, respectively. The first 20 minutes after starting drug delivery and 20 minutes following termination of drug delivery are presented in the left and right columns, respectively. Simulations assumed the same conditions and patient demographics as in Figure 8–2.

Inhaled Agent | Minimum Alveolar Concentration (MAC)a | Blood–Gas Partition Coefficient |
---|---|---|
Isoflurane | 1.15% | 1.4 |
Desflurane | 6.0% | 0.42 |
Sevoflurane | 1.71% | 0.65 |
Nitrous oxide | 104% | 0.47 |
Xenon | 71% | 0.11 |
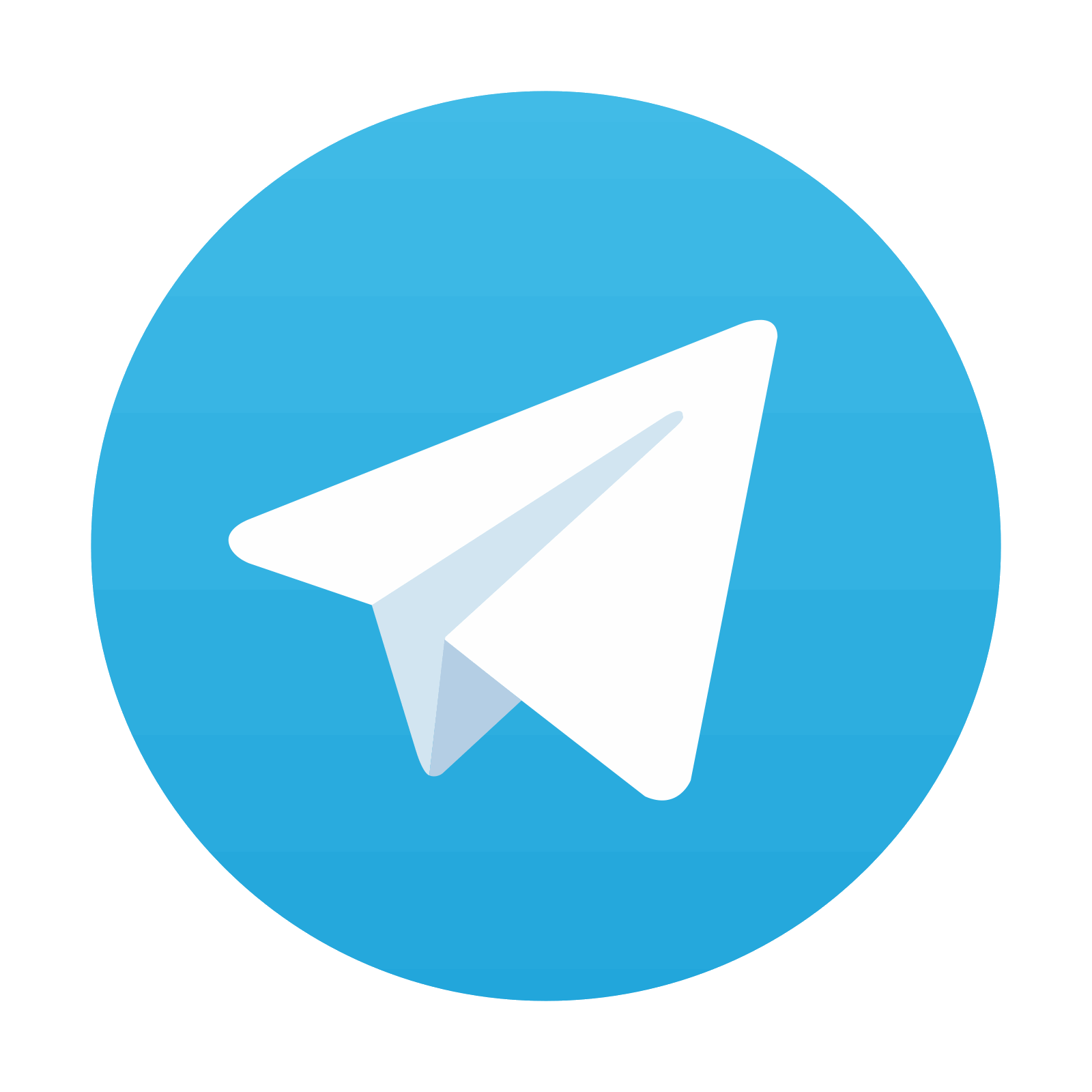
Stay updated, free articles. Join our Telegram channel
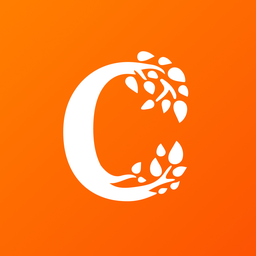
Full access? Get Clinical Tree
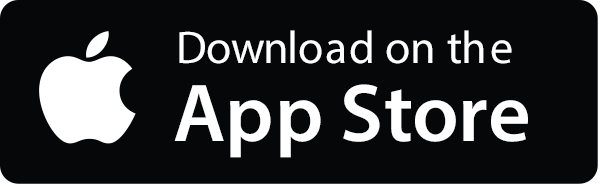
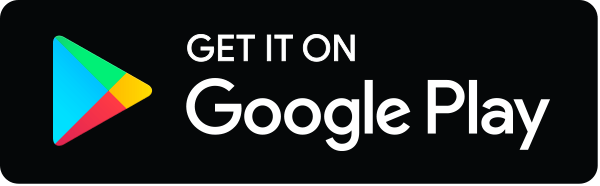
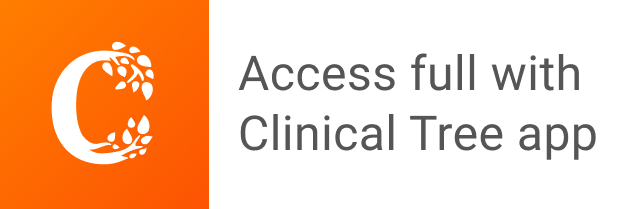