A typical neurone
Fibre type | Function | Fibre diameter (mm) | Conduction speed (ms–1) |
---|---|---|---|
Aα | Proprioception, somatic motor | 12–20 | 70–120 |
Aβ | Touch, pressure | 2–12 | 30–70 |
Aγ | Muscle spindle motor | 3–6 | 15–30 |
Aδ | Pain, temperature, touch | 2–5 | 12–30 |
B | Preganglionic ANS | < 3 | 3–15 |
C dorsal root | Pain, temperature, mechanoreceptors, reflex responses | 0.4–1.2 | 0.5–2 |
C sympathetic | Postganglionic ANS | 0.3–1.3 | 0.7–2.2 |
The ionic basis of membrane potential
Membrane potential at rest
As in other excitable tissues in the body, the electrical potential of a neurone in the resting state is more negative on the inside of the cell than on the outside. This polarity is maintained by the active transport of Na+ ions out of the cell, together with the active transport of K+ ions into the cell. However, there is a tendency for both ions to diffuse passively down their concentration gradient through leaky ion channels. In its resting state, the membrane is more permeable to K+ than to Na+ ions, and therefore more K+ ions leak out of the neurone than Na+ ions enter the cell. At the same time, the resting membrane is impermeable to anions. The result is that the interior of the neurone is more electronegative (–70 mV) than the outside. This is the resting membrane potential.
Resting membrane potential
The resting membrane potential in a neurone is –70 mV, the inside of the membrane being negative with respect to the outside.
This is due to the K+ permeability in the resting neurone membrane being greater than Na+ permeability.
Characteristics of the action potential
Neurones respond to a stimulus by transiently producing changes in ion permeability or conductance in the cell membrane. Ion conductance is defined as the reciprocal of electrical resistance of the membrane to a given ion, and it therefore reflects permeability. When the stimulus is below the threshold potential, the changes produced remain localised. However, when the stimulus reaches the threshold, the membrane becomes depolarised. When this depolarisation is propagated along the axon, it gives rise to an action potential. The latter can be divided into a number of phases (Figure 18.4).
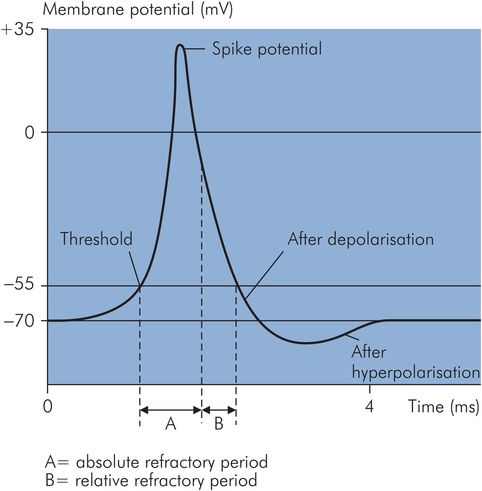
Different phases of an action potential
At the beginning of an action potential, the rate of depolarisation increases so that the inside of the cell becomes increasingly positive until it rises to a peak, then falls when repolarisation begins. This sharp rise and decline is called the spike potential, and it is due to an increase in Na+ conductance, so that Na+ ions diffuse down their electrical and concentration gradients.
However, there are three factors which limit the depolarisation process:
The Na+ channels open only very transiently.
As the inside of the cell becomes increasingly more electropositive, the initial gradients which facilitate Na+ influx disappear.
K+ conductance also increases. The rate of repolarisation slows down when the process is about 70% complete; this phase is known as after-depolarisation.
During the final recovery phase, there is a slight but prolonged overshoot after the resting potential is reached. This is due to the slow return of K+ conductance to normal (Figure 18.5). This phase is called the after-hyperpolarisation.
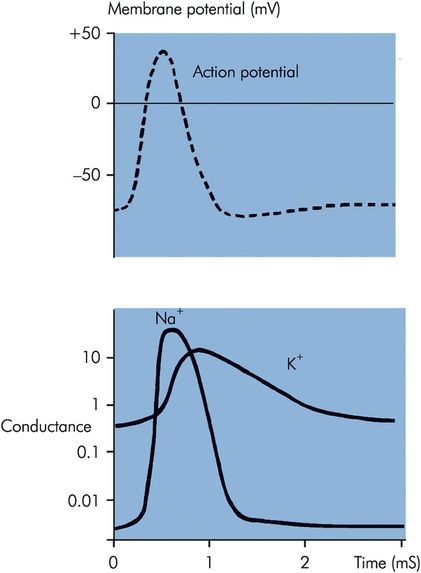
Changes in Na+ and K+ conductance during the course of an action potential
A neurone is said to be in the absolute refractory period when it is totally unresponsive to any stimulus regardless of its strength. This corresponds to the period between the threshold being reached and when repolarisation is one-third completed. The relative refractory period starts at this point until the beginning of after-depolarisation. During this period, a stronger than normal stimulus may lead to excitation.
Synaptic transmission
Nerve impulses are transmitted from one neurone to another through junctions known as synapses. Synapses are formed between the terminal buttons of a neurone and the cell body or axon of another neurone. The number of terminal buttons forming synapses with a neurone varies from one to several thousand. Synapses almost invariably allow unidirectional impulse conduction, i.e. from the presynaptic to the postsynaptic neurone. This ensures nerve impulses are transmitted in an orderly fashion. Most synaptic transmissions are chemical in nature; others are either electrical or mixed. In electrical synapses, the membranes between the presynaptic and postsynaptic neurones meet to form gap junctions, which contain channels that facilitate diffusion of ions.
Structure of a synapse
Chemical synapses consist of a small gap known as a synaptic cleft. The synaptic cleft measures about 20 nm in width and contains extracellular fluid across which the neurotransmitters diffuse. There are three essential structures that can be found in the cytoplasm of the terminal button: synaptic vesicles, mitochondria and endoplasmic reticulum (Figure 18.6). The synaptic vesicles, containing the neurotransmitters, are usually found in large concentrations in the release zone adjacent to the synaptic cleft. The endoplasmic reticulum is responsible for the production of new vesicles, and recycling the used ones. Mitochondria provide the energy required for chemical transmission and the formation of synaptic vesicles by the endoplasmic reticulum.
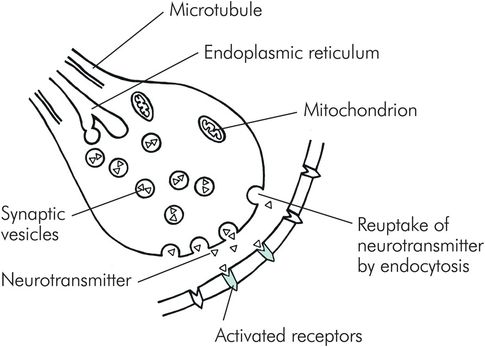
Structure of a chemical synapse
Synaptic mechanism
When an action potential is transmitted down an axon, the depolarisation opens the voltage-gated calcium channels, allowing an influx of Ca2+ ions into the terminal button. In the release zone the Ca2+ ions bind with groups of protein molecules in synaptic vesicle membranes. These protein molecules spread apart, allowing fusion between vesicle and terminal button membranes. This releases neurotransmitter into the synaptic cleft. The amount of neurotransmitter released is directly proportional to the Ca2+ influx. The postsynaptic receptors are activated by the binding of neurotransmitters, which leads to the opening of ion channels, resulting in postsynaptic potentials.
Postsynaptic potentials are transient in action because of three mechanisms. The first is transmitter reuptake; this is the predominant mechanism, and is performed by the presynaptic terminal buttons, which rapidly and actively take up neurotransmitters at the end of transmission. The second mechanism is enzymatic deactivation by enzymes in the synaptic cleft. Finally autoreceptors on the presynaptic membrane inhibit the continued release of neurotransmitter. Figure 18.7 summarises the different types of neurotransmitter found in the central nervous system (CNS).
Neurotransmitter | Location | Function |
---|---|---|
Acetylcholine | Cerebral cortex, thalamus, limbic system | Likely to be involved in memory, perception, cognition, attention and arousal functions |
Noradrenaline | Locus coeruleus | Descending pain pathway |
Cerebellum | Inhibits Purkinje cells | |
Hypothalamus | Regulates secretion of anterior pituitary hormones | |
Adrenaline | Medulla | Functions uncertain |
Dopamine | Substantia nigra | Control of motor functions |
Hypothalamus | Regulates prolactin secretion | |
Serotonin | Neocortex and limbic system | Alters mood and behaviour |
Hypothalamus | Increases prolactin secretion | |
Nucleus raphe magnus and spinal cord | Pain modulation |
The events following the generation of postsynaptic potentials depend mainly on two conditions: first, the amount of neurotransmitter released, and, second, the type of ion channel that is being opened. Not all postsynaptic potential changes are propagated as action potentials in the postsynaptic neurone. When an insufficient amount of neurotransmitter becomes bound to the postsynaptic receptor, the change in membrane potential may not reach the firing threshold of the neurone, thus giving rise only to local potential changes. When sodium channels are open, there is a sudden influx of Na+ ions down its concentration and electrical gradients, producing an excitatory postsynaptic potential (EPSP). However, when K+ or Cl– channels are open, K+ and Cl– ions move down their concentration gradients, making the inside of the neurone more electronegative with respect to the outside of the neurone, i.e. hyperpolarised, resulting in an inhibitory postsynaptic potential (IPSP). When the postsynaptic potential reaches threshold an action potential occurs.
Synapses
Synapses are junctions between the terminal buttons of one neurone and the body or axon of another.
A neurone may have between one and several thousand synapses contacting it.
Transmission across the synapse is unidirectional and is usually chemical but may be electrical.
Sensory receptors
Sensory receptors are specialised structures that receive and transmit information from the external and internal environment to the CNS. A sensory receptor may be part of a neurone, such as nerve endings, or a separate structure that is capable of generating and transmitting action potentials to a neurone. They are essentially transducers that respond to different forms of energy, such as mechanical or thermal energy, and convert them into electrical signals. Special sense organs such as the eye are a collection of sensory receptors supported by highly organised structural and connective tissue.
Sensory receptors may be classified according to whether they perceive visceral or somatic sensory changes:
Visceral receptors are primarily concerned with perceiving changes in the internal environment; such information does not usually reach consciousness. These include chemoreceptors which are sensitive to changes in glucose level, oxygen tension, osmolality and acidity in the plasma. Stretch receptors in the lungs and pressure receptors in the carotid sinus are other examples of visceral receptors.
Somatic receptors are sensory receptors that respond to external stimuli such as temperature, light touch, and pressure. Pain is initiated by noxious or potentially damaging stimuli; pain receptors are, therefore, also known as nociceptors. Information from the somatic receptors usually reaches consciousness and is represented at the cerebral level, giving rise to a variety of sensations. Sensory pathways are multisynaptic and thus involve a first-order neurone such as a dorsal root ganglion (DRG) which then synapses with a central chain of second-order and third-order neurones, as outlined in Figure 18.8.
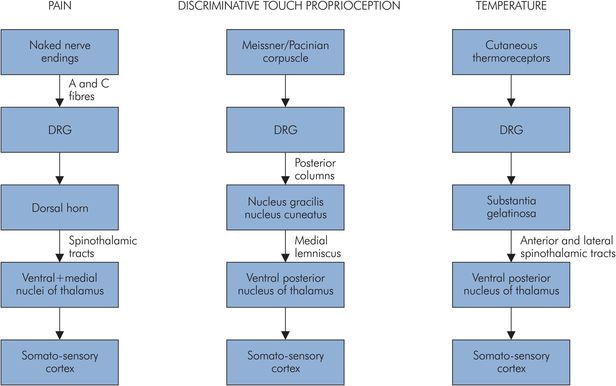
Somatic sensory perceptions and their afferent pathways to the CNS
Organisation of the nervous system
The nervous system is divided into two main parts: the central nervous system, consisting of the brain (cerebral cortex, basal ganglia, cerebellum, brainstem) and the spinal cord (Figure 18.9), and the peripheral nervous system, which includes the cranial and spinal nerves and their ganglia. The autonomic nervous system also forms an important part of the nervous system, and can be subdivided into the sympathetic and parasympathetic nervous systems.
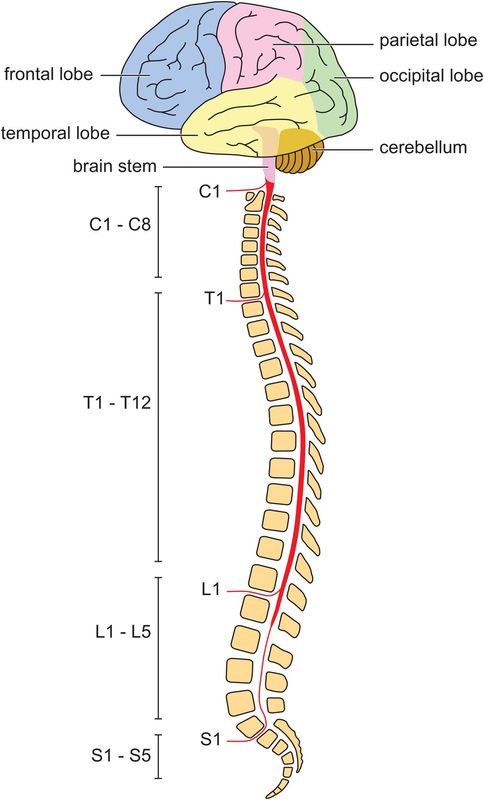
Gross anatomy of the central nervous system
Coverings of the central nervous system
A fibrous membrane, comprising three layers known collectively as the meninges, surrounds the entire CNS. Outermost is the dura mater, which covers the brain and is composed of two layers – an inner or meningeal layer of cerebral dura and an outer endosteal layer, which at the foramen magnum merges with the periosteum of the skull. The cerebral dura is represented in the vertebral canal by its periosteum, while the inner layer continues down to cover the spinal cord. The dural sac usually ends at the level of the second sacral vertebra in adults. Dura covering the spinal cord is attached to the edges of the vertebral canal except posteriorly, where it is completely free. The middle meningeal layer is called the arachnoid mater and closely lines the dural sheath. The innermost layer is known as the pia mater and is in contact with the neural tissues of the brain and the spinal cord. The trabeculated space between the arachnoid and pia mater contains cerebrospinal fluid (CSF).
Cortex
The cerebral cortex is topographically the highest level of the central nervous system, and always functions in association with the lower centres. The surface of the cerebral cortex is thrown into convolutions called gyri, which are separated by the sulci.
The cortex is divided into various lobes, namely frontal, temporal, parietal and occipital, which have different functions.
The frontal lobe lies in front of the central sulcus and is divided into the precentral area and prefrontal cortex. The precentral area is further divided into anterior and posterior regions. The posterior region is referred to as the primary motor area or Brodmann’s area 4, and the anterior region is known as the premotor area. The supplemental motor area is also situated in the frontal lobe. The rest of the frontal lobe, the prefrontal cortex, is divided into superior, middle and inferior frontal gyri. Broca’s speech area is situated in the inferior frontal gyrus of the dominant hemisphere. The prefrontal cortex is concerned with personality, initiative and judgement. The frontal cortex controls motor function of the opposite side of the body, insight and control of emotions.
The temporal lobe contains primary and secondary auditory areas. The ability to locate the source of sound is impaired with the destruction of the primary auditory area. Bilateral destruction leads to complete deafness. The secondary auditory area is necessary for the interpretation of sounds. Wernicke’s area, which is the sensory speech area, is located in the categorical (dominant) hemisphere and is associated with comprehension of speech.
The parietal lobe houses the primary sensory area in the postcentral gyrus. The majority of sensations reach the cortex from the contralateral side of the body, although some signals from the oral region go to the same side, and those from the pharynx, larynx, and perineum go to both sides. A secondary sensory area is situated in the wall of the Sylvian fissure. Loss of the primary sensory cortex leads to inability to judge shape or form (astereognosis), degree of pressure or weight of an object. Problems occur with position sense and in localising different sensations occurring in different parts of the body. The secondary sensory area is involved in receiving information and relating it to past experience so that the information can be interpreted.
The occipital lobe contains the primary visual area (area 17) close to the occipital pole. The macula lutea of the retina is represented on the cortex in the posterior part of area 17 and accounts for a third of the visual cortex. Lesions of the occipital pole produce central scotomas.
Higher functions of the cerebral cortex
Consciousness
There are different levels of consciousness, ranging from alertness to coma. The level of consciousness is determined by activities of both the cerebral cortex and the reticular activating system (RAS). The latter is a diffuse network of neurones situated in the brainstem reticular formation. It receives sensory information from ascending sensory tracts as well as auditory, visual, olfactory and trigeminal tracts. The reticular formation projects to the cerebral cortex directly and indirectly via the thalamic nuclei. RAS activity is closely related to the electrical activity of the cerebral cortex.
Electrical activity of the cerebral cortex can be recorded by scalp electrodes as an electroencephalogram (EEG). Various EEG rhythms can be identified (Figure 18.10).
α rhythm | Seen in an adult human at rest with eyes closed. It is more common in parieto-occipital areas and has a frequency of 8–12 Hz with an amplitude of 50–100 mV. |
β rhythm | Lower-amplitude wave with a frequency of 18–30 Hz. It is seen over the frontal region in a normal alert adult. |
θ rhythm | Large amplitude with a frequency of 4–7 Hz. It is normally seen in the very old and very young. |
δ rhythm | Consists of large slow waves with a frequency of less than 4 Hz. |
Two basic sleep patterns are seen: rapid eye movement sleep (REM) and non-rapid eye movement sleep (NREM). NREM has four stages. From stage 1 to 4 there is progressive slowing of the EEG with associated increase in EEG amplitude. Stage 1 sleep occurs at the beginning of sleep, while stage 4 sleep represents deep sleep. In stage 2 bursts of α-like rhythm known as sleep spindles are seen. In REM sleep the NREM pattern of EEG is replaced by fast low-voltage electrical activity similar to that seen in alert individuals. However, sleep is not disturbed and in fact the threshold for arousal is increased. This kind of sleep is associated with rapid eye movements, hence the name. In REM sleep, large phasic potentials are seen, there is a decrease in muscle tone, and it is also associated with dreaming.
Language
Wernicke’s area in the categorical hemisphere receives auditory and visual information and is concerned with comprehension of this information. It communicates via the arcuate fasciculus with Broca’s area, which is concerned with output of speech. It processes the information from Wernicke’s area to produce appropriate movements of the vocal apparatus.
Memory
Memory is the process of retention and storage of acquired information, and can be divided into explicit and implicit memory.
Explicit memory involves hippocampus and medial temporal lobes of the brain. It requires conscious retrieval or awareness and can be further divided into episodic memory (memory of events) and semantic memory, which is memory of words, rules, language and the world around us.
Implicit memory does not require conscious awareness and is not processed in the hippocampus. It is associated with skills required for day-to-day activities and habits. When learning certain tasks, such as driving or cycling, explicit memory is used, but once the tasks are well learned then implicit memory is used to perform them.
Both forms of memory can also be divided into short-term and long-term memory. Information is processed in the hippocampus as a short-term memory, and then stored as a long-term memory, which lasts for years. Short-term memory lasts from a few seconds to hours, and is easily affected by drugs and trauma.
It is thought that short-term memory is a transient store of limited capacity which permits instantaneous encoding and retrieval, but when the material is no longer the focus of conscious attention, only some of this material may pass on to long-term memory. The latter represents a long-term storage of indefinite capacity that requires effortful encoding and retrieval. The more elaborate and effortful the encoding process, the better the memory of the material.
At the cellular level, it is thought that information is stored in the short-term memory as reverberating electrical activity in the brain, whereas long-term memory is stored in a more robust form. It is thought that memory formation may lead to an alteration in the transmission of electrical signals through parts of the brain. However, it is not clear whether this is the result of facilitation of existing synapses or due to the formation of new synapses. In both situations, however, protein synthesis is thought to be ultimately involved in the formation of long-term memory, and this is brought about through either structural or enzymatic changes in the neurones. The hippocampus is primarily involved in memory storage, since pathological lesions in this area result in both anterograde and retrograde amnesia.
Higher cerebral function
Conscious level depends on the activity levels of the reticular activating system (RAS) and the cortex.
Sleep may either be associated with dreaming and rapid eye movement (REM), or non-rapid eye movement (NREM) sleep.
Memory is associated with the hippocampus and temporal lobes. It may be explicit (conscious) or implicit (unconscious).
Basal ganglia
The basal ganglia consist of interconnected deep nuclei including the caudate, globus pallidum, substantia nigra and putamen. The basal ganglia are involved in control of posture and movement.
Cerebellum
The cerebellum consists of two cerebellar hemispheres and a central structure. In contrast to the cerebral hemispheres, the cerebellar hemispheres control the structures on the same side of the body. The central cerebral structures control gait and maintain balance, including when seated.
Brainstem
Included in the brainstem are the midbrain, pons and medulla. The brainstem contains the reticular formation, which maintains consciousness, and the nuclei of all the cranial nerves except I and II. It also has ascending and descending tracts from cerebral structures and spinal cord. The corticospinal tract (one of the main descending tracts) and the dorsal columns, which are the ascending tracts, cross over in the medulla. Thus a lesion in the brainstem can produce cranial nerve lesions on the same side but limb signs on the other side.
The brainstem contains control centres for respiration, cardiovascular homeostasis, gastrointestinal function, balance, equilibrium and eye movements. Irreversible brainstem lesions are therefore frequently incompatible with life without artificial support.
Cerebral circulation
The arterial blood supply to the brain is derived from the right and left internal carotid arteries (ICAs) and the vertebrobasilar system (Figure 18.11). Each ICA gives rise to a posterior communicating artery (PCoA) before dividing into an anterior cerebral artery (ACA) and middle cerebral artery (MCA). The basilar artery (formed by the fusion of the vertebral arteries) lies on the ventral surface of the brainstem before dividing to form the two posterior cerebral arteries (PCAs). The anastomoses between the internal carotid and vertebrobasilar systems form the circle of Willis.
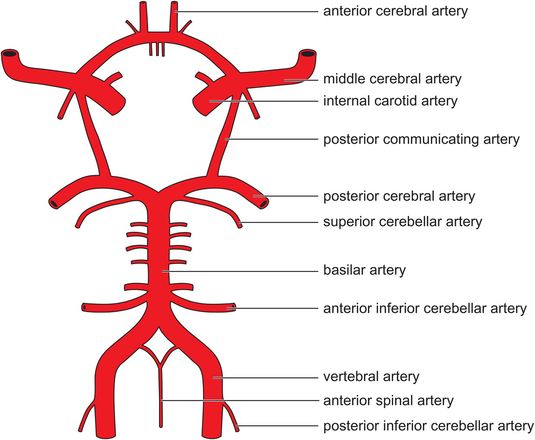
Arterial blood supply to the brain
Venous drainage occurs through a series of sinuses which lie between folds of dura mater and empty into the internal jugular vein.
Regulation of cerebral blood flow
The brain has the highest metabolic requirement of any organ, accounting for 20% of basal oxygen consumption (50 mL min–1) and 25% of total body glucose consumption. Cerebral blood flow (CBF) is approximately 700 mL min–1 (14% of cardiac output) in the resting adult. While this equates to a mean blood flow of approximately 50 mL per 100 g tissue per minute, flow is variable, with grey matter (110 mL 100 g–1 tissue min–1) receiving on average five times that of white matter (22 mL 100 g–1 tissue min–1). The pressure required to maintain CBF is the cerebral perfusion pressure (CPP).
CBF is usually tightly regulated to ensure constant delivery of glucose and oxygen to the brain. Four major factors control CBF:
Pressure autoregulation
Flow–metabolism coupling
PaO2
PaCO2
Pressure autoregulation
Autoregulation is the process by which the cerebral circulation maintains a constant blood flow despite alterations in CPP.
When cerebral perfusion pressure (CPP) varies, autoregulation maintains cerebral blood flow (CBF) by changes in cerebrovascular resistance (CVR). This is mediated by the myogenic response to changes in intramural pressure, since:
As shown in Figure 18.12, as MAP (or CPP) increases the cerebral vasculature constricts to maintain CBF. Conversely as MAP (or CPP) decreases cerebral vasodilatation occurs and CBF is maintained. Cerebral blood volume increases but vascular resistance decreases with vasodilatation. Classically, CBF remains relatively constant in healthy adults between CPP values of 50 and 150 mmHg. Chronic hypertension shifts the autoregulatory curve to the right. The presence of intracranial pathology and a number of drugs utilised in anaesthetic practice may impair autoregulation. For example, volatile anaesthetic agents cause dose-dependent vasodilatation of the cerebral vasculature and hence impair autoregulation. Hypocapnia is thought to augment autoregulation by increasing vascular tone.
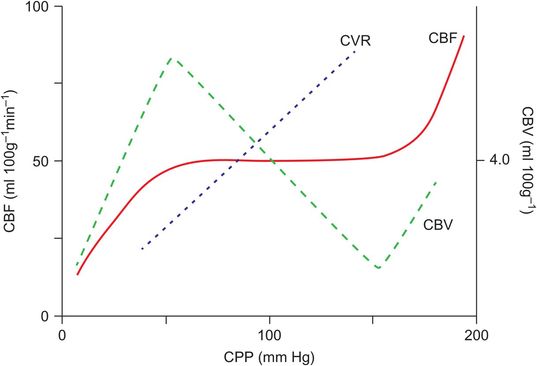
Relationship between cerebral blood flow (CBF), cerebral blood volume (CBV) and cerebrovascular resistance (CVR) over a range of perfusion pressures
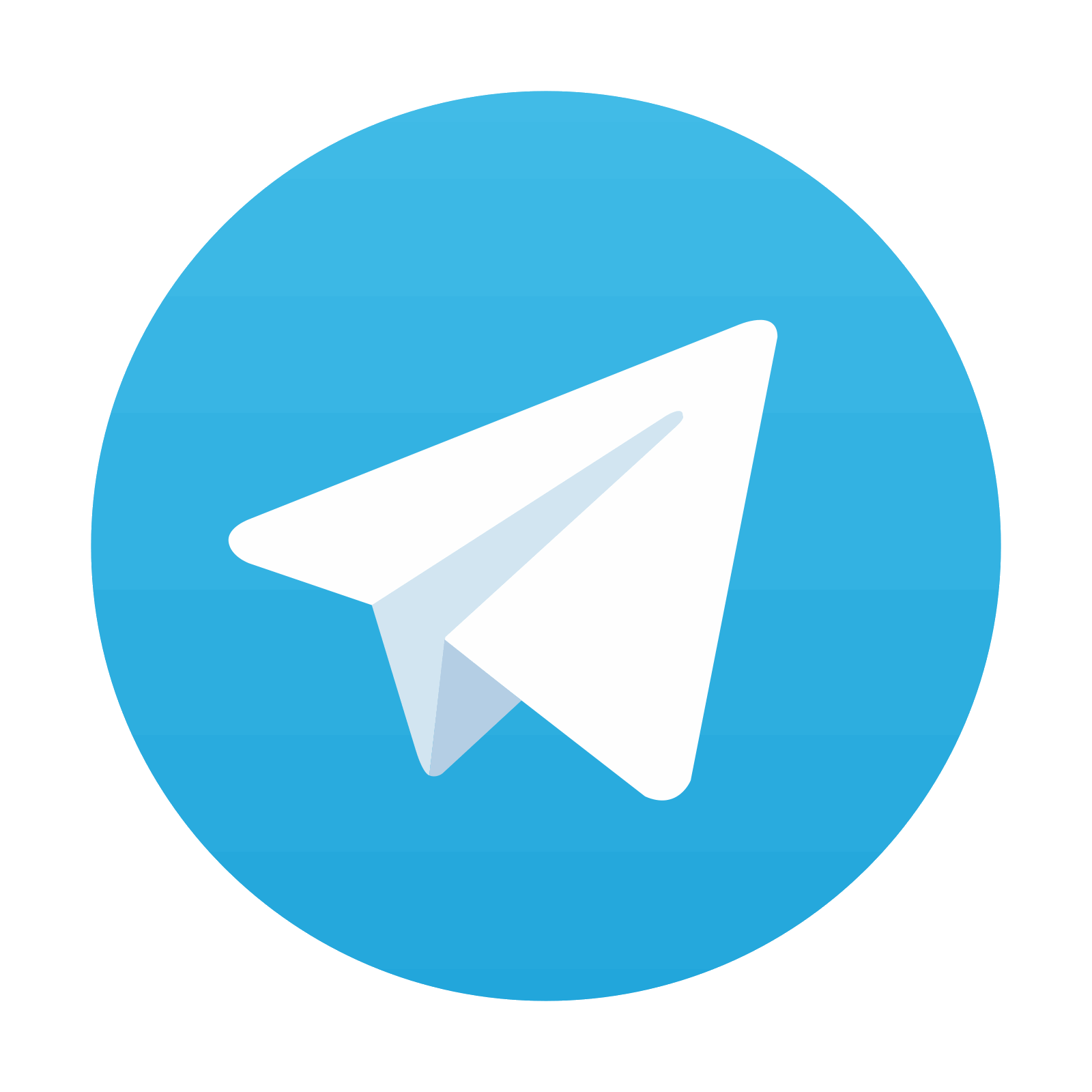
Stay updated, free articles. Join our Telegram channel
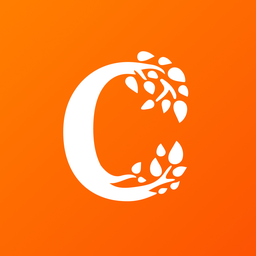
Full access? Get Clinical Tree
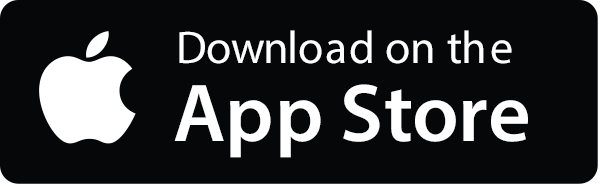
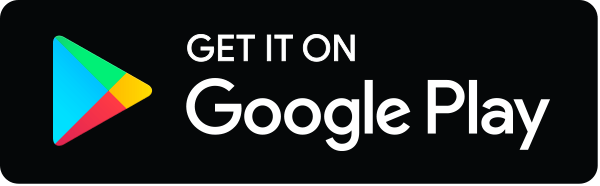
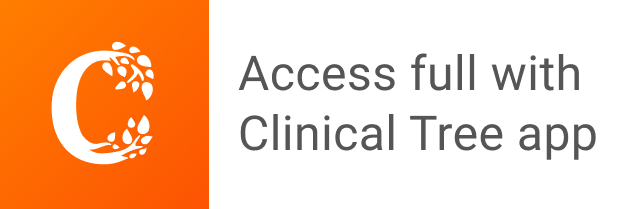