Edmond Cohen Thoracic procedures are performed with the patient in the lateral decubitus position. The distribution of ventilation and perfusion and the degree of venous admixture of shunt (Qs/Qt) is unique during the lateral position. The physiologic changes during the paralysis and opening the chest cavity must understood in order to manage the patient during the thoracic procedures. Factors that influence the transpulmoanry shunt and the effect of hypoxic pulmonary vasoconstriction is discussed in this chapters. One lung ventilation; ventilation/perfusion distribution; trans pulmonaryshunt during the upright and the; lateral position Thoracic procedures are usually performed with the patient in the lateral decubitus position. To understand the distribution of ventilation and perfusion in the lateral decubitus position and the degree of venous admixture of shunt (Qs/Qt) is expressed as a percentage. Alveolar ventilation/perfusion ratio distributions are plots of the total amounts of ventilation and total amounts of perfusion that are supplying each collection of lung units operating at a specific VA/Q ratio.1,2 This chapter will discuss the distribution of perfusion and ventilation in the upright and in the lateral position and the ventilation-perfusion ratio and will focus on issues relevant to thoracic surgery and one-lung ventilation (OLV). The information regarding the lung physiology can be found in Chapters 5 and 7, while a detailed discussion of the hypoxic pulmonary vasoconstriction (HPV) is available in Chapter 15. There are several reasons for hypoxemia: The causes of hypoventilation are depicted in Fig. 6.1. Following shunt, hypoventilation is the second cause of hypoxemia. One of the determinants of alveolar partial pressure of oxygen (PaO2) is the balance or ratio between ventilation and blood flow. In the patient with hypoventilation and normal blood flow results in a less oxygen delivery and unchanged removal of O2, which lowers PaO2. In an awake patient breathing room air, hypoventilation may result in severe hypoxemia because arterial partial pressure of carbon dioxide (PaCO2) increases to dangerously high levels. Whereas during anesthesia, providing supplemental O2 above 21%, will increase the PAO2 and arterial oxygenation will be maintained. The oxygen cascade is illustrated in Fig. 6.2. There are several factors that are essential for adequate gas exchange that must be considered as a cause of hypoxemia: diffusion block, shunt fraction, HPV and V/Q matching. The shunt equation quantifies the amount of blood in the three-compartment model that reaches the left heart without exchanging any gases. The equation quantitates venous admixture as a fraction of total lung blood flow. Arterial oxygen content (CAO2) and mixed venous oxygen content Cv-O2 are sampled from arterial and mixed venous blood from the pulmonary artery (through a pulmonary artery [PA] catheter), respectively. The CaO2 is assumed that the capillary saturation is 100% (equals that of ideal alveolar gas equation). Of interest, the shunt is essentially a ratio between the blood flow of a ventilated alveolar and the nonventilated alveoli. The equation for dead space displays a simple concept of a ratio. The shunt equation refers to oxygen in the capillary blood whereas the dead space equation refers to CO2 in the aerated alveoli. The reader should be familiar with the physiology of the upright position (Fig. 6.5). The blood flow is directly dependent upon the relationship between the alveolar (Pa) pressure, the pulmonary artery (Pa) pressure, and the pulmonary venous (Pv) pressure. There are several facts that should be highlighted: Because blood flow distribution to the lung is gravity-dependent and therefore is primarily directed to the dependent portion of the lung. The amount of blood flow depends on the pressure difference between the Pa and the Pv. There are several factors that influence the distribution of perfusion: It is important to highlight that these lung zones are physiologic, not anatomic. The boundaries between the zones are neither fixed nor anatomically defined. These boundaries can move downward with positive-pressure ventilation which increases Pa pressure or upward with exercise which increases Pa pressure. Changes in CO and lung volumes can have significant effects on the distribution of pulmonary blood flow either independently or by modulating the determinants of flow mentioned earlier. An increase in CO results in greater uniformity of blood flow throughout all of the lung fields. The mechanisms proposed were an attenuation of HPV because of an increase in pulmonary arterial pressure and a lessening of the gravitational gradient. Changes in lung volumes can alter the distribution of blood flow. The lung volume at which the pulmonary vascular resistance (PVR) is lowest is at FRC. When the lung volume is lower than FRC, the resistance of the pulmonary vascular system is increased because of decreased caliber of the extraalveolar vessels. This may be caused in part by the loss of radial traction supporting these vessels at low lung volumes. At lung volumes above FRC, PVR increases because of the stretch of the capillaries. Alveolar ventilation in the upright position gradually falls from the base to the apex of the lung because of posture and gravity. The lung is a visceral elastic organ and because of the lung’s weight, it tends to assume a bell shape in the chest cavity. Because the chest cavity is a closed space, the distance between the pleural lung and the parietal lung is greater at the apex of the lung. As a consequence, the intrapleural pressure (PIP) is more negative at the apex than at the base when the subject is upright. The intrinsic mechanical properties of the airways are the same, regardless of whether the tissue is at the base or at the apex. At the base, where PIP might be only −2.5 cm H2O at FRC, the alveoli are relatively underinflated compared with tissues at the apex, where PIP might be −10 cm H2O and the alveoli are relatively overinflated. However, because the base of the lung is underinflated at FRC, it is on a steeper part of the pressure-volume curve (Fig. 6.6). Ventilation is distributed mainly to the more compliant regions at the base of the lung. Because the alveoli at the apex of the lung in zone 1 are already distended by the negative intrapleural pressure at the apex, they are less compliant. In contrast, the alveoli at the base of the lung are less distended but more compliant. Therefore for the same increment in intraalveolar pressure, the alveoli at the base of the lung receive the major portion of the tidal volume. Because both perfusion and ventilation are primarily directed to the dependent portion of the lung, it would preserve the good ventilation/perfusion matching.9 V/Q matching is essential for normal gas exchange in the lungs. For normal gas exchange, alveoli must be in close proximity to pulmonary capillaries ventilation, which must be close to blood flow. The V/Q ratio expresses the matching of ventilation (V in L/min) to perfusion or blood flow (Q in L/min). It is useless if ventilated alveoli are not near perfused capillaries, or if perfused capillaries are not near ventilated alveoli. For the entire lung, the average normal value of V/Q is 0.8. This means for the whole lung, ventilation (L/min) is 80% of the lung perfusion (L/min). However, V/Q is not uniformly 0.8 throughout the entire normal lung; the regions above the level of the third ribs will have higher V/Q and some regions have lower V/Q. An average V/Q of 0.8 results in an arterial PaO2 of 100 mm Hg and arterial PaCO2 of 40 mm Hg, the normal values. A maldistribution of ventilation and perfusion causes a defect in gas exchange. Alveoli that are ventilated and not perfused are considered a “dead space” while the perfused capillaries that are not ventilated represent a “shunt.” A distribution of every possibility in between, high V/low Q = high V/Q and low V/high Q = low V/Q will be present, depending upon the lung zone. These regional variations in the upright position in both ventilation and blood flow are depended on the gravitational effects that cause blood flow to be highest at the base and lowest at the apex. The regional variations in ventilation occur in the same direction as those for blood flow; thus ventilation is highest at the base and lowest at the apex. However, and importantly, the variations in blood flow are greater than the variations for ventilation.9 The apex (zone 1) has the highest V/Q with the lowest blood flow, the lowest ventilation, but higher the ventilation relative to perfusion, the higher PaO2 and a lower PaCO2. The base (zone 3) has a lower V/Q, the highest blood flow, the highest ventilation, but lower the ventilation relative to perfusion, lower PaO2 and a higher PaCO2. It is the main site of gas exchange. In zone 2, the V/Q ratio is in between (Fig. 6.7). Fig. 6.8 shows a plot of the blood flow, ventilation, and V/Q ratio, the blood flow and ventilation lines cross at about the third rib in the diagram (ratio = 1). Below that rib the V/Q ratio is below 1.0 and rapidly increases above the third rib (>3).1,2,10,11 The distribution of blood flow in the lung as being gravity-depended, as described in the chapter, has been challenged by the model of fractal distribution of both perfusion and ventilation. In a human, approximately 5 L of blood per minute are distributed into a single red blood cell layer surrounding approximately 300 million alveoli. The highly complex structure of the pulmonary vascular network accomplishing this may easily be understood if the single vascular bifurcation is considered. The pulmonary vascular tree consists of a multitude of bifurcations, all having a similar structure but different sizes. This concept of self-similarity is also termed fractal (Latin frangere, to break; fractus, broken). It emphasizes the possibility that in contrast to objects in Euclidian geometry (lines, triangles), in which dimensions are integer numbers, natural objects may have fractional dimensions (e.g., 1.5 or 2.3).12,13 The pulmonary vasculature, by virtue of its tree-like structure, is a fractal object. At each bifurcation, a minimally uneven distribution of blood flow occurs. Heterogeneity is amplified by the many bifurcations in series. The function of the vascular tree is the distribution of blood to the pulmonary parenchyma. It is therefore logical to assume that the result of this distribution, perfusion heterogeneity, is also fractal. Therefore statistical measures of heterogeneity are also self-similar at different scales of magnification (i.e., fractal). Heterogeneity of pulmonary perfusion depends on the resolution of the measurement technique. Intuitively, this can be grasped by considering that low resolution hides more bifurcations (which are sources of heterogeneity) than high resolution. Efficient gas exchange is dependent on the close matching of regional ventilation and perfusion. Given the high degree of heterogeneity observed at small regional volumes in this study, ventilation and perfusion must be closely correlated to achieve this matching. In the normal lung, this must occur by one of the following three mechanisms: (1) active matching of regional perfusion to ventilation, (2) active matching of regional ventilation to perfusion, or (3) passive matching of ventilation and perfusion by innate pulmonary structure. In the normal lung, basal pulmonary vascular tone is minimal, suggesting that vasoregulation is of minor importance for maintaining close V/Q matching in uninjured lungs. Passive matching of ventilation and perfusion by pulmonary structure is appealing because it requires the least amount of energy. An optimally engineered system requires no active feedback mechanisms during normal function. Fractal distribution networks for ventilation and perfusion provide several inherent advantages. Fractal structures are the most efficient way to fill a three-dimensional structure and provide the most energy efficient substrate transport. Studies that evaluated the effect of gravity on blood flow used animal models that were exposed to three or more G forces (using centrifuge) while tracing the blood flow distribution with fluorescent microsphere. Chornuk et al.14 assessed the influence of cranial-to-caudal inertial force (+G) during positive pressure breathing during G (PBG) on regional pulmonary blood flow distribution. Unanesthetized swine were exposed randomly to a variety of G forces: from 0 G (resting), +3 G, +6 G, up to +9 G, with and without anti-G suit and PBG with the use of the Air Force Research Laboratory centrifuge. Fluorescent microspheres were injected into the pulmonary vasculature as a marker of regional pulmonary blood flow. Lungs were excised, dried, and diced into around 2-cm3 pieces, and the fluorescence of each piece was measured. As =G was increased from 0 to +3 G, blood flow shifted from cranial and hilar regions toward caudal and peripheral regions of the lung. This redistribution shifted back toward cranial and hilar regions as anti-G suit inflation pressure increased at +6 and +9 G. Perfusion heterogeneity increased with +G stress and decreased at the higher anti-G suit pressures. The distribution of pulmonary blood flow was not affected by PBG. Of interest, as depicted in Fig. 6.9, the blood flow distribution within the lung was similar when the animal was exposed to 1 G or 3 G. That lead to the conclusion that almost 60% of the blood flow distribution was caused by anatomic fractal structure of the lung rather than gravity depended. The anatomic structure is the major determinant of pulmonary blood flow. Analysis of the variability of blood flow within individual regions shows that more than 60% of flow variation is caused by pulmonary vascular structure. Inertial force and anti-G suit account for less than 20% of the flow variation. Altemeier et al.15 evaluated the fractal distribution of the ventilation. High-resolution measurements of pulmonary perfusion reveal substantial spatial heterogeneity that is fractally distributed and the vascular tree is the principal determinant of regional blood flow. Recent studies using aerosol deposition show similar ventilation heterogeneity that is closely correlated with perfusion. The authors hypothesized that ventilation has fractal characteristics similar to blood flow. They measured regional ventilation and perfusion with aerosolized and injected fluorescent microspheres in anesthetized, mechanically ventilated pigs in both prone and supine postures. Coefficients of variation were calculated for each cluster size to determine fractal dimensions. At the smallest size lung piece, local ventilation and perfusion are highly correlated, with no significant difference between ventilation and perfusion heterogeneity (Fig. 6.10). Ventilation has fractal properties similar to perfusion. Efficient gas exchange is preserved, despite ventilation and perfusion heterogeneity, through close correlation. They concluded that one potential explanation is the similar geometry of bronchial and vascular structures.
Physiology of the Lateral Position andOne-Lung Ventilation
Abstract
Keywords
Introduction
Hypoxemia
Ventilation Prefusion Upright Position
Distribution of Perfusion7,8
Other Factors That Influence the Distribution of Blood Flow
Distribution of Ventilation1,2,7,8
Ventilation-Perfusion Matching
Fractal Distribution of Perfusion and Ventilation
Fractal Distribution of Perfusion
Stay updated, free articles. Join our Telegram channel
Full access? Get Clinical Tree
Physiology of the Lateral Position andOne-Lung Ventilation
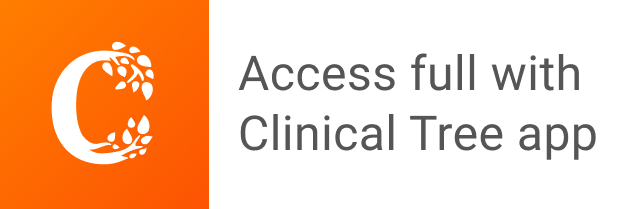