Physiology of One-Lung Ventilation
Raquel R. Bartz
Richard E. Moon
Optimal operating conditions for many cardiothoracic procedures require collapse of one lung, producing a challenge for the anesthesiologist who must maintain arterial PO2, PCO2, and hemodynamics within tolerable levels while ventilating the single remaining lung. One-lung ventilation (OLV) for thoracic surgery is usually performed while the patient is in the lateral decubitus position, with the nondependent lung collapsed. For other types of procedures, for example whole lung lavage, the patient may be in the supine position and the nonventilated lung remains inflated with saline resulting in different physiologic consequences. Therefore, a thorough understanding of pulmonary physiology will help facilitate the delivery of anesthesia for procedures requiring OLV.
PULMONARY VENTILATION
Flow of gas into the lungs is dependent upon the pressure difference between the upper airway (or endotracheal tube) and the alveoli. This pressure change can be induced either by inspiratory muscle contraction (spontaneous breathing) or positive pressure ventilation. Time-related variation in lung and chest wall mechanical properties induce variable tidal volumes and airway pressures during both spontaneous breathing and in some modes of positive pressure ventilation. To expand the lungs and overcome elastic recoil and resistive loads, a sufficient transpulmonary pressure gradient must either be generated with the use of positive pressure breathing or from negative pressure by the contraction of the diaphragm and muscles of inspiration as occurs during spontaneous breathing.
Elastic Effects
The lungs can be considered as many small, interconnected balloons inside the chest cavity, the elastic properties of which produce a recoil force. During lung expansion, recoil force of the lung is generated due to smooth muscle, elastin, and collagen as well as from other surface forces. The latter are due to surface tension generated by liquid within the alveoli. This is attenuated by surfactant produced by type II pneumocytes present at the alveolar-air interface. Changes in lung volume are accompanied by parallel changes in the volume of the chest wall, which has its own elastic properties. There is also a resistive component, mostly due to the properties of the airways, discussed below.
The lungs expand due to an increase in transpulmonary pressure (Ptp), equal to the difference between alveolar pressure (PA) and pleural pressure (Ppl) (Ptp = PA – Ppl). The pressure generated within the lungs to produce lung inflation must also be great enough to overcome forces independent of the lung including the chest wall, diaphragm, and abdomen (defined collectively as “chest wall”).
The change in a given unit volume (eg, lung volume) per unit change in pressure is termed compliance. Compliance (C) is calculated by the following equation:
Where V is intrapulmonary gas and P is transthoracic pressure. Lung compliance (CL) in a normal awake human is typically in the range 150 to 250 mL/cm H2O. Because elastic recoil forces increase at maximal lung volumes, compliance decreases as the lung inflates. Lung compliance is also decreased in diseases that result in scarring, fibrosis, and pulmonary edema. Although lung compliance normally increases with aging, diseases that disrupt the lung architecture of the alveolar septa, such as emphysema, will accelerate this process. Changes of lung compliance also occur with general anesthesia. Measurements before and after induction of anesthesia have typically revealed a 15% to 50% decrease in CL.1
Because transpulmonary pressure is difficult to measure, the most clinically relevant ΔP is transrespiratory pressure, PTR, defined as the pressure required to expand both lungs and the chest wall, which in a mechanically ventilated patient is equal to airway pressure. PTR can be used to calculate total respiratory system compliance (CRS), which is typically 90 to 120 mL/cm H2O. Compliances for individual hemithoraces in normal anesthetized volunteers in the lateral decubitus position have been reported as 39 mL/cm H2O (nondependent hemithorax) and 29 mL/cm H2O (dependent hemithorax).2
Several factors affect lung compliance including lung volume, patient position, pulmonary blood volume, age, restriction of chest wall expansion, smooth muscle tone, lung diseases, and general anesthesia. After induction of general anesthesia there is a 20% to 35% reduction in CRS. In common clinical usage, respiratory system compliance is defined as flow-dependent inflation (dynamic compliance) and non-flow-dependent inflation or static compliance. Dynamic and static compliance can be easily measured during positive pressure ventilation. Dynamic compliance represents the function of airway resistance on pressure changes whereas static compliance is measured when gas flow is static at the end of inspiration and thus mostly represents the compliance of the alveolar units. Changes in peak airway pressure measured by the mechanical ventilator at the end of inspiration reflect changes of dynamic compliance whereas changes of the plateau pressure (alveolar pressure) reflect changes in static compliance (Figure 3–1). Measurement of lung expansion and deflation, however, show that deflation pressures exceed inflation pressures. This difference in the volumes between inflation and deflation within the lung represents the affects of airway resistance and hysteresis, which is mostly due to the air–water surface forces at the beginning of inflation. The change in compliance during inflation and deflation can also be graphically plotted as pressure/volume loops, which many mechanical ventilators on anesthesia machines now display. The conventional clinical definition of dynamic compliance described above for mechanically ventilated patients differs from the classic definition, in which inspiratory and expiratory pressures are obtained at zero flow.
Figure 3–1. Measurement of respiratory system (thoracic compliance). The pressure-volume relationship (peak and plateau pressures, positive end-expiratory pressure (PEEP), and tidal volume, VT) during delivery of a positive pressure tidal volume can be used to calculate clinically defined static and dynamic respiratory system compliances (CDyn, CStat).
Resistive Effects
The airways of the respiratory system not only serve as the conducting vessels for gas transport but also contribute to the overall homeostasis of the lungs. In the noninstrumented patient, air is humidified in the upper airway and ciliated epithelial cells clear particles as they move down respiratory passages. Airway diameters, and thus resistance (R), change in response to sympathetic, cholinergic and nonadrenergic noncholinergic (NANC) systems, which act on bronchial smooth muscle and mucus glands. NANC mediators include substance P, neurokinins A and B (which cause bronchoconstriction) and vasoactive intestinal peptide (VIP, which causes bronchodilation). Bronchodilation also occurs as a result of local generation of nitric oxide (NO), which induces smooth muscle relaxation through soluble guanylate cyclase-dependent mechanisms. Like compliance, airway resistance changes with lung volume and differs during inspiration and expiration. During inspiration, lung expansion tends to increase airway diameter and thus decrease resistance; during expiration the decreasing lung volumes can result in compressed airways and increased airway resistance. Clinically, this may be an issue for patients with pathological narrowing of the airways, such as asthma, which may result in intrinsic positive end-expiratory pressure at the end of expiration or auto PEEP. Because inhaled anesthetics inhibit smooth muscle contraction of the airways, they produce a significant decrease in airway resistance and in the past have been used successfully to treat severe asthma exacerbations.
NORMAL LUNG VOLUMES AND EFFECTS OF ANESTHESIA, POSITIONING, AND POSITIVE PRESSURE VENTILATION
Lung volumes can be divided into different components (Figure 3–2). Normal, resting breathing occurs with a tidal volume (VT) of approximately 5 to 7 mL/kg of ideal body weight. The volume of gas that can be exhaled beyond a resting expiration is termed the expiratory reserve volume (ERV), which is approximately 1000 mL in males and 600 mL in females. A small amount of gas is always present within the lungs at the end of maximal expiration and is termed the residual volume (RV). The summation of RV and ERV make up the functional residual capacity (FRC), which represents the point where alveolar pressure equals ambient pressure and the expansive chest wall forces are balanced by the elastic recoil of the lungs. Several factors influence FRC. Stiffening of the chest wall from aging and a decrease in the elastic forces of the lung leads to a gradual increase in RV and FRC over time. FRC is linearly related to height and decreases significantly due to obesity. In women, FRC is about 10% lower than in men. FRC is also decreased by the supine position and is affected by general anesthesia. FRC decreases during general anesthesia by 10% to 20% primarily due to a change in the shape of the ribcage.3 In awake volunteers in the lateral decubitus position, the dependent lung has a lower FRC than the nondependent lung, which during anesthesia can lead to atelectasis and regions of poor ventilation. In studies in anesthetized volunteers after being turned from supine to lateral decubitus position, the normal reduction in FRC due to anesthesia was partially reversed, due to an increase in the nondependent lung FRC.2 In the supine position each lung contributes approximately the same proportion of ventilation. However, in the lateral decubitus position during spontaneous breathing ventilation of the dependent lung is greater than the nondependent lung,2,4 while during positive pressure ventilation the reverse is true.2
Figure 3–2. Subdivision of lung volumes. Several factors can different subdivisions of the lung volumes especially Functional Residual Capacity (FRC). FRC is linearly related to height, which increases FRC. Obesity and the supine position cause a reduction in FRC. (Adapted with permission from Shier D, Butler J, Lewis R. Hole’s Human Anatomy and Physiology. New York: McGraw-Hill; 2004).
VENTILATION AND PULMONARY GAS EXCHANGE
Ventilation exchanges air from the environment to the lungs by producing a transpulmonary pressure gradient that facilitates transport of oxygen from the upper airway to the alveoli and movement of carbon dioxide in the opposite direction. Several factors influence both the alveolar oxygen and carbon dioxide pressure. The main determinants of alveolar PO2 (PAO2) are inspired PO2 and alveolar ventilation . Arterial PO2 (PaO2) also depends on ventilation/perfusion matching, right-to-left (intrapulmonary and intracardiac) and alveolar-capillary diffusion, although the latter is rarely a limiting factor in clinical medicine except during exercise in patients with interstitial disease.
After leaving the right heart, deoxygenated blood enters the pulmonary capillaries and flows into the pulmonary veins. Hypoxemia results from desaturated blood (venous admixture) emanating from abnormal gas exchange units with low ventilation/perfusion ratio <1) or shunt units. Pulmonary arteriovenous right-to-left shunts have been described within the normal lung and are detectable when pulmonary artery flow and pressure are high, such as during exercise. Two other physiological sources of deoxygenated blood include the bronchial and thebesian veins, which drain directly into the pulmonary veins and left atrium, respectively (“left-to-left” or post-pulmonary shunt). Normally these account for <1% of the cardiac output (CO) and therefore do not significantly affect arterial oxygenation. However, in patients with severe bronchiectasis, where the bronchial circulation can increase several fold, left-to-left shunt may contribute significantly to arterial hypoxemia.
Secondary factors affecting PaO2 include CO and second gas effect. The alveolar/arterial PO2 difference in a young healthy adult breathing air is usually < 10 mm Hg but increases with age and lung disease. This difference results from physiological shunt or venous admixture. In diseased lungs this difference may increase significantly and is the main cause of arterial hypoxemia.
In the steady state, PCO2 in arterial blood is determined by the amount of CO2 produced from metabolism and the amount eliminated by ventilation. Total ventilation (minute ventilation, [], which is the product of respiratory rate [f] and tidal volume [VT]), is made up of alveolar ventilation and dead space ventilation (
). Dead space is composed of the volume of the upper airway and conducting airways (“anatomic” or “series” dead space) and unperfused or hypoperfused distal gas exchange units (physiological or parallel dead space). Alveolar PCO2 is determined by the following equation:
Alveolar ventilation can be written as:
Where VD/VT is dead space/tidal volume ratio. Therefore, since is a function of both
and VD/VT, any variation in
, or VD/VT will also affect PCO2. O2 consumption
, and hence
, decreases slightly during general anesthesia, to a large extent because of the frequently associated reduction in body temperature (Figure 3–3).
Figure 3–3. Alveolar ventilation is determined by the amount of anatomic and physiologic dead space as well as the minute ventilation, which is a function of respiratory rate and tidal volume.
During OLV, maintenance of PaCO2 at preinduction levels will require at or slightly higher than during two-lung ventilation. This is to account for the effect of right-to-left shunt and less efficient
matching. Indeed, the majority of individuals requiring OLV have lung disease, which may limit the degree to which isocapnia can be maintained. In particular, obstructive lung disease is associated with increased VD/VT. Airways obstruction may limit the adequacy of expiration and lead to dynamic hyperinflation also known as auto PEEP, particularly during OLV5, which in turn can reduce pulmonary blood flow and lead to systemic hypotension.
PULMONARY CIRCULATION
The pulmonary arteries divide several times to form arterioles that have larger diameters and thinner vessel walls than their counterparts in the systemic circulation. This interconnected network of vessels leads to small single-cell layered capillaries where alveolar-capillary exchange of O2 and CO2 occurs via diffusion. While the majority of pulmonary gas exchange occurs in the capillaries, a significant portion also occurs in the larger arterioles of the pulmonary vasculature. During air breathing, pulmonary blood may acquire as much as 15% of its O2 load before reaching the capillaries; during 100% oxygen breathing the pulmonary arterial blood may be fully oxygenated before traversing the capillaries.6
Because the pulmonary circulation is a large and highly compliant interconnected network of vasculature under relatively low pressure and normal physiologic conditions, the right ventricle requires less contractile force to generate blood flow into the capillary network of the lungs than the force generated by left ventricle for the systemic circulation. For this reason, the right ventricle has less musculature than its counterpart on the left side. Adequate ejection of blood from the right ventricle and the distensibility or resistance of the pulmonary vasculature are the main determinants of pulmonary arterial blood flow.
Positional changes in pulmonary blood flow distribution would be expected when the patient is turned to the lateral decubitus position. While animal studies suggest in fact that very little flow redistribution occurs; human data support increased blood flow in the dependent lung.4,7,8 This facilitates matching and maintenance of arterial oxygenation during OLV in the lateral decubitus position.
PULMONARY CIRCULATION PRESSURE-FLOW RELATIONSHIPS
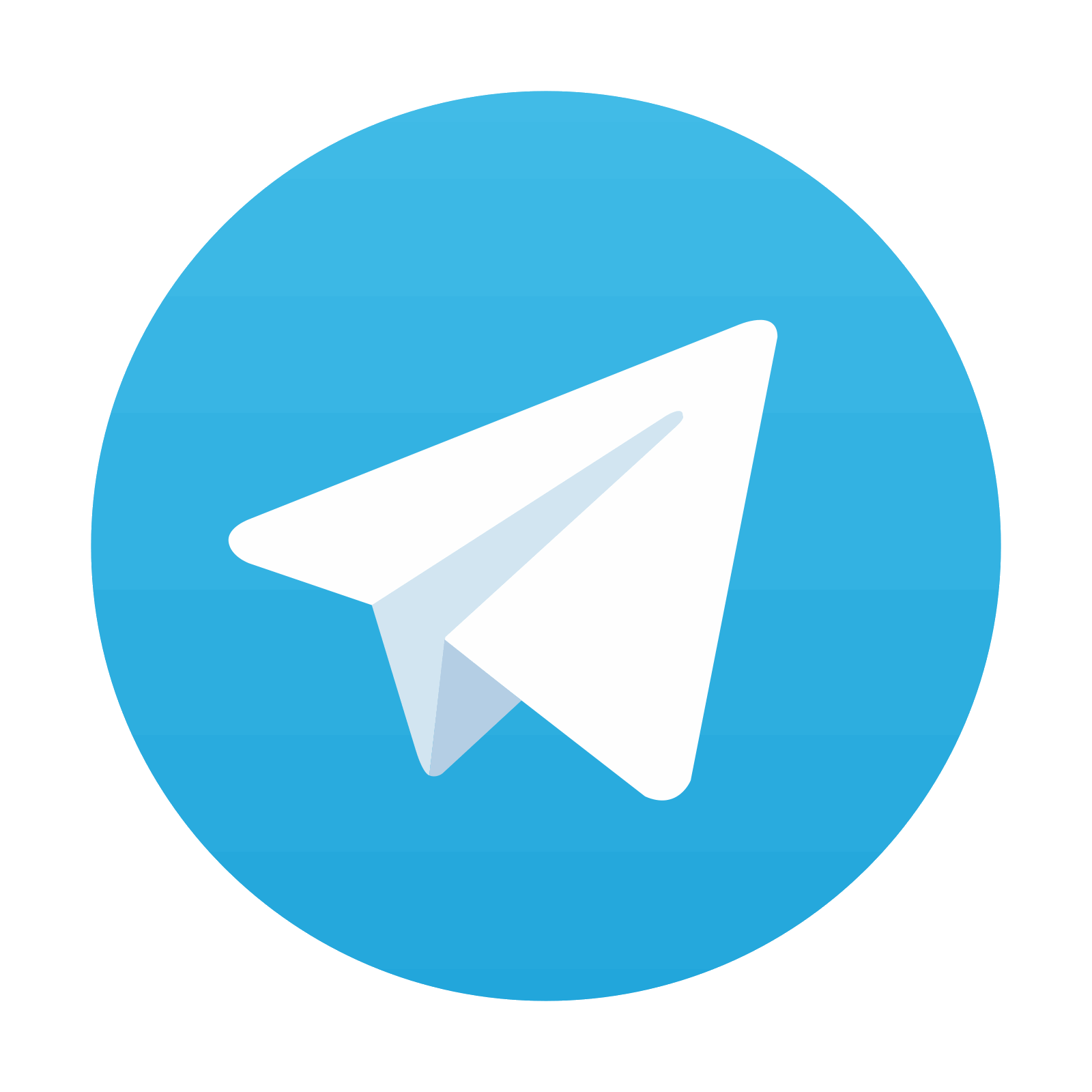
Stay updated, free articles. Join our Telegram channel
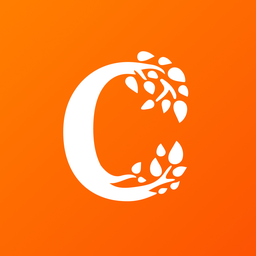
Full access? Get Clinical Tree
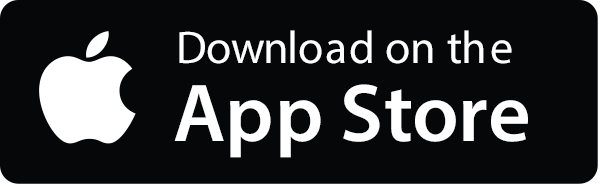
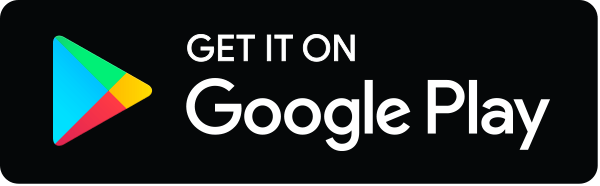