o2 = CO × CaO2
where O2 is equal to oxygen delivery, CO represents cardiac output, and CaO2 is the O2 content of arterial blood.
In a 70-kg person, oxygen delivery is assumed to be 1 L per minute, derived by multiplying cardiac output (5 L per minute) times arterial oxygen content (200 mL O2/L). Arterial oxygen content can be calculated by multiplying 15 g hemoglobin/dL (or 150g/L) times 1.39 (the amount of oxygen each gram of hemoglobin can hold when fully saturated) times the arterial oxygen saturation. For this calculation, we are assuming a hemoglobin oxygen saturation of 100%.
From these equations, one can gain insight into the three mechanisms from which death might occur from cardiac and brain ischemia if the patient is not resuscitated in a timely and effective fashion. (a) During ventricular tachycardia, ventricular fibrillation, or during asystole, cardiac output decreases to zero as will oxygen delivery to the tissues. (b) During hemorrhagic shock, as hemoglobin levels decrease, the arterial oxygen content decreases and cardiac output falls because of decreased intravascular volume resulting in decreased left ventricular end diastolic volume. (c) During apnea from whatever cause, as whatever oxygen is contained in the functional residual capacity of the lung decreases, the amount of oxygen available to bind with hemoglobin decreases, which also results in a steadily decreasing oxygen delivery and eventually, cell death.
For the purposes of this chapter then, the focus will be on resuscitation from cardiac events, traumatic injury, and decreased fractional inspired concentration of oxygen (FIO2).
Pathophysiology
The basic physiology of death due to decreased oxygen delivery is in some ways straightforward but at the same time, quite complex. As oxygenated hemoglobin is delivered to peripheral tissues, O2 dissociates from hemoglobin because of the concentration gradient between the oxygenated hemoglobin in red blood cells and the capillary blood perfusing the tissue. The dissociation of O2 from hemoglobin is facilitated by the respiratory acidosis created by CO2 released through cellular respiration. Oxygen diffuses out of red blood cells through the plasma, across cell membranes, and into the cytoplasm where it is taken up by mitochondria. In oxidative metabolism, O2 breaks down pyruvate, derived from glucose, amino acids, and fatty acids in the Krebs cycle. Water and CO2 are the byproducts and the energy produced is used by the coenzymes nicotinamide, adenine, dinucleotide, and flavin adenine dinucleotide to add a phosphate molecule to adenosine diphosphate to create adenosine triphosphate (ATP). The third phosphate bond is readily broken and the energy that is released is used by cells for protein synthesis, for endocytosis and exocytosis, to provide energy for other transporter processes, for maintenance of the electrical potential across the membrane, and to maintain the integrity of the cell membrane itself. Oxygen is the molecule that serves as the electron acceptor by which nicotinamide adenine dinucleotide and flavin adenine dinucleotide produce more ATP than can be created by anaerobic metabolism. Based strictly on the byproducts of the chemical reactions involved, anaerobic metabolism of pyruvate leads to the production of 4 molecules of ATP, whereas the metabolism of pyruvate in the presence of O2 can potentially produce 38 molecules of ATP. Anaerobic metabolism is insufficient in the long term to produce enough ATP molecules to maintain cell integrity. In the absence of oxygen, cells die (Fig. 47-1) at a variable rate depending on their metabolic rate. Typically, neurons are the most sensitive to lack of O2 and will develop irreversible damage within 3 to 5 minutes; myocytes and hepatocytes on the other hand can survive 1 to 2 hours in the absence of O2; muscle cells may survive for several hours. By decreasing metabolic rate, hypothermia can prolong the “safe” ischemic time. Neurons for example decrease their metabolic rate by approximately 7% for every 1°C decrease in temperature.4 There is a limit, however, to how much hypothermia can delay a cell’s demise. For example, during circulatory arrest while a patient is on cardiopulmonary bypass, at 15°C to 20°C, neurons do not display irreversible cell damage for as long as 30 to 60 minutes, but after 60 minutes, the risk for potential irreversible neuronal damage increases markedly. Hypothermia has the same effect on other cells such as myocytes and hepatocytes, as well as cells in other organs. This factor allows transplant surgeons to prolong the amount of time between when an organ is harvested and when it is transplanted, thus improving the matching potential for transplantable organs.

Although temperature has an impact on survivability of ischemic cells, time is the factor that most directly and importantly impacts the reversibility of ischemia—the safe ischemic time. Independent of all other factors, the number of minutes that cardiac function is arrested, the amount of time that the hemoglobin concentration is below 6 to 7 g/dL, or the duration of apnea or asphyxia all directly correlate with morbidity and mortality. For cardiac arrest, evidence from assessment of the effect of duration of the arrest on survivability suggests that mortality increases by 8% to 10% for each minute for which there is no cardiac output.5 From a physiologic perspective, the most vital factor then in determining outcome is the timely restoration of normal cardiac, pulmonary, and hemostatic function. Drugs play a limited, but very important, role in the return of the aforementioned functions in certain circumstances as will be described later.
Cardiac Arrest
The National Library of Medicine in its MeSH (Medical Subject Headings) terms defines “heart arrest” as the cessation of effective cardiac function, which if not recognized and treated within minutes will lead to “sudden cardiac death.” Sudden cardiac death in turn is the unexpected rapid natural death due to cardiovascular collapse within 1 hour of the onset of symptoms usually caused by the worsening of existing heart disease.
Ventricular tachyarrhythmias account for many cardiac arrests; 6% to 14% of the time, no known structural lesions of the heart can be identified.6 If no structural cause can be identified, individuals who have a prolonged QT interval are at increased risk of developing polymorphic ventricular tachycardia, that is, torsades de pointes especially if they receive certain drugs that are also known to prolong the QT. Mutations in genes that code for ion channels that generate the cardiac action potential have been identified in patients with the long QT syndrome.7,8 There are more than 150 drugs that have been reported to prolong the QT interval; list the drugs that are most relevant to anesthesiologists. Drugs that prolong the QT interval are thought to do so by modifying the activity of the same ion channels responsible for the long QT syndrome. The effects of the different drugs range from mild to severe with the latter having the greatest probability of precipitating torsades de pointes.9 The ability of a drug to precipitate a malignant tachyarrhythmia is related not just to the drug itself but also to the dose, to drug interactions, to genetic factors, to the gender of the patient, and to the type and severity of preexisting cardiac disease.
Ventricular fibrillation is the cause for most of the other instances of sudden cardiac arrest with a relatively small proportion of people developing asystole as the initial manifestation of sudden cardiac arrest. The true incidence of the precipitating arrhythmia is not known because most sudden cardiac arrests occur out of hospital and typically, several minutes pass before the rhythm can be assessed. The cardiac diseases that lead to the genesis of ventricular fibrillation resulting in cardiac collapse are varied, and the association with sudden death in some cases is not well understood. Similar to patients who have cardiovascular collapse from ventricular tachyarrhythmias, there are patients who develop ventricular fibrillation who do not have identifiable structural heart disease. There is a correlation between what is normally considered to be benign, early repolarization (defined as an elevation of the QRS–ST segment of at least 0.1 mV in the inferior or lateral leads on the electrocardiogram) and the development of ventricular fibrillation.10,11 As such, early repolarization may be a sign of electrochemical imbalance within the myocardium.
Independent of the etiology of the cardiac collapse, for resuscitation to be successful, CPR must be initiated as soon as possible. Weisfeldt and Becker12 have proposed a three-phase model of resuscitation: the electrical phase, the circulatory phase, and the metabolic phase. The electrical phase begins with the cardiac collapse and lasts for approximately 4 minutes; the circulatory phase represents an approximation of 6 minutes window from 4 to 10 minutes; and the metabolic window which commences at approximately 10 minutes. The best outcomes are achieved if the heart is defibrillated within the first 4 minutes after the cardiac arrest, during the electrical phase. This observation has been borne out by studies of patients who have an implantable cardioverter defibrillator. One meta-analysis of three large such studies that compared the administration of amiodarone to implantation of an implantable cardioverter defibrillator demonstrated decreased arrhythmias and improved survivability in patients who had an internal defibrillator.13 In a canine model, Yakaitis and colleagues14 demonstrated that for defibrillation per se to be successful, the electroshock had to be delivered within 3 minutes of the onset of ventricular fibrillation. Once the circulatory phase has begun, the most important intervention to achieve the return of spontaneous circulation (ROSC) is to commence chest compressions that will deliver some oxygenated blood to tissues. Another animal study showed that once the circulatory phase has begun, a threefold improvement in results is obtained if chest compressions are performed first, along with the administration of epinephrine and then defibrillation attempted compared to performing the defibrillation first and then performing chest compressions.15
Once the patient transitions into the metabolic phase, the chances of ROSC and more importantly, the chances of a full neurologic recovery are quite low—neither immediate countershock nor chest compressions followed by countershock are associated with good outcomes. Investigators have speculated that even with ROSC, 10 minutes or more of global ischemia induces cells to release cytotoxins,16 and bacteria are more able to translocate across the intestinal endothelial barrier.17 Cytotoxins and endotoxin may further injure an already damaged heart, and worse, may disrupt mechanisms maintaining cellular integrity.18 As circulation returns, the hyperoxia that is often present will exacerbate the reperfusion syndrome and has been associated with increased mortality.19
In those patients with ROSC, the enthusiasm clinicians had for the ability of hypothermia to attenuate the neurologic injury that many of these patients developed has been tempered by a more recent well-performed study that did not demonstrate an improvement in neurologic outcome with hypothermia (33°C vs. 36°C).20
Once patients enter the metabolic phase after 10 minutes of untreated cardiac arrest, the chances of meaningful recovery are slim. Improvement in outcome in patients who do have ROSC will require development of therapies to attenuate the ischemia-reperfusion syndrome.
Hemorrhagic Shock
In patients who sustain traumatic injury, hemorrhage accounts for 30% to 40% of the deaths. Of these, a majority occur before the patients arrive at a hospital. Among traumatized patients who arrive at the hospital, mortality in the first several hours correlates with inadequate resuscitation and the presence of coagulopathy.21 During the recent combat experiences in Iraq and Afghanistan, particularly during the later years of the combat in Afghanistan when dismounted complex blast injury was recognized, there was a strong correlation between the degree of hemorrhage, the severity of the injury, and the overall mortality.22 However, service members who were alive upon arrival at a level III medical facility had a greater than 95% chance of survival. These results were obtained because of the military’s emphasis on controlling hemorrhage at the point of injury, recognition and treatment of acute traumatic coagulopathy (ATC), and the use of damage control surgery. In both civilians and wounded warriors, it is impossible to quantify the amount of blood that is lost from the intravascular circulation, but an estimate can be made from the amount of blood transfused, the degree of lactic acidosis, and the alterations and vital signs. In an observational study of patients who refused blood transfusions because of religious beliefs, there was no 30-day mortality among patients whose hemoglobin was greater than 7.1 g/dL. However, as the hemoglobin level decreased below 6 g/dL, mortality increased significantly.23
Although the volume of blood lost cannot be accurately measured, the number of units of blood given and changes in coagulation factors are readily recorded and analyzed. Improvements in early hemorrhage control and resuscitation and the prevention and aggressive treatment of coagulopathy appear to have the greatest potential to improve outcomes in severely injured trauma patients. A cascade of life-threatening medical problems can begin with severe hemorrhage, and many of these occur simultaneously: (a) hemorrhage, (b) impaired resuscitation, (c) shock, (d) inflammation, and (e) coagulopathy. The severity of each problem is commonly associated with the extent of overall blood loss. Low blood pressure due to blood loss indicates immediate complications, including the incidence of multiple organ failure and life-threatening infections.
Respiratory Arrest
Respiratory and cardiac arrest are distinct, but if untreated, one inevitably leads to the other. There are a multitude of etiologies of respiratory arrest, and respiratory arrest per se implies any process that inhibits the delivery of sufficient oxygen to the mitochondria to maintain aerobic metabolism. The respiratory centers in the brainstem can be injured by penetrating injury and by increased intracranial pressure due to infection or intraventricular hemorrhage. Even blunt trauma to the head of sufficient force can produce a similar result. Atkinson et al.,24 using a mechanical blow to the head, demonstrated in a rodent model that as the force of the blow incrementally increased, there came a point that the animal became completely apneic. There are a number of drugs that can depress the respiratory centers, most notably opioids, sedatives, and hypnotics. These drugs can suppress ventilation or induce complete apnea even at low doses in patients with central sleep apnea,25 in patients with chronic obstructive pulmonary disease (COPD) and hypercarbia, and in geriatric patients with comorbid conditions. Although it is true that opioids suppress ventilation, some have used this relationship to justify withholding opioids from patients with severe COPD at the end of their lives.26 One study of over 2,000 patients with severe COPD found that there was a correlation between the dose of opioids and mortality at high doses, but at lower doses, the effect was not seen, and yet at lower doses, the patients’ dyspnea was atenuated.27
Whatever the cause, be it through a central nervous system mechanism, or a peripheral mechanism such as glottic edema from anaphylactic shock, or airway disruption from trauma or a foreign body, death does not occur immediately. The hemoglobin in the circulating blood carries enough O2 for maintenance of aerobic metabolism for 1 to 3 minutes. In addition, there is O2 that has already entered the lungs. During normal breathing at end expiration, there is O2 in the functional residual capacity (FRC) that can be calculated as the FIO2 times the volume of air in the FRC which in turn is equal to the person’s weight in kilograms times 15 mL/kg. For a 70-kg person, breathing room air the FRC would contain approximately 220 mL of O2 ([70 kg × 15mL/kg] × .21), enough to meet metabolic demand. At 3 to 4 minutes of complete apnea, evidence of tissue ischemia would become apparent and at longer than 5 minutes, irreversible damage would occur, especially in the brain. Cardiac arrest would soon follow unless oxygenation and ventilation were immediately rapidly restored.
Other causes of hypoxemic death are related to the neuromuscular system. A spinal cord transection above C4 would result in apnea because the phrenic nerves arise from C1 through C4. Likewise, patients with amyotrophic lateral sclerosis in which upper and lower motor neurons in the motor cortex, in the brainstem, and in the spinal cord die over time are unable to maintain respiratory effort. The cause is not understood but genetic factors play a role.28 Neuromuscular blocking agents produce the same effect albeit more quickly. Any process that decreases the alveolar to arterial O2 gradient can likewise interfere with the delivery of O2 to tissues, for example, drowning or acute respiratory distress syndrome. Cyanide poisoning is the most well-known cause of inhibition of O2 use within mitochondria by inhibiting cytochrome C oxidase. Adequate oxygen is supplied but cannot be used for aerobic metabolism.
All the causes of hypoxemic death discussed so far come about from inhibition or destruction of the body’s normal physiologic mechanisms. There are also environmental factors that play a role as well, the most important of which would be any factor that decreases the FIO2 would interrupt aerobic metabolism. The best example for anesthesiologists would be a helium quench from a magnetic resonance imaging scanner. If the required safety valves in the room were not working correctly, the helium could displace all the O2 in the room.
Pharmacology
Cardiopulmonary Resuscitation
The primary goal when resuscitating a patient from cardiac arrest is the ROSC, which is best achieved with effective chest compressions delivered immediately by a bystander, and if the patient has ventricular fibrillation, the delivery of electroshock therapy to defibrillate the heart. However, if these maneuvers are unsuccessful in restoring cardiac function and an advanced practitioner is present, drug therapy has been shown to increase the rate of ROSC. Recommendations for advanced cardiac life support (ACLS) are continually updated and provided by the American Heart Association. Although the drugs currently recommended for ACLS improve the likelihood of ROSC, none has improved long-term outcome.29
Epinephrine
Epinephrine is a catecholamine (vasoactive compound with a catechol—benzene ring with two hydroxyl groups [Fig. 47-2] in its structure). Dopamine is its precursor, and in turn, epinephrine is the precursor for norepinephrine. Chromaffin cells in the adrenal medulla and the terminal boutons of certain nerves produce and release epinephrine, which gains its biologic activity by binding to α- and β-adrenergic receptors. Among its many effects, epinephrine activation of α receptors produces vasoconstriction in the peripheral vasculature, whereas activation of β receptors within the heart increases chronotropy, inotropy, dromotropy, and lusitropy. The majority of epinephrine produced is metabolized within the same cells; it was synthesized simply because there is so much leakage of the catecholamine from the vesicles where it is stored into the cytoplasm. There is a very dynamic equilibrium between vesicular and cytoplasmic epinephrine.30

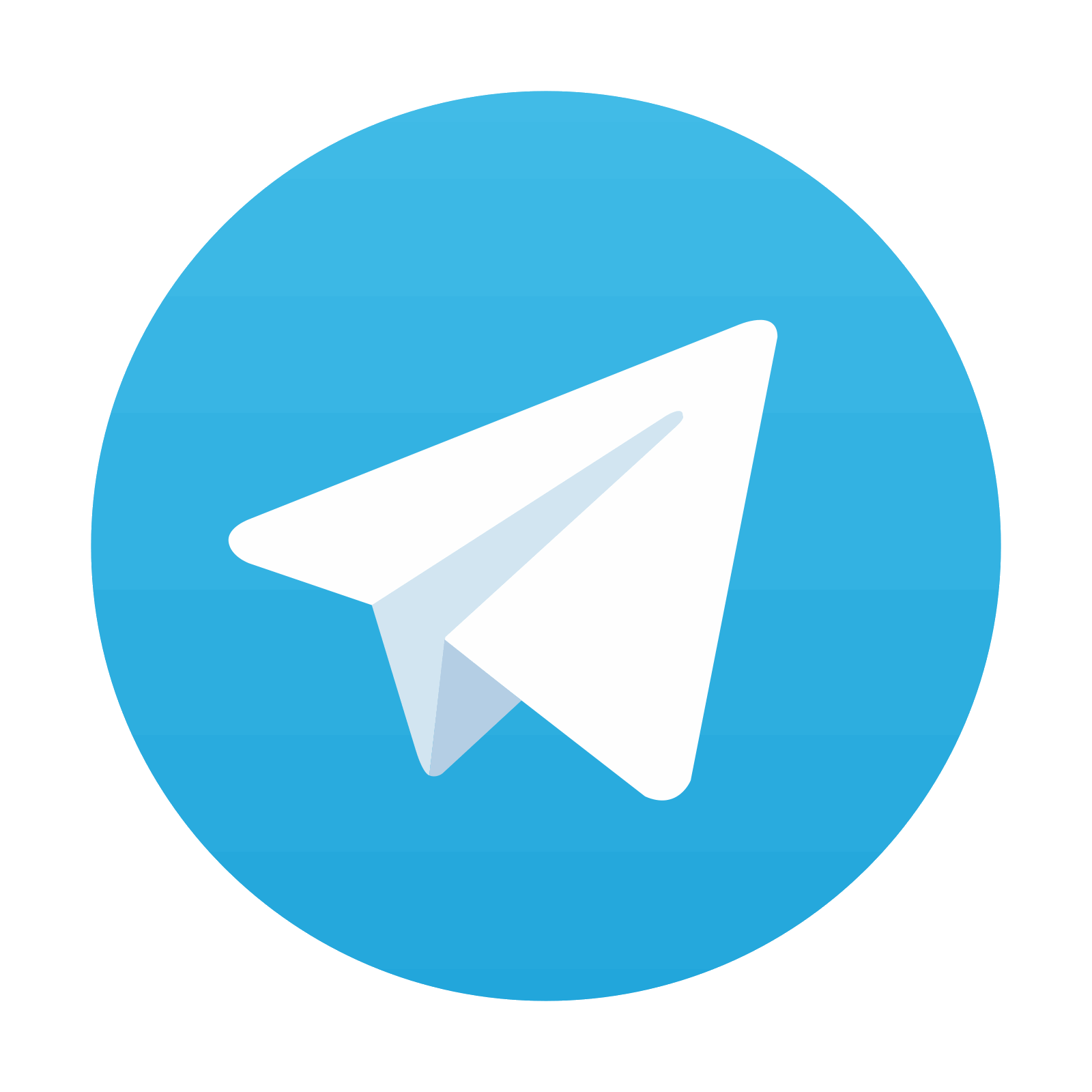
Stay updated, free articles. Join our Telegram channel
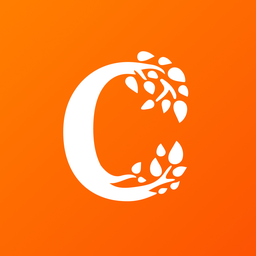
Full access? Get Clinical Tree
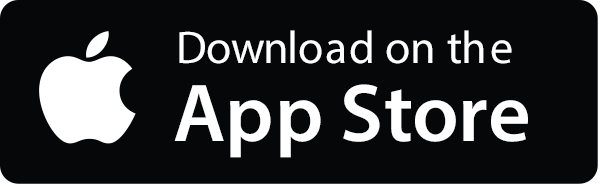
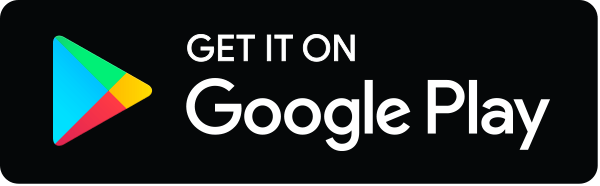