Fig. 2.1
The fetal circulation is characterized by high pulmonary arterial mean arterial pressure and pulmonary vascular resistance and low pulmonary blood flow. At birth, the dramatic decrease in pulmonary vascular resistance is accompanied by a parallel decrease in pulmonary arterial blood pressure and an increase in pulmonary blood flow. Of note, the pulmonary vascular resistance gradually decreases further over the first 6 weeks of life (from Rudolph [31])

Fig. 2.2
The fetal circulation. Desaturated blood from the superior vena cava preferentially flows into the right ventricle, into the pulmonary artery, across the ductus arteriosus and to the descending aorta to the placenta. Relatively well-saturated blood from the ductus arteriosus enters the inferior vena cava and preferentially crosses the foramen ovale to the left atrium, the left ventricle, and to the cerebral circulation (from Marx [32])
The umbilical vein enters the hilum of the liver where it divides into three branches: vessels that provide flow directly to the left lobe, a large arcuate branch that joins the portal vein to supply the right lobe, and the ductus venosus that proceeds cephalad to join the inferior vena cava (Fig. 2.3). Umbilical venous blood flowing into the inferior vena cava is preferentially directed across the foramen ovale into the left atrium and then the left ventricle. Thus, highly oxygenated blood exits the placenta, bypasses the liver, and flows directly to the myocardium and brain. Desaturated blood from the superior vena cava and the abdominal vena cava enters the right atrium and ventricle and then returns to the placenta after crossing the ductus arteriosus. A variety of anatomic features of the atrial septum (e.g., crista dividens, eustachian valve) and the angle of entry of the ductus venosus into the inferior vena cava facilitate this preferential streaming [33].


Fig. 2.3
The fetal circulation. The blood from the umbilical vein that enters the ductus venosus preferentially streams across the foramen ovale to enter the left atrium and ventricle and the cerebral circulation (from Rudolph [34])
Although reduced throughout gestation, pulmonary blood flow in the human fetus increases from ~13 % of the combined ventricular output at mid-gestation to 25 % at 30 weeks [35]. In addition, the pulmonary vascular resistance of human fetuses responds to maternal oxygen administration (FIO2 = 0.60) after 31 weeks gestation [36]. Similar studies in fetal lambs documented that the fetal circulation is briskly responsive to both hyperoxia and hypoxia and that these responses are greater in late gestation (Fig. 2.4) [38]. Vasomotor control of the pulmonary circulation in utero, however, may be disturbed in the presence of a congenital heart defect in which oxygen delivery to the lungs may be abnormal (e.g., aortopulmonary transposition) thereby dramatically affecting the development of vessel morphology [37]. At birth, when the placenta separates, pulmonary vascular resistance decreases dramatically, and pulmonary blood flow increases in response to the rapid increase in oxygen tension and the onset of alveolar ventilation (Fig. 2.1). Simultaneously, both systemic vascular resistance and left atrial pressure increase, eliminating the right-to-left shunting through the foramen ovale. However, bidirectional shunting through the ductus arteriosus may continue in the normal full-term infant during the first 48 h of life. With normal transition, the distinct, separate pulmonary and systemic circulations are established.


Fig. 2.4
Response of the fetal pulmonary circulation to hypoxia (fetal lamb). With advancing gestation, the response of the pulmonary circulation to decreasing oxygen saturation becomes more pronounced (from Rudolph [37])
Postnatally, the marked vasoconstrictor responses of the pulmonary circulation to hypoxia persist, and pulmonary vascular resistance also responds significantly to changes in pH (Fig. 2.5) [39]. This may be due in part to the persistent increase in pulmonary vascular resistance during the first few weeks of postnatal life and greater than that in the older infant despite decreasing dramatically at birth (Fig. 2.1). The reactivity of the pulmonary vasculature can be striking, and, in the neonate, arterial hypoxemia or acidosis can vasoconstrict the pulmonary arteries and induce pulmonary hypertension, thereby impeding forward blood flow and, by default, forcing right-to-left shunting through the foramen ovale and/or ductus arteriosus. This return to a fetal circulatory pattern, termed persistent fetal circulation or persistent pulmonary hypertension of the newborn (PPHN), further exacerbates the hypoxemia and acidosis. PPHN may be an isolated phenomenon or a part of various clinical scenarios including meconium aspiration, sepsis, polycythemia, and diaphragmatic hernia. An echocardiogram is routinely performed in infants manifesting signs and symptoms of persistent pulmonary hypertension to definitively exclude structural cyanotic heart disease [40].


Fig. 2.5
Response of the neonatal pulmonary circulation to hypoxia and acidosis (lamb). The break point for marked increase in pulmonary vascular resistance in response to decreasing oxygen saturation is dramatically dependent on pH (used with permission from Rudolph and Yuan [39])
Although the control of the pulmonary circulation, especially during the transition from fetal to postnatal life, is complex and depends on the interaction of a variety of mediators and factors, receptors, and neurologic, endocrine, and vascular control mechanisms, nitric oxide (NO) undoubtedly plays a critical role in mediating the vasodilating effect of oxygen [41], although other mechanisms contribute as well (e.g., oxygen-sensitive K+ channels in pulmonary vascular smooth muscle) [42]. Despite the enormity of the research that has been focused on this topic, no single factor has yet been identified as the primary trigger responsible for the initiation of pulmonary vasodilatation at birth, nor do we know whether the endothelial cell or the smooth muscle cell is the prime target [43].
A wide range of therapies and agents (hyperventilation, vasoactive agents) have been investigated to treat PPHN, but the only selective vasodilator of the pulmonary circulation is NO. In many cases, inhaled NO induces a selective, rapid, and potent decrease in pulmonary vascular resistance without affecting systemic vascular resistance [43]. At low doses, toxicity is minimal. Unfortunately, up to 40 % of infants with PPHN do not respond to NO. The effects of NO on mortality, the incidence of bronchopulmonary dysplasia, and neurodevelopmental deficits have been conflicting [44, 45], as has its effects as an antioxidant and anti-inflammation (in animals) [46]. Finally, endogenous NO may have a role in alveolar and vascular development in the immature lung [47, 48]. Because production and metabolism of NO are regulated on several levels, it is not surprising that its clinical effectiveness in both term and preterm infants remains controversial. For example, NO production depends on endothelial nitric oxide synthetase activity (eNOS; lung eNOS is type III). eNOS has both reductase and oxygenase domains. When its substrate l-arginine is available, oxidation of NADPH and NO synthetase produces NO. On the other hand, when the concentration of substrates or cofactors (e.g., heat shock protein 90) is reduced, NOS is uncoupled, and reactive oxygen or nitrogen species such as peroxynitrite are produced instead of NO. In fact, oxidative stress may contribute to the development of PPHN [49]. Similarly, the activity of soluble guanylate cyclase and cGMP-specific phosphodiesterase (PDE5) on smooth muscle cells potentiates the effectiveness of NO in augmenting cGMP production. PDE5 hydrolyzes cGMP, which controls the duration and magnitude of cGMP’s effect. Agents that inhibit PDE5, such as sildenafil, promote pulmonary vasodilatation and have been used extensively to treat pulmonary hypertension in adults, although there is less experience with this therapy for PPHN in neonates [50].
Myocardial Development
Myocytes:
Fetal and adult myocardia contract and relax similarly. That is, with activation, the cytosolic calcium concentration increases, inducing force generation, and as the cytosolic calcium concentration decreases, relaxation ensues. At all stages of maturation, the ventricle develops force against a varying resistance or load (contraction/ejection) followed by a period of relaxation (filling). The membranes in the adult myocardium that control calcium flux and the contractile system that responds to calcium are present in the fetal heart; however, structures associated with the mechanics of force generation (sarcomere, myofibril), those correlated with controlling calcium flux (sarcoplasmic reticulum and other membrane components including receptors, channels, exchangers, transporters, and pumps), those related to myocardial compliance (extracellular matrix/cytoskeleton), and sympathetic innervation undergo qualitative and quantitative age-related changes. In part, age-related differences in cardiovascular function and responses to calcium and other pharmacologic agents can be attributed to the developmental state of these various components of myocardial anatomy and function.
During fetal and neonatal development, myocytes differentiate markedly and increase in number and size, which correlate with profound changes in mechanical properties and therefore contractility and performance. In the perinatal period, the myocytes complete “terminal differentiation” and lose their capacity to proliferate, as evidenced by their transition from mono- to binucleation (a final nuclear division without cellular division) [51]. Concurrently, the shape and size of myocytes change, gradually remodeling from a spherical to a more rectangular shape by adulthood. Since shortening typically occurs along the long axis, the adult myocyte (dimensions of the adult vs. neonate myocyte: 150 vs. 40 μm in length; 5 vs. 25 μm in width) generates a more rapid response and larger increase in amplitude than the neonatal myocyte [52].
Recent studies in both human [53] and animal [51] fetuses have documented that the late gestational exponential cardiac growth secondary to the increase in size and number of myocytes correlates with an increase in both left ventricular volume and active tension per unit of myocardial volume. Of possible importance in the setting of congenital heart disease (e.g., single ventricle associated with pulmonary or aortic atresia), marked differences between the development of the left and right ventricles have been noted, including fewer but larger myocytes in the right ventricle [51].
Subcellular Components:
In addition to the increase in the number of myocytes, age-related changes in subcellular components also contribute to maturation in excitation-contraction and force development in the myocardium and, therefore, cardiovascular function. Not only does the number of myocytes per cross-sectional area increase, but the organization of the myofibrils also undergoes striking age-related changes [52]. In the immature myocardium, myofibrils appear in thin layers, and groups of nuclei and mitochondria congregate chaotically in the center of the cell. In contrast, in the mature myocardium in adults, long parallel rows of myofibrils alternate with rows of mitochondria (and sarcoplasmic reticulum). The chaotic arrangement of mitochondria and myofibrils in the immature myocardium contributes in part to the reduced contractility compared with the adult heart.
In addition to the effects of age-related changes in gross anatomy, changes in various membranes that control the regulation of calcium flux also exert substantial effects on the force of myocardial contraction. Both the cell membrane (sarcolemma) and the intracellular sarcoplasmic reticulum modulate the increase in cytosolic calcium that facilitates contraction and undergo developmental changes. In the adult, a small calcium influx via l-type calcium channels stimulates calcium release from the sarcoplasmic reticulum (calcium-induced calcium release, CICR). CICR depends on an intricate coupling of the C-type calcium channel, the ryanodine receptor, and t tubules (invaginations of the sarcolemma) [54, 55]. In spite of differences among various species, in general, CICR has been allocated a secondary role in the generation of a myocardial contraction in the immature myocardium because of limited sarcoplasmic reticulum and underdeveloped t tubules [56, 57]. Recently, attention has focused on a role that links the Na+–Ca+ exchanger with the ryanodine receptor to allow an age-defined reverse version of CICR [58, 59]. An l-type calcium current mediated by CICR is now recognized to play an increasingly critical role as the myocardium matures, although the details of the molecular mechanisms remain unclear.
Clinically relevant developmental changes are pervasive in the developing myocardium including the sarcoplasmic reticulum, t tubules, and various channels and regulatory proteins. That is, the volume of sarcoplasmic reticulum and its ability to pump calcium (uptake, longitudinal sarcoplasmic reticulum; storage and release, junctional sarcoplasmic reticulum) increase in utero and postnatally. Furthermore, the various subtypes of sarcoplasmic reticulum are less differentiated functionally in the immature heart. As a result, immature hearts are more sensitive to calcium channel antagonists [60], and maximal contractility depends to a greater extent on extracellular calcium that does the mature heart [61].
When compared with the adult myocyte, the reduced velocity and magnitude of sarcomere shortening in the immature myocyte may also be attributed to age-related changes in expression of various isoforms of contractile proteins such as troponins [62]. The troponin complex, which consists of 3 subunits (structural proteins: troponin C, I, and T) and binds to the thin filament of the myofibril, regulates the calcium-dependent activation of actin and myosin interaction that results in force generation. Both troponin I and T exist in multiple isoforms that are developmentally regulated. For example, an isoform identical to that in slow skeletal muscle (ssTnI) is expressed in embryonic, fetal, and neonatal myocardium, whereas the cardiac isoform (cTnI) is expressed predominately in the adult myocardium. The myocardial expression of the slow skeletal muscle in terms of the cardiac isoforms of troponin I may be of particular importance in the immature myocardium since this has been correlated with the relative resistance of the immature heart to acidosis [63]. The response of the immature myocardium to sympathetic stimulation is similarly correlated with the expression of slow skeletal troponin I. Cardiac, but not slow skeletal muscle troponin, is phosphorylated in response to β-stimulation, which may correlate with the difference in diastolic function noted in the neonate [64]. This phosphorylation decreases the sensitivity to calcium, facilitating diastolic relaxation.
TnT isoforms vary among species and stages of development. The human cardiac muscle contains four isoforms of TnT (cTnT 1–4); cTnT1 expression peaks in the fetus, whereas only cTnT3 is expressed in the adult. Calcium sensitivity has been correlated with the shift in the expression of the troponin T isoform [62]. The expression of specific isoforms of troponin T has been correlated with the responsiveness of myofilaments to calcium.
Although cardiac troponins are routinely used to evaluate and monitor cardiac injury in children and adults, only recently has their importance in the intensive care nursery been recognized [65]. Troponins are typically bound to the thin filament of the myofibril, but with acute injury to the myocardium, bound troponin is released from damaged tissues, first appearing in blood after 2–4 h and persisting for up to 21 days. The range of normal concentrations of the isoforms of troponin in the neonate has been reported for both cTnT and cTnI. The concentrations of troponins in cord blood samples vary as a function of gender, mode of delivery, and assay. Both cTnT and cTnI increase after asphyxia-associated myocardial injury, although the variability in the responses among studies is notable. As in children and adults, laboratory evidence of injury is often an adjunct to other assessments (e.g., echocardiogram) of cardiac function. For example, asphyxiated neonates have greater concentrations of cTnT than normal-term infants that correlate with echocardiographic evidence of myocardial dysfunction. Nonetheless, in many cases, cardiac output is similar in asphyxiated and non-asphyxiated neonates. The concentrations of troponins are generally increased in preterm infants, although treatment with inotropes correlates with greater cTnT concentrations in both term and preterm neonates. Thus, although troponin concentrations in neonates are likely to become an integral segment of evaluating perinatal injury in both preterm and term infants, its specific prognostic and therapeutic roles have not been completely defined.
Myocardial Compliance: Extracellular Matrix/Cytoskeleton
The cytoskeleton includes the contractile proteins and titan (a large protein that extends over half the span of the sarcomere) as well as microfilaments, intermediate fibers, and microtubules. This intricate complex provides the structural framework for intracellular and extracellular communication that allows the contractile movement of the individual sarcomeres to be translated into effective systolic contraction and diastolic relaxation. That is, the cytoskeleton provides the system for mechanical signaling. Examining the general appearance of the immature sarcomere provides an overview of the dramatic changes in organization during early development. In the immature myocyte, A and I bands are more irregular, M bands are absent, and Z bands vary in width. Several of the proteins and microfilaments, intermediate fibers, and microtubules develop postnatally, which is critical in mediating a range of activities such as cell growth, migration, and adhesion as well as signaling for remodeling in response to adaptation of the transitional circulation at birth [33]. For example, desmin, a protein important that links Z bands of myofibrils, improves the connection of myofibrils with mitochondria facilitating the mechanics of contraction. The amount and types of collagen change their expression of various isoforms that improve resting load and passive state of the myocardium. For example, the type I isoform correlates more tightly with developing rigidity, whereas the type III isoform contributes to elasticity, and the relative proportion of these two correlates with myocardial compliance. As the collagen network becomes progressively more organized with maturation, the population of type III isoform increases, eventually equaling or exceeding that of type I [66]. That is, the ratio of types I–III isoforms is increased in preterm and term infants, a level that persists until at least age 6 years and decreases thereafter to ~0.5 by adulthood [66].
Sympathetic Innervation:
The sympathetic nervous system modulates cell growth and differentiation, as well as the distribution of and sensitivity to calcium. For example, the increased concentration of α-adrenoreceptors in the early postnatal period may be critical in stimulating left ventricular growth [67]. Furthermore, the increase in the network of adrenergic fibers innervating the myocardium induces widespread development and expression of the various contractile systems (e.g., the expression of the contractile proteins, the efficiency of the calcium channel, expression of the myosin ATPase isoforms). Finally, the activity of adenylate cyclase, an important enzyme involved in the intracellular transmission of β-stimulation, increases in parallel with the increase in catecholamine concentrations [33].
The Preterm Infant
The typical changes of the transitional circulation are blurred in the setting of the very low birth weight (VLBW; <1,500 g) and extremely low birth weight (ELBW; <1,000 g) infant. At less than 30 weeks gestational age, the ductus arteriosus often fails to close, systemic and pulmonary vascular resistances are high relative to the placental circulation, and, often, cardiac output does not increase dramatically in the first 24 or more postnatal hours. Because of the unique physiology of the ELBW infant, measuring cardiac output and establishing “normal” values for systemic blood pressure and heart rate become complicated. As a result, defining hypotension becomes difficult (see below) [68]. Consequently, diagnostic and therapeutic regimens in the intensive care nursery cannot be established based on clear-cut, abundant, evidence-based data for these infants.
At any developmental stage, ventricular function is governed by the same factors: preload, afterload, contractility, and heart rate. However, the ability to compensate for perturbations in these factors is limited in the neonate, especially in ELBW infant. In the preterm infant (i.e., in some cases, a mid-gestation fetus), the immature myocardium and the peripheral circulation present significant disadvantages when, at birth, the low-resistance placenta is abruptly replaced with the greater resistances of the pulmonary and systemic vascular beds, and interventions such as positive pressure ventilation and inotropic support are introduced. Sensitivity to changes in heart rate is also more dramatic for the preterm infant. Marked variability in the quantity of blood flowing across the foramen ovale and ductus arteriosus adds to the complexity of monitoring the cardiovascular function of the premature infant.
The relationship between blood pressure, cardiac output, and systemic vascular resistance is constant throughout life: BP = CO X SVR. That is, pressure and flow are not equal but are related via resistance (or SVR). Thus, flow to an organ may increase, decrease, or remain constant over a wide range of blood pressures depending on changes in resistance. Although the normal ranges for blood pressure correlate with gestational age [69, 70], the definition of hypotension remains elusive. Because a mean arterial pressure <30 mmHg for more than 1 h (measured on the first day of life, beginning at 5 h) has been associated with intracranial lesions [71] or reduced cerebral blood flow [72], many set a break point for hypotension at 30 mmHg in preterm infants. However, there are those who hold that the normal mean arterial blood pressure is <30 mmHg in the most preterm infants during the first 3 postnatal days (Fig. 2.6) [73]. Others suggest that a mean blood pressure that is less than the gestational age correlates with the 10th percentile for blood pressure [74], and this constitutes a definition of hypotension. Although studies have reported an association between hypotension and intraventricular hemorrhage [75, 76], a clear cause-and-effect relationship remains controversial. That is, aggressive treatment of “hypotension” has not been shown in prospective studies to affect morbidity or mortality [68] or long-term outcome [77]. And, unfortunately, identifying links between a specific inotropic agent, change in cerebral blood flow, and outcome remains at best an estimate. No study “has shown any improvement in any meaningful clinical outcome, short or long term, in response to a specific inotrope” [78].


Fig. 2.6
Mean blood pressure in neonates predicted lower limit (initial 72 h of life) (from Nuntnarumit et al. [73])
Thus, the variability in “normal” for blood pressure and heart rate among infants of the same gestational and/or postnatal age creates a dilemma in the setting of clinical care, especially in the commonly unstable setting of the operating room. Furthermore, without access to reliable measures of perfusion to critical organs (i.e., the brain), devising precise guidelines for treating “hypotension” in the neonate is virtually impossible.
A number of investigators [79, 80] have proposed a novel next step to monitor cerebral blood flow in the setting of the transitional circulation of the preterm infant in the first few postnatal days. Recognizing that neither blood pressure [79, 80] nor capillary refill time [81] reliably correlates with left ventricular output in the preterm infant, the flow in the superior vena cava has been measured to estimate systemic blood flow to the brain (and upper body). Measuring blood flow of the superior vena cava eliminates the need to consider the influence of shunting via either the ductus arteriosus or the foramen ovale on the accuracy of their measurement [82]. In these studies, mean arterial pressure correlated poorly with superior vena cava flow (Fig. 2.7). Similarly, others noted that during the first 48 h of life, blood pressure did not correlate with the volume of blood flow in the descending aorta, superior vena cava, or left or right ventricle [80]. In fact, at some points, if any relationship between blood pressure and blood flow in the superior vena cava existed, it would most be accurately labeled as being “inverse,” as reported in earlier studies [84]. Of interest, neither dopamine nor dobutamine increased contractility when introduced in response to reduced superior vena cava blood flow [85].


Fig. 2.7
Ultrasound measured flow in the superior vena cava has been validated as an estimate of cerebral blood flow. Notice the lack of correlation of SVC flow with simultaneously measured mean arterial blood flow (from Kluckow and Evans [83])
Measuring superior vena cava flow (and other flows) may improve the accuracy of ensuring adequate oxygen and nutrient delivery, especially to the brain, and monitoring the effectiveness of therapy. For example, superior vena cava flow has been correlated with both the incidence of periventricular hemorrhage and neurodevelopmental outcomes [83, 86]. Unfortunately, “functional echocardiography” requires sophisticated equipment and experience precluding its use as a routine, bedside monitor.
Thus, given our inability to accurately measure the cardiac output and organ-specific blood flow (e.g., cerebral blood flow) in the neonate (Fig. 2.7), we are left with systemic blood pressure and heart rate and metabolic indices including blood gases/lactate/electrolytes and trends in clinical status to track cardiovascular homeostasis in the preterm infant. In fact, even investigators who have reported the relevance of superior vena cava flow have suggested a rational approach to clinical care based on commonly available cardiovascular indices as mentioned [78]. As always, a parameter such as blood pressure must be interpreted within the wider context of other clinical and diagnostic data. For example, even if superior vena cava flow were measured, these data might be correlated with MRI and near-infrared spectroscopy [see Section “Monitoring Cerebral Blood Blow: Near-Infrared Spectroscopy (NIRS)”] to establish rational therapies.
Clinical Significance and Summary
The neonate has the greatest cardiac output per body weight of all age groups (~300 cc/kg/min). The increased resting cardiac output of the neonates limits its ability to respond to an increased oxygen demand or to adapt to wide variations in preload or afterload. That is, the neonate cannot readily compensate for inadequate blood flow if the preload becomes inadequate or if the afterload, heart rate, or contractility wane. For example, the non-distensible heart has limited capacity to increase stroke volume to augment cardiac output in response to increasing preload [87, 88]. Although volume loading in the immature ventricle increases cardiac output, the effect is attenuated compared with the response at older ages. Similarly, the immature myocardium poorly tolerates increases in afterload compared with that at older ages. The increased content of collagen and high ratio of types I–III collagen may account for the relative noncompliance of the neonatal heart. These factors are all magnified in the case of the ELBW infant where marked variability exists for blood flow across the foramen ovale and ductus arteriosus. Finally, the normal heart rate in the neonate is quite rapid. Increasing the heart rate may not further increase the cardiac output, but decreasing the heart rate may dramatically decrease the cardiac output. Some propose that the resting myocardium in the neonate exists at a greater level of “β-adrenergic tone” than does the child and adult. As this β-adrenergic tone wanes over the first weeks to months after birth, adrenergic stimulation elicits a greater increase in cardiac performance [89].
The fetus (i.e., preterm baby) and neonate may have impaired ventricular function secondary to a decreased number of myofibrils, decreased sympathetic innervation, decreased β-adrenoceptor concentration, immaturity of the sarcoplasmic reticulum structurally and functionally, maturation-specific mechanisms for calcium uptake, release and storage, and a specific spectra of expression of various isoforms of contractile/noncontractile proteins, channels, exchangers, and enzymes. Over the first months of life, myocardial contractility gradually increases, which maintains cardiac output over wider ranges of preload and afterload. Similarly, the increase in contractile proteins and the shift in expression to various isoforms, the development of sarcoplasmic reticulum and t tubules, and adrenergic innervation all contribute to complex changes in force development, calcium recruitment, and transport that prime the myocardium for a more powerful response to stress and increased oxygen demands.
Central Nervous System Function
Discussing the central nervous system after cardiovascular function is intentional, since the physiologic vulnerability of the immature brain is inextricably linked to age-related hemodynamic function. Circulatory immaturity undoubtedly plays a fundamental role in perinatal brain injury. Hemodynamic instability is common in the neonatal period (especially in the preterm infant) and has been implicated in ischemic and hemorrhagic brain injury in both term and preterm infants. Unfortunately, cerebrovascular injury has been linked to lifelong dysfunction including motor disorders, learning/developmental delays, seizures, and secondary complications (e.g., from chronic lung disease secondary to recurrent aspiration pneumonia). Minimizing or preventing neurologic complications from perinatal injury requires a complete understanding of the cellular and molecular mechanisms of the responses of the developing brain to asphyxia, hypoxia, and/or inflammation. To date, such mechanisms have not been definitively mapped, and the extent and nature of the initial injury cannot clearly predict long-term outcomes.
The general pattern of injury to the developing brain involves an initial insult from hypoxia, inflammation, and/or ischemia followed by secondary damage from reperfusion that results in excitotoxicity and release of a host of cytokines [90]. Recently, Volpe introduced the concept of “panencephalopathy” [91] and “encephalopathy of prematurity” [92–94] to emphasize that the initial white and gray matter injury incurred by the preterm infant is manifested by more than a simple loss of tissue but also significantly impairs subsequent brain development. Volpe emphasizes that “neonatal brain injury and its subsequent clinical and anatomic consequences must be viewed as an amalgam of destructive and developmental disturbances.” Kinney asserted that the term “encephalopathy of prematurity” emphasizes that “the constellation of cognitive, motor, and emotional impairment in long-term survivors of prematurity reflects the particular patterns of white and gray matter damage in combination with arrested developmental programs—patterns that depend upon the severity, timing, and chronicity of the injury as well as individual confounding factors” [95].
Normal Development of the Central Nervous System
The two primary structures of embryogenesis of the brain that develop early in gestation are the neural tube (future brain and spinal cord) by 3–4 weeks and the prosencephalon (future forebrain) by 2–3 months [96]. Early neural tube anomalies are dramatic and often fatal: anencephaly, craniorachischisis totalis, myeloschisis, and encephalocele. Similarly, severe prosencephalic anomalies (holoprosencephalies) are often fatal, especially when associated with chromosomal abnormalities (e.g., trisomy 13–15, trisomy/ring/deletion 18).
The less severe form of abnormal neural tube closure, lesions of spina bifida (incidence 3–7 per 10,000 births) are clinically important because affected infants frequently survive and have lifelong problems. The four primary types of spina bifida are categorized according to the severity of the defect [96]. In spina bifida occulta, the divided vertebral arch, the spinal cord, and the meninges are covered with skin. Hair often protrudes from the skin overlying the defect, forming a sacral dimple. In spina bifida cystica, neural tissue and its coverings protrude through the incompletely formed vertebral arch as a cystic-like structure. In meningocele, the neural tube lies in its normal position, but the meninges protrude through the defect; skin usually covers this lesion. In the fourth type, the myelomeningocele, both the spinal cord and the meninges protrude, often without skin. These lesions can occur at any level of the spine. Although commonly accompanying myelomeningocele (60 % in occipital, cervical, thoracic, or sacral lesions and about 90 % in thoracolumbar, lumbar, and/or lumbosacral lesions) [97], hydrocephalus often is not evident before the meningomyelocele is closed because cerebrospinal fluid leaks through the open lesion and decompresses the ventricles.
Arnold-Chiari malformation, an abnormality of the hindbrain, occurs in most patients with myelomeningocele. The medulla oblongata is flattened and elongated and, along with the fourth ventricle, protrudes into the spinal canal through the foramen magnum. The downward position of the medulla elongates the lower pons and upper medulla and may compress brainstem nuclei and cranial nerves. If the cerebellar tonsils are displaced through the foramen magnum, aqueductal stenosis and hydrocephalus can develop. Severe anomalies may cause apnea, vocal cord paralysis, and/or central and obstructive ventilatory disturbances and require early correction [98]. Of significance, as many as 20 % of infants with myelomeningocele have sleep-disordered breathing [99].
Agenesis of the corpus callosum and septum pellucidum are often associated with abnormal neuronal migration and significant clinical abnormalities. Agenesis of the corpus callosum is usually associated with a syndrome (e.g., Aicardi or Andermann syndrome) or a chromosomal [11, 14, 16, 18, 21] abnormality. As many as 80 % of patients without a corpus callosum have other brain anomalies, plus they have non-CNS malformations [100, 101]. Partial agenesis of the brain probably occurs later in development and is associated with clinical syndromes that have migrational and structural disorders. Agenesis of the septum pellucidum is never an isolated lesion and often occurs in conjunction with optic nerve hypoplasia (septo-optic dysplasia).
During the third trimester, cortical gray and white matter volumes increase four to fivefold [93, 94], secondary to growth and differentiation of dendrites and axons, proliferation of glia, synaptogenesis, and myelination (Fig. 2.8) [102]. The proliferation in the cerebellum is even more rapid than the cerebral cortex and is characterized by intense migration of various populations of cells (Fig. 2.9) [104–106]. In parallel with the intense growth of the parenchyma, the vascular network of the late gestation brain also develops rapidly. The long and short penetrators lengthen and arborize, decreasing border/end zone blood flow.



Fig. 2.8
Growth of the human brain over the second half of gestation as a function of % of the weight for the full-term infant. The 34-week gestation brain is only 65 % of its weight at term (from Kinney [102], Fig. 1, p. 82)

Fig. 2.9
Growth of the human cerebellum (5th, 50th, 95th percentile) during the last half of gestation as a function of % of the volume for the full-term infant. The 34-week gestation brain is only 65 % of its volume at term (from Volpe [103], Fig. 1, p. 1086)
Age-Related Patterns of Injury
In addition to the overall intense growth of the brain in late gestation, specific transient cell types define the unique susceptibility of the preterm brain to ischemia, inflammation, excitotoxicity, and free radical injury. That is, various populations of cells are critical in forming cerebral pathways and, at the same time, are exquisitely sensitive to injury. This selective vulnerability of specific populations of cells at different developmental stages (24–28 weeks vs. 28–32 weeks vs. 32–37 weeks vs. term) leads to predictable patterns of pathology. Three examples have received prominent attention by experts in neonatal neuropathology (see Section “Vulnerable Cell Populations”).
In general, the preterm infant is regarded as most vulnerable to white matter injury, whereas the term infant is regarded as vulnerable to injury in the deep gray nuclei (e.g., basal ganglia and thalamus). Emerging evidence suggests that periventricular white matter in premature infants is selectively vulnerable to ischemia during hypotension [107]. The pattern of clinical deficits in survivors of hypoxic-ischemic perinatal brain injury also differs between term and preterm infants. Recent evidence suggests that the new knowledge about brain development acquired from advanced MRI imaging (e.g., high-resolution MRI, spectroscopic imaging, diffusion tensor imaging) has produced a “blurring of the ‘gray-white’ dichotomy” [108]. Increasingly, white matter injury has been recognized in term infants, and gray matter injury has been identified in preterm infants. In addition, the detailed anatomy of injury identified via MRI imaging may be combined with the known selective vulnerability of various cell populations to estimate, first, the patterns of defective “connectivity” in brain development that develop subsequent to the injury and, second, to correlate these patterns with the clinical outcome identified during long-term follow-up.
Although glutamate plays a critical role in the proliferation, differentiation, and migration of developing cells, its excessive release in the setting of a hypoxic-ischemic injury contributes to a state of “excitotoxicity” that is associated with cell death. When glutamate receptors (NMDA, N-methyl-d-aspartic acid; AMPA, alpha-3-amino-hydroxy-5methyl-4-isoxazole propionic acid; kainate) are excessively stimulated (especially certain subtypes), intracellular calcium accumulates and activates caspase-3, which leads to abnormal apoptosis. The NMDA receptor predominantly mediates this activity, and one subunit (NR2B) that predominates early in gestation mediates slower deactivation, resulting in prolonged action compared with other subunits [109].
In addition to excitotoxicity, oxidative stress seems to be fundamental to neonatal brain injury. Of interest, areas of the brain expressing NMDA receptors also express neuronal (nNOS) and inducible nitric oxide synthase (iNOS) [110]. While endothelial nitric oxide (eNOS) mediates cerebral vasodilatation, excessive nitric oxide mediated by iNOS in response to hypoxia-ischemia generates reactive nitrogen species (free radicals). The excess oxygen and nitrogen free radicals generated in response to hypoxia-ischemia or to free iron (Fenton reaction generates the hydroxyl radical when hydrogen peroxide encounters free iron) [111, 112] associated with hemorrhage deplete endogenous antioxidant systems, damaging cell membranes and resulting in cell death. Oxidative stress seems to enhance excitotoxicity via regulatory effects on glutamate receptors [113]. Of note, antioxidant systems (e.g., Mg and Cu superoxide dismutase, catalase, vitamin A, and C) are also underdeveloped in the neonatal brain [109].
Vulnerable Cell Populations. The Preterm Infant:
Two unique cell types (subplate neurons and pre-oligodendrocytes) with marked sensitivity to hypoxia-ischemia populate the white matter of mid-gestation fetuses and express glutamate receptors. The subplate neurons located in subcortical white matter first appear at ~10 weeks gestation and peak in number between 24 and 32 weeks [109, 114], when the subplate zone is 4–5 times thicker than the cortical plate. The subplate serves as a waiting zone for cells and thalamocortical axons, until their final ultimate location in the cortex is defined [115]. By expressing various neurotransmitters and growth factors, this site orchestrates the migration of cells from the germinal neuroepithelium to distant sites (e.g., corpus callosum, basal ganglia, thalamus) and initiates formation of synapses. These neurons have abundant receptors for excitatory amino acids (e.g., glutamate) that are critical modulators for normal development, but hypoxia-ischemia may stimulate excessive release of these modulators, excitotoxicity, and white matter injury. Recent in vitro studies documented the relative sensitivity of subplate neurons to glutamate compared with other cortical cells [116]. Damage to the subplate may disrupt axon development in vital sites such as thalamocortical areas, impeding critical innervation and interaction, interrupting feedback between the cortex and thalamus, and leading to long-term functional consequences. The connectivity among various distant structures during a period of critical brain development has been postulated to correlate with deficits identified in the ex-premature in the specific areas of behavior, cognition, and other complicated higher cortical functions (e.g., executive function) [105, 117–120]. In humans, the subplate gradually involutes during the third trimester and disappears by 6 months after birth. Thus, the subplate neurons are a transient cell population that mediates essential thalamocortical development.
Before myelination and during the time of peak vulnerability to periventricular leukomalacia (PVL) (23–32 weeks) (see Section “Cerebral White Matter Injury”), the late oligodendrocyte progenitors (pre-oligodendrocytes) are the predominant cells that populate the subcortical white matter. While mature oligodendrocytes are relatively resistant, pre-oligodendrocytes are especially susceptible to injury from oxidative and excitotoxic stress and, when injured, fail to reach full maturation, resulting in diffuse hypomyelination and axonal disruption. Thus, injury to progenitors inhibits their maturation, allowing the persistence of a population of cells that exhibit excitotoxicity as well as fail to generate mature oligodendrocytes that are capable of myelination [121].
Finally, microglia are unique macrophages that are initially identified in normal brain myelination development. They are concentrated in the cortical white matter during the third trimester, but decline rapidly after 37 weeks of gestation [122]. Although microglia are critical during normal development (apoptosis, axonal development, vascular development), their abnormal activation secondary to infection/inflammation precipitates cytokine and glutamate release that result in reactive oxygen and nitrogen species [112].
Thus, after the neural tube and the prosencephalon are established, the central nervous system primarily develops by proliferation and migration. Neurons proliferate from ventricular and subventricular regions at every level of the developing nervous system. From the second to the fourth month of gestation, most proliferating cells are neurons. From the fifth month of gestation into adulthood, glia are primarily proliferating. Interruption of migration (e.g., from the subplate), abnormal activation (e.g., microglia), and/or failure of proliferation/maturation (e.g., pre-oligodendrocytes) are key factors underlying the pathophysiology of injury to the immature brain.
The risk of white matter injury and the associated neurodevelopmental abnormalities increase with systemic illness such as chronic lung disease [123] and necrotizing enterocolitis [124]. In addition, the critical care of neonates is complex with their pharmacologic therapy, ventilatory support, infections, seizures, and hemodynamic instability all interacting to produce a final outcome.
Vulnerable Cell Populations. The Term Infant:
The term infant experiences hypoxia-ischemia from several clinical sources [placental injury (e.g., abruption, infarct), birth trauma, umbilical cord prolapse/compression] that are distinct from those that commonly injure the preterm infant and others who are similar (e.g., infection/inflammation). In general, hypoxic-ischemic encephalopathy in the term infant primarily targets the deep gray nuclei, especially the basal ganglia. As in the preterm infant, the selective vulnerability is linked to overexpression of certain glutamate receptors (especially NMDA) and various subunits (e.g., NR2B > NR2A). In the basal ganglia, these receptors coexist with an abundant population of neurons that express nNOS, thus creating a site of increased susceptibility to injury. Additionally, free radicals generated from hypoxia-ischemia as well as from reactions with free iron (Fenton reaction produces the hydroxyl radical when hydrogen peroxide reacts with free iron) generated after hemorrhage may lead to oxidative damage [125].
Contrary to the traditional belief that injury at term is commonly associated with chronic in utero events (e.g., infection/inflammation), most encephalopathy at this developmental stage seems to be secondary to insults at or near birth [126–128] and evolves over the first few weeks (or months) of life. Since injury is relatively acute and evolves over time, neuroprotective therapy may be critically relevant for improving long-term outcome. For example, hypothermia has moved into the realm of standard therapy for the term infant with moderate to severe encephalopathy secondary to hypoxia-ischemia [129]. However, in spite of some evidence for its effectiveness, hypothermia is unlikely to be a panacea for preventing long-term complications associated with neonatal encephalopathy. Rather than relying on a single modality, many suggest that a multipronged approach to perinatal neuroprotection/rescue will be essential to improving outcome [111].
Advanced MRI techniques have identified two primary patterns of injury in the term infant [93, 108, 125]. First, a watershed pattern involves the vascular border/end end zones of the white matter that may extend into cortical gray matter after a severe insult. In general, watershed lesions are more often associated with cognitive than motor disorders. Second, the basal nuclei (basal ganglia/thalamus) pattern involves the deep gray nuclei that may extend throughout the cortex after a severe insult. That is, this pattern often includes diffuse cortical injury. At presentation, these neonates have had more severe clinical abnormalities including intensive resuscitation, severe encephalopathy, and seizures. In general, these lesions are typically associated with more severe cognitive and motor disabilities than in the watershed pattern. However, even when one pattern predominates, less severe damage is common in the other domain [127]. Thus, the spectrum of deficits on follow-up correlates with the pattern of injury on MRI, rather than simply the severity of the lesion.
Congenital Heart Disease:
For more than a decade, we have known that more than 50 % of newborns with congenital heart disease have microcephaly and neurologic deficits that correlate with later neurodevelopmental abnormalities [130]. More recently, impaired brain development and metabolism that resemble the pattern of the preterm infant have been identified preoperatively in full-term infants with congenital heart disease, especially complex cyanotic lesions (hypoplastic left heart syndrome/single ventricle and aortopulmonary transposition). This delayed development may predispose these infants to similar vulnerability to white matter injury as that seen in the preterm infant [131–134]. Their outcomes reflect a complex interaction between a focal brain injury (e.g., white matter injury) and its profound effects on subsequent brain development. Although the specific mechanism for the in utero delay in development is unclear, disturbances in the fetal circulation with decreased delivery of oxygen and other metabolites to the brain may play a fundamental role [33, 135]. Although complex cyanotic heart disease confers a particularly high risk of developmental delay, delay is also common in children with either acyanotic or cyanotic lesions, especially those who undergo surgical intervention in the first few days of life [136].
Summary:
That the brain of the 34-week gestation human weighs only 65 % of that of a term infant (Fig. 2.8) [102] implies dramatic development during the last month of normal fetal life. Overlap in the pathophysiology of lesions common in the preterm [periventricular leukomalacia (PVL) and germinal matrix-intraventricular hemorrhage (GM-IVH)] with those most common in the term infant (hypoxic-ischemic encephalopathy, arterial stroke) is not surprising. In part, the overlap can be explained by responses to injury in the setting of selective vulnerability of the immature/newborn brain coupled with disturbances in regulation of cerebral blood flow.
Autoregulation of Cerebral Blood Flow:
Cerebral auto-regulation accounts for a constant cerebral blood flow over a wide range of systemic blood pressure (in adults, between mean arterial pressures 60 and 150 mmHg) [137]. Such a phenomenon implies that vasoconstriction and vasodilation occur in response to changes in perfusion pressure to maintain a stable blood flow. Various vasoactive factors have been linked to local regulation of cerebral blood flow (e.g., hydrogen ions, potassium, adenosine, prostaglandins, osmolarity, and calcium) [111]. The term “pressure passive” refers to the disruption of this phenomenon so that changes in blood pressure alter cerebral blood flow. Pressure passive cerebral blood flow has been proposed as a critical risk factor for central nervous system injury in neonates and the subsequent adverse neurodevelopment, especially the preterm infant. Although autoregulation is intact in normal fetal/preterm and term animals and humans, the autoregulatory range is narrower in this age group compared with their more mature counterparts. Normal blood pressure increases markedly during the third trimester, but the upper limit of autoregulation does not. As gestational age decreases, the normal blood pressure in preterm infants is close to the lower limit of autoregulation [138–140]. Rapid increases in arterial blood pressure can rupture the fragile vessels in the immature brain (see Section “Germinal Matrix-Intraventricular Hemorrhage”), whereas hypotension and low perfusion pressure can cause ischemia. Thus, the cerebral blood flow of the preterm infant seems to be vulnerable to both hypo- and hypertension.
Greisen posited that in critically ill neonates, cerebral autoregulation should be presumed to be “imperfect,” not simply present or absent or “all or nothing.” That is, the flat part of the autoregulatory plateau is not horizontal but upward sloping. The slope of this part of the curve defines the degree of disturbance in the autoregulation (Fig. 2.10) [141]. Although the limits of the autoregulatory plateau have not been defined with certainty, the range in the term infant is 25–50 mmHg (mean arterial pressure), whereas in the preterm infant, it is less and narrower. Similarly, postnatal age and other factors affect the range and the limits of autoregulation [111]. To complicate matters, metabolic abnormalities common in the neonate (e.g., hypoxia, hypercarbia) impact on cerebral autoregulation [139, 142–144]. For example, the series of reports by Lou documented reduced cerebral blood flow in the neonate (i.e., 20 mL/100 g/min) and, in various clinical settings, cerebral flow changed in response to blood pressure (i.e., disturbed autoregulation) [139, 143].


Fig. 2.10
Theoretical concept of autoregulation in the neonate. The flat section of the heavy line represents intact autoregulation, where cerebral blood flow changes minimally over a range of arterial blood pressure. Below the knee of this curve, cerebral blood flow decreases as blood pressure decreases. Similarly, above the flat part of the curve, cerebral blood flow increases as blood pressure increases. Greisen suggests that the flat part of the autoregulatory plateau may never be horizontal and the shape of the curve may change in various clinical scenarios. The degree of the tilt may be the critical clinical factor. He suggests that autoregulation should not be simply considered “present” or “absent” but instead should be approached as quantifiable (from Greisen [141], Fig. 6, p. 213)
In the immature human (spontaneously breathing or after 48 h in the mechanically ventilated), with intact autoregulation, the response of cerebral blood flow to changes in carbon dioxide (4 % change for each 1 mmHg in PaCO2) is more robust than to changes in blood pressure (1 % change for each 1 mmHg in pressure) [145, 146]. However, such responses are not predictable when autoregulation is markedly impaired, such as in severely asphyxiated infants [147], in response to seizures [148], and, to a less degree, in mechanically ventilated preterm infants especially in the first day of life [149, 150]. Thus, although impossible to accurately predict and although responses to changes in PaCO2 vary with gestational and postnatal age, neurologic injury, systemic illness, and metabolic derangement, both hypo- and hypercarbia should be considered to have potential dramatic effects on cerebral blood flow [151]. In fact, in the neonate, both hypercarbia and hypocarbia have been associated with severe neurologic insults [152, 153]. In addition, hypercarbia has been shown to directly inhibit cerebral autoregulation in preterm infants (500–1,500 g) [152]. Similarly, within various developmentally distinct ranges, both hypoxemia and hypoglycemia directly increase cerebral blood flow [154].
Thus, common therapeutic maneuvers (mechanical ventilation, airway suctioning), metabolic derangements (hypoglycemia, hypercarbia, hypocarbia, hypoxia, hypo-/hypernatremia, and hypocalcemia), presence of a patent ductus arteriosus, and other insults (seizures, sepsis) may dramatically affect the neonate’s ability to compensate for derangements in either hemodynamic or cerebrovascular status.
Monitoring Cerebral Blood Flow
Near-Infrared Spectroscopy (NIRS):
NIRS is a noninvasive monitor that measures regional cerebral oxygen saturation. In preterm neonates (<32 weeks gestation) and term neonates, cerebral oxygen saturation is stable, reliable, and relatively unchanged among regions of the brain, although the measurements varied up to 18 % [155].
No bedside technique allows continuous monitoring of cerebral blood flow, and a “normal” blood pressure does not reliably reflect adequate cerebral perfusion (Fig. 2.7) (see Section “Cardiovascular Function”) [79, 156]. Recently, some have proposed that NIRS reflects cerebral oxygenation and hemodynamics [157, 158]. NIRS takes advantage of the difference in absorption of infrared light by oxygenated and deoxygenated hemoglobin (HbD) to derive changes in cerebral blood flow. However, cerebral blood volume, systemic oxygen saturation, and cerebral metabolic rate also affect HbD. Thus, although in many cases, the difference in HbD trends with changes in cerebral blood flow, other derived parameters [tissue oxygenation index (TOI), regional cerebral oxygenation saturation (rScO2), and cerebral fractional tissue oxygen extraction (cFTOE)] have been proposed to increase the accuracy of NIRS as a measure of cerebral blood flow.
Alternatively, several experts have combined continuous, simultaneous arterial pressure and NIRS data to quantify “cerebral pressure passivity” [159]. The loss of autoregulation was defined when changes in HbD correlated directly with changes in blood pressure. In this study of infants <1,500 g between the first 12 h after birth and day 5 of life, cerebral perfusion was pressure passive on average 20 % of the time, but up to 50 % of the time in some ELBW infants. Similarly, hypotension was most common (exceeding 80 % in some) in the ELBW. Because the monitoring was continuous, the authors documented the fluctuant nature of the pressure-passive cerebral flow, noting that arterial hypotension was not invariably associated with a pressure-passive state (and vice versa). That is, blood pressure and cerebral pressure passivity were not consistently related; the absolute value of blood pressure did not predict passivity. In contrast, others suggest a relationship between hypotension and loss of autoregulation [160]. Although cerebral pressure passivity did not correlate with the incidence of germinal matrix and/or intraventricular hemorrhage (GM-IVH), a subsequent study incorporated a complicated “coherence and transfer functional analysis” of HbD data that demonstrated a correlation between “high MAP-HbD gain” and the incidence of GM-IVH [161]. Using a sophisticated system of interrelating blood pressure and cerebral pressure passivity, the investigators generated a measure of the magnitude of pressure passivity that predicted GM-IVH (imaged on cranial ultrasound).
Thus, although this monitor has advanced the process of monitoring cerebral perfusion, NIRS currently is appropriately labeled “semiquantitative” and has been recognized as a trend monitor, but has not yet achieved a status of a “robust quantitative variable” [162]. For example, since movement artifacts interfere with continuous, long-term measurement of HbD, other analyses such as TOI or rScO2 or other complicated derivations such as those of O’Leary [161] may be more reliable [163, 164]. Most agree that a reliable, easy-to-use, portable monitor of cerebral blood flow would contribute to rational care both in the intensive care nursery and the operating room.
Cerebral White Matter Injury
Periventricular leukomalacia (PVL) can have focal (cystic) and non-cystic components; [112] the incidence correlates inversely with gestational age. The focal lesion typically develops in the deep, periventricular white matter, consists of macroscopic necrosis, eventually evolves into cysts that are easily imaged on cranial ultrasound, and in the last decade, only develops in <5 % of VLBW infants. The diffuse, non-cystic component of PVL is generally identified in the more central white matter; preferentially involves the pre-oligodendrocytes; includes areas of astrogliosis and microgliosis; consists of focal, microscopic necrosis; is not readily identified on cranial ultrasound; and currently accounts for most of the cerebral white matter injury of the preterm brain [112, 165, 166]. With advanced MRI imaging techniques, white matter injury has been identified in as many as 50 % of ELBW infants [165]. Furthermore, the incidence of diffuse white matter abnormalities increases with postnatal age, for example, from 21 %, to 53 %, and 79 % between the first postnatal week and term equivalent [167].
Although white matter injury has been identified in premature infants for decades, associated gray matter developmental abnormalities have been increasingly recognized only over the last decade as quantitative MRI techniques have evolved. Volumetric analyses have documented neuronal/axonal disease most commonly in the thalamus, basal ganglia, cerebral cortex, and cerebellum. These lesions appear on MRIs performed as early as term–equivalent and persist to adulthood [91, 118]. Although the focal, cystic, necrotic lesions of PVL seem to correlate with motor deficits, only a more extensive insult can account for the widespread neurologic insults of the ex-ELBW infant. The non-cystic component of white matter injury of PVL may account for the cognitive deficits of ex-premature infants, although the attention deficits and behavior/socialization abnormalities are more likely related to the associated axonal/gray matter injury. Volpe emphasizes that the ultimate clinical outcome from this “panencephalopathy of prematurity” (i.e., white and gray matter injury) evolves not only from the primary injury (e.g., white matter injury) but possibly more importantly from the profound abnormalities in subsequent development [93, 94].
The vulnerability of the immature brain to white matter injury is attributed to a combination of insults: ischemia/disturbed autoregulation and/or infection/inflammation. The border/end zones of the immature cerebral circulation, with incomplete development of both long and short penetrators into the white matter, are inherently at risk for ischemia [168]. In fact, the deep focal necrotic lesions of PVL are identified in the end zones of the long penetrating vessels from the middle cerebral artery. In addition, the extremely low blood flow in the white matter especially in the first 1–2 days of life adds to the vulnerability [169]. Global cerebral blood flow is ~15 mL/100 g/min (compared with the human adult, 45–50 mL/100 g/min). Of importance, flow to white and gray matter can vary significantly. In one study, blood flow to white matter was only 17 % of that to the basal ganglia/thalamus [169]. Specifically, blood flow to the white matter is ~25 % of that to the cortex, ranging between 1.6 and 3.0 mL/100 g/min [112]. In the newborn puppy, the decrease in cerebral blood flow as a result of hypotension differed among various regions of the brain, but the white matter was most vulnerable [170].
In addition to the substantial incidence of hypotension in the premature infant in the setting of the transitional circulation during the first 48 h after birth, disturbed autoregulation (see Section “Autoregulation of Cerebral Blood Flow”) augments the risk for injury from either over- or underperfusion.
Although hypoxia and/or ischemia stimulates the release of proinflammatory cytokines, a robust relationship between infection (e.g., production of cytokines/free radicals) and PVL remains elusive. Nonetheless, a clear-cut maturation-dependent susceptibility of pre-oligodendrocytes to free radical injury (both reactive oxygen species and reactive nitrogen species) in the presence of iron and decreased antioxidant mechanisms has been documented (see Section, Vulnerable Cell Populations: the Preterm Infant) [112]. Thus, the development of PVL seems to be multifactorial, involving complex interactions of immature cardiovascular, neurologic, and immunologic (and other) factors often in the presence of infection (e.g., neonatal sepsis) or chronic inflammation (e.g., chorioamnionitis).
Germinal Matrix-Intraventricular Hemorrhage
The etiologies of intracranial hemorrhage in the term infant generally include trauma, coagulation abnormalities, anatomic anomalies (aneurysm, arteriovenous malformation), and perinatal asphyxia. However, the most common intraventricular hemorrhage, the germinal matrix-intraventricular hemorrhage (GM-IVH), predominantly occurs in the preterm infant, with incidence and severity increasing with decreasing gestational age.
GM-IVH has been divided into categories that correlate with the severity and extent of the initial injury and with the clinical outcome. GM-IVH has been classified based on data from computerized tomography [171]. Grade I is a subependymal hemorrhage with minimal or no IVH (i.e., restricted to the germinal matrix). Grade II is an IVH into a lateral ventricle without distention. Grade III is IVH with enlargement of the ventricles by intraventricular blood. In that classification, a Grade IV hemorrhage includes ventricular dilatation and hemorrhage into the parenchyma of the brain.
This grading system was revised after correlating the severity of the GM-IVH with the severity of hemorrhage in the lateral ventricle on the parasagittal cranial ultrasound. In Grade I, the hemorrhage is limited to the germinal matrix, and ventricular enlargement is less than 10 %. In Grade II, 10–50 % of the ventricle is filled with blood. In Grade III, more than 50 % of the ventricle is involved, usually with enlargement of the lateral ventricles. Grade IV was eliminated, and, instead, an echo density of the periventricular parenchyma secondary to hemorrhage, a periventricular hemorrhagic infarction (PVHI), was included [172] to distinguish the lesion from GM-IVH. Thus, intraparenchymal lesions were labeled Grade IV GM-IVH, hypothesizing that the IVH had extended from the ventricle laterally into the white matter. The latter classification emphasizes that the pathophysiology of PVHI is a hemorrhagic venous infarction (see below), not simply a massive GM-IVH. This classification further stratifies PVHI according to severity based on three factors: size (localized vs. extensive), unilateral versus bilateral, and evidence of midline shift [172, 173].
GM-IVH has an overall incidence of 7–23 % [174] but may be as great as 30 % (750–1,000 g) to 40 % (501–750 g) in the ELBW infants [5]. In this same large cohort of the NICHD Neonatal Research Network, the incidence of severe IVH [Grade III and (PVHI)] (in 2000–2002) was 16 % and 24 %, respectively. In a small cohort, the overall incidence of GM-IVH decreased from 42 % in two earlier groups (1982–1989 and 1990–1999) to 22 % in the time frame (2000–2002). The incidence of severe GM-IVH also decreased from 15 to 2 % [175].
Although mortality is substantial in infants with PVHI (~50 %), it is not increased in those with Grades I–II bleeds [176]. Even though less significant neurodevelopmental delay occurs in near-term infants with uncomplicated Grade I or II IVH, they are reported to have reduced developmental functioning [173], and gray matter volumes are 16 % less than predicted based on MRI [177]. While Grades I and II IVH are not associated with severe neurologic sequelae, PVHI can be a devastating lesion. In a recent report, two-thirds of survivors of PVHI had motor delays, and one-half had cognitive impairment, with visual field abnormalities in one-third and epilepsy in 20 %. Ninety percent of PVHI have poor neurodevelopmental outcomes [178]. Furthermore, posthemorrhagic hydrocephalus (another complication of IVH) severe enough to require a ventricular-peritoneal shunt has the highest incidence of severe neurocognitive impairment in early childhood (78 % in the group with Grade III lesions and 92 % in those with a PVHI) [174].
The pathophysiology of GM-IVH is, at least in part, related to the structure of the immature brain. The specific vulnerability of the immature neurovasculature to GM-IVH correlates with the delayed development of the venous drainage compared with the arterial system. The immature veins are thin walled and their branching pattern predisposed to collapse. Because of underdevelopment of the superficial veins, most cerebral venous drainage depends on the deep venous system that drains the germinal matrix and most of the white matter.
The anatomy of the germinal matrix, the site of bleeding in this lesion (usually between the caudate nucleus and the thalamus at the level of the foramen of Monro) [172], also contributes to the greater risk for hemorrhage in the premature. Proliferating ventricular and subventricular areas of the germinal matrix produce neuroblasts (cerebral precursors for both gray and white matter) and glioblasts between 10 and 20 weeks that are densely cellular and vascularized, but gelatinous, providing poor support for the vascular network. The gelatinous nature of the area gradually decreases with increasing age, being barely present in term infants. This dense vascular network arises from the middle and anterior cerebral and anterior choroidal arteries that enter into a bed of immature, large, irregular capillary-like vessels and eventually drain into the deep venous system from the brain. The veins become the terminal vein, which makes a “U-turn” near the head of the caudate nucleus to join the vein of Galen. Thus, the “terminal vein” courses within the germinal matrix and makes an abrupt change in course at the common site of GM-IVH. Because of this unique anatomy, a large GM-IVH may obstruct the terminal vein, leading to venous distension, ischemia, and rupture, producing a PVHI. Thus, PVHI either accompanies or follows but never precedes a large GM-IVH, and, if the GM-IVH is bilateral, the PVHI is always ipsilateral to the side of the larger hemorrhage [172].
The primary site of bleeding in the premature brain is the junction of the veins and capillaries, rather than at the junction of the arteries/arterioles and capillaries. Between 24 and 28 weeks gestation, the germinal matrix is most prominent at the body of the caudate nucleus and between 28 and 32 weeks resides at the level of the head of the caudate, involuting by ~36 weeks gestation [172, 179]. When hemorrhage extends from the germinal matrix into the ventricles, blood spreads throughout the ventricular system possibly causing arachnoiditis and obstructive hydrocephalus. The free iron that is released from hemoglobin may produce hydroxyl free radicals (Fenton reaction, see Section, Age-Related Patterns of Injury) [125], in addition to other free radicals that are generated during hypoxic-ischemic injury. Ideally, antioxidant systems (e.g., superoxide dismutase; glutathione peroxidase; catalase; vitamins A, C, and E; beta carotenes; glutathione) are robust and redundant to effectively scavenge free radicals. However, when excess free radicals are generated, stores of antioxidants are depleted. In that setting, the downstream effect is cell membrane damage, increased intracellular calcium, and eventually cell death. The neonatal brain encounters increased risk for injury secondary to the combination of high oxygen consumption coupled with a reduced concentration of antioxidant systems [180]. Coincident with the often-tumultuous events characterizing the transitional circulation including the high incidence of hemodynamic instability over the first few days of life, 50 % of GM-IVHs are diagnosed by day 1 and 90 % on day 4 [179]. In fact, the high incidence of pressure-passive cerebral blood flow correlates with the presence of GM-IVH (see Section “Autoregulation of Cerebral Blood Flow”) [161]. In the presence of pressure-passive flow and because both the periventricular white matter and the germinal matrix lie within arterial end zones, both areas are at high risk for ischemia during periods of decreased cerebral perfusion and for rupture during periods of increased flow. Reperfusion after ischemia may contribute to excitotoxic injury [181].
Thus, in the presence of disturbed autoregulation, noxious stimuli (e.g., airway suctioning, painful procedures such as surgery), a rapid delivery of intravenous fluids, metabolic disturbances (e.g., hypoglycemia), and hypercarbia [144] may precipitate an acute increase in arterial and/or venous pressures that may contribute to a GM-ICH. In the opposite direction, hypotension from a variety of insults (e.g., asphyxia, anesthetic agents, sepsis, hypocarbia) may cause alternating cycles of decreased cerebral blood flow and reperfusion. Positive pressure ventilation or pneumothorax can abruptly increase central venous pressure, impeding cerebral venous drainage, possibly increasing the risk for venous hemorrhage.
Thus, although PVL (e.g., nonhemorrhagic, symmetrical) and GM-IVH (hemorrhagic, nonsymmetrical) are characterized by distinct pathophysiology, their underlying etiologies overlap and are related to the combination of immature cardio- and cerebrovascular function in the presence of vulnerable anatomy and age-specific metabolic requirements. Not surprising, PVL and GM-IVH are commonly identified on the same MRI.
Cerebellar Injury
In addition to the pervasive neuronal/axonal disturbances involving the cerebral cortex, thalamus, and basal ganglia of newborns secondary to PVL and GM-ICH, the cerebellum has recently been identified as a very vulnerable site for injury. “From 24 weeks to 40 weeks of gestation, the cerebellum undergoes a rate of growth nearly unparalleled elsewhere in the brain” (Fig. 2.9). The surface area of the cerebellar cortex increases more than 30-fold during this time period [103]. Of particular relevance to the preterm infant, cerebellar development accelerates between 20 and 30 weeks of gestation.
As with other neurologic injuries in the neonate, the incidence of insults to the cerebellum is inversely related to gestational age and can be attributed to two etiologies: destructive (hemorrhage/infarction) and impaired development. As in the case of GM-IVH, cerebellar hemorrhage is usually unilateral, with 77 % associated with supratentorial lesions (mostly white matter injury) [103]. Impaired cerebrovascular autoregulation predictably contributes to the pathogenesis. For example, in a group of ex-ELBW infants with cerebral palsy, 64 % (32/50 patients) had parenchymal cerebellar loss which coexisted with cystic PVL and/or cerebral white matter loss, suggesting a common etiology (i.e., ischemia, infection/inflammation) [182]. Destructive lesions can be confined to the cerebellum, and infants can be asymptomatic until delayed development is noted months to years after birth [183]. In those with severe destructive injuries, the impact on function can be significant, including spastic-ataxic-dyskinetic cerebral palsy, severe cognitive deficits, microcephaly, and epilepsy [184].
In other cases, MRIs reveal cerebellar underdevelopment (unilateral or bilateral symmetric deficits in cerebellar volumes) without destructive lesions [185] but in association with supratentorial lesions (i.e., PVL and/or GM-IVH). Injury to granular cells (e.g., secondary to injury from free iron after hemorrhage) [185] is significant not only because of decreasing this population of cells but also because of disturbing the excitatory input to other cells (e.g., Purkinje cells) that disrupts development of the intricate cerebellar circuitry [103]. The association of cerebellar underdevelopment with supratentorial lesions suggests the role of “multiple remote tropic transneuronal interactions” [103]. For example, unilateral PVHI may be associated not only with an expected loss of ipsilateral cerebral volume but also with loss of contralateral cerebellar volume; similarly, unilateral cerebellar hemorrhage is associated not only with decreased ipsilateral cerebellar volume but also with decreased contralateral cerebral volumes. Bilateral primary injury in the cerebrum is associated with secondary bilateral injury in the cerebellum and vice versa. Volpe suggests, “an intact reverberating circuit between cerebrum and cerebellum and vice versa may be especially critical for normal growth during this critical phase of development” [103]. In those with impaired cerebellar development, long-term outcome includes deficits in executive, visual-spatial functions, and language [186]. Others have disturbances of language and socialization [187].
Thus, cerebellar injury is associated with significant neuromotor and intellectual deficits, as well as learning, language. and social behavior deficits. That is, cerebellar injury can result in cognitive and affective disturbances, including socialization difficulties and some positive autism findings [188]. Finally, a “cognitive-affective disorder” (executive, visual-spatial, linguistic, affective deficits) has been described in older children and adults with cerebellar injury [189]. Thus, abnormalities in cerebellar developmental may cause cognitive, language, and socio-affective abnormalities in the ex-premature infant.
Clinical Significance and Summary
Many characteristics of the immature neurologic system predispose the neonate to injury in the predictably labile intraoperative period. Disturbed autoregulation combined with vulnerable populations of cells in the setting of labile hemodynamic and respiratory status in the perinatal period without a reliable system for monitoring cerebral perfusion demands that the neonatal anesthesiologist focus on defining “normal” preoperatively for each infant and attempting to maintain that status intraoperatively. Although easy to recommend, avoiding wide fluctuations in blood pressure, PaCO2, and PaO2 is frequently difficult but critical to achieve, especially in the intraoperative setting when cardiorespiratory instability is common. Hemorrhage often requires rapid infusions of crystalloid and colloid, which can clearly inflict neurologic injury. NIRS may be of value as a trend monitor, but artifacts are notoriously common.
The Pulmonary System
The transition from fetal to postnatal life requires dramatic adaptation in the physiology of respiration from complete dependency on the placenta to gas exchange via air-filled, perfused lungs within seconds after birth. For premature or asphyxiated infants or in the setting of anomalies associated with cardiorespiratory dysfunction (e.g., congenital diaphragmatic hernia, tracheoesophageal fistula, some types of congenital heart disease), the superimposed effects of surgery, inhalational anesthetics and other medications, positive pressure ventilation, and infection add to the critical short-term challenge of maintaining physiologic stability while minimizing long-term morbidity.
Embryology
The development of the pulmonary system has been divided into five stages (embryonic, pseudoglandular, canalicular, saccular, alveolar) based on morphology [190] (Fig. 2.11). Knowledge of the sequence of developmental events can inform estimates of the timing of congenital malformations [192] associated with fetal-maternal factors (e.g., oligohydramnios), genetic factors, or developmental insults [191].


Fig. 2.11
Stages of normal lung development with the airway structure developing within each stage (from Kotecha [191])
The Embryonic Stage (0–7 Weeks Gestational Age):
At 3–4 weeks gestational age, the laryngotracheal grove first appears as a ventral diverticulum from the primitive foregut, lined by epithelial cells of endodermal origin [192]. During the embryonic stage, the large airways first appear as epithelial cells from the foregut that eventually invade the mesenchyme to form the trachea. This structure then undergoes a series of branching events [193], requiring interaction of epithelial and mesenchymal cells [194]. By the fifth week of gestation, the branching has advanced to the level of lobar and segmental bronchi [193], so that five pulmonary lobes have been formed. By the end of the embryonic stage, the 18 major lobules are easily recognized [195]. Although the airway epithelium resembles that of the esophagus at this stage, over the course of development, differentiation and maturation of the primitive endodermal cells produce the population of epithelial cells that characterize the adult lung [193]. During the embryonic stage, the pulmonary vasculature develops in parallel with the airways. At 4 weeks gestational age, endothelial cell precursors around the developing lung bud eventually form endothelial tubes that continuously coalesce to form the intrapulmonary arteries [196]. Congenital malformations associated with events during the embryonic stage involve the large airways and/or whole sections of lung and include pulmonary agenesis, ectopic lobes, lobar cysts, agenesis, malformation, stenosis, or malacia and vascular malformations [192].
Pseudoglandular Stage (7–17 Weeks Gestational Age):
The most rapid branching of the airways occurs during the pseudoglandular stage. As the epithelial cells divide, the surrounding mass of gland-like mesenchyme [191, 193] regulates the branching. The mesenchyme inhibits branching in the trachea but induces branching in bronchi [197]. By 14 weeks gestational age, 70 % of the airways present at birth have formed, and by 17 weeks, the conducting airways, terminal bronchioles, and primitive acini are completely established [191]. In parallel with the development of the airways during the pseudoglandular stage, the vascular structures branch rapidly leading to the formation of the pulmonary arteries and veins, which, along with the airways are derived from mesenchymal tissue [192, 196]. Further differentiation of the pseudostratified epithelium of the airway involves its progressive replacement with columnar cells proximally and cuboidal cells distally. Between 11 and 16 weeks gestation, ciliated epithelium appears and airway mucus is first synthesized [193]. The cuboidal cells eventually mature into type II pneumocytes. Insults during this stage of lung growth can alter bronchial growth patterns, producing lesions characterized by poor lung growth (pulmonary hypoplasia), sequestration lesions, and cystic adenomatoid malformations [192].
During this critical period of lung growth, the musculotendinous, dome-shaped diaphragm develops, which, in addition to becoming the primary muscle of respiration, serves to separate the pleural and peritoneal cavities and promote pulmonary growth [198]. At the end of the third week of gestation, the diaphragm consists of a collection of mesodermal tissue, the septum transversum, which separates the pleural-pericardial cavity from the peritoneal cavity. Of note, pleural-peritoneal canals allow limited but persistent communication between these two cavities [198, 199]. The septum transversum migrates downward from the level of the occipital and upper cervical somites (C3) to the level of the thoracic somites by the sixth week of gestation and to the level of L1 by the eighth week [198]. During the descent, the neural tissue of C3–C5 origin penetrates the mesoderm and eventually develops into the phrenic nerve. At approximately this time, the right and left pleuroperitoneal membranes close the communication between pleural and peritoneal cavities [198]. As elucidated in murine models, muscle precursor cells migrate laterally to form the lip of the primitive diaphragm and then radiate throughout the developing diaphragm accompanied by new phrenic nerve branches [199].
Congenital diaphragmatic hernia (CDH) results from failure of complete separation of the pleural and peritoneal cavities. At 10–12 weeks gestation, before the bowel returns to the abdominal cavity from the amnion (where it resides in early gestation), closure of diaphragm is complete. If the separation of the two body cavities is incomplete, the bowel enters the chest, the path of least resistance. However, bowel then occupies space needed for lung growth. A posterolateral CDH (Bochdalek hernia) results from failure of closure of the pleural-peritoneal membranes, accounts for 95 % of diaphragmatic hernias (approximately 1/2,000–1/4,000 live births) [200, 201], and is usually unilateral (78 % on left, 20 % on right, 2 % bilateral) [200] but often is associated with significant ipsilateral pulmonary hypoplasia as well as abnormal development of the contralateral lung [202]. In addition to abnormal lung development, other anomalies not directly related to the hernia often accompany this lesion (e.g., congenital heart disease, central nervous system anomalies) [200]. The etiology of CDH is not completely understood, but genetic associations have been noted (e.g., chromosomal aneuploidy) [203] (for a review, see [204]).
Intrauterine exposure to teratogens affecting the retinoic acid enzyme pathway induces CDH through malformation of the primordial nonmuscular diaphragmatic tissue as early as the fifth to seventh weeks of gestation via a cascade of events that leads to failure of closure of the posterolateral walls in later gestation [199, 205]. Of note, experiments in transgenic mice have induced absence of lung development without CDH, implying that CDH is a primary event [206, 207].
Canalicular Stage (17–27 Weeks):
During the canalicular stage, the distal airways develop into primary acini, consisting of respiratory bronchioles, alveolar ducts, and rudimentary alveoli. The surrounding capillaries develop in parallel [195]. These represent the first units of gas exchange.
Epithelial cells differentiate into types I and II pneumocytes, with type I cells incorporated into the first alveolar-capillary barrier. Surfactant can be detected at approximately 24 weeks with active production beginning at 26–28 weeks. After 26 weeks gestation, respiratory saccules lie in close contact with pulmonary capillaries, increasing the likelihood of adequate gas exchange essential for extrauterine viability. Before this developmental age, gas exchange may be compromised because of inadequate surface area and function of the lung parenchyma and/or vasculature [190]. For example, survival after birth during the canalicular stage, in part, is determined by surfactant deficiency (respiratory distress syndrome) (see Respiratory Distress Syndrome, below). Although delivery of exogenous surfactant can improve neonatal lung function, subsequent insults associated with supportive care of the premature infant (e.g., supplemental oxygen, mechanical ventilation, infection) often result in pulmonary hypoplasia or acinar dysplasia [192] and later bronchopulmonary dysplasia.
Saccular Phase (28–36 Weeks Gestation):
An increase in the surface area of gas exchange is the major feature of the saccular phase of lung growth [192]. The peripheral areas of the lung enlarge, as the acini dilate and the acinar walls thin. Gas exchange is further facilitated as type II pneumocytes increasingly differentiate into type I cells and capillaries develop in close approximation [195].
Alveolar Phase (36 Weeks Gestation Until ~2–3 Years):
During the alveolar phase, the surface area for gas exchange increases as alveoli septate and increase in number, a process that continues through the third year of life [191, 196]. Type II pneumocytes proliferate and become prominent after 34–36 weeks of gestation. The key feature of type II pneumocytes is eosinophilic lamellar bodies, specialized vesicles that store and release surfactant lipids and proteins [208]. At term, the total number of alveoli in the healthy infant is only 20–50 million [195], increasing to adult numbers of more than 300 million per lung, by 2–3 years of age [209, 210]. Developmental abnormalities during the alveolar phase can result in respiratory distress syndrome, chronic lung disease (see below), and dysplasia of the acini or alveolar capillaries [192]. Although rare, several genetic disorders can also adversely affect lung development, such as mutations in the surfactant protein system [195].
The molecular basis of the control of lung development is incompletely understood, but involves many transcription and growth factors in critical roles [192]. In addition, the surrounding mesenchyme appears to direct the developing epithelial cells, and the mesenchymal-epithelium interactions seem to be essential for normal development [195]. A variety of mechanical factors affect lung growth in utero and postnatally [192]. For example, in animal models, inadequate fetal lung fluid secondary to a deficiency of amniotic fluid can induce pulmonary hypoplasia [211, 212]. In humans, pulmonary hypoplasia is prominent in the oligohydramnios sequence (“Potter’s syndrome”) associated with low urine output secondary to renal dysgenesis [213]. Similarly, oligohydramnios secondary to chronic leakage of amniotic fluid can interfere with lung development [214]. Finally, the role of maintaining adequate lung volume with fluid in promoting lung growth and development during the canalicular and saccular stages is the basis of various fetal therapeutic interventions (e.g., in utero tracheal occlusion) intended to induce lung growth in the presence of CDH [192, 215].
Fetal breathing may contribute to maintaining sufficient lung volume and, therefore, growth of the lung, possibly by activating stretch-mediated release of growth factors [192]. When fetal breathing is ablated in animals, lung growth decreases [216]. Decreased fetal breathing movements may underlie the pulmonary hypoplasia associated with some neurologic disorders, abdominal wall defects, and in utero exposure to certain substances (e.g., chronic exposure to diazepam and possibly maternal smoking) [217]. Although lesions occupying the intrathoracic space (e.g., CDH, congenital cystic adenoid malformations) and skeletal defects involving the thorax can impede lung development secondary to obvious mechanical effects, these anomalies may also impair fetal breathing, which may exacerbate the primary effect of the space-occupying lesion [217].
Postnatal Development of the Lung
Postnatal development of the lung includes the completion of the alveolar stage [218] to achieve airway and microvascular maturation (birth to 2–3 years). That is, alveolar-capillary microarchitecture is transformed from double to single capillary networks [219]. Postnatal therapy with corticosteroids may impair lung growth by arresting this process [192]. Beyond the first two years of life and until late adolescence, the lungs continue to grow via increase in size of both bronchioles and alveoli [220]. Whether late alveolarization continues after the toddler years (and beyond), and by what mechanism, remains controversial [219].
Premature birth and/or infection can severely impact growth of the lung, especially alveolarization [191]. For example, in utero infection (e.g., chorioamnionitis) and markers of lung inflammation have been associated with an increased incidence of chronic lung disease characterized by poor lung development [221, 222]. In addition, the lifesaving supportive therapies (e.g., mechanical ventilation, supplemental oxygen) commonly delivered to the premature infant have been reported to impair normal lung development, resulting in abnormal alveolarization [223]. Specifically, oxidative stress has also been implicated in disorders of lung growth (see Oxygen Therapy, below). Other physiologic events associated with prematurity, such as patent ductus arteriosus and immune dysfunction, are also associated with growth disorders [191]. Similar to the close relationship of the parenchyma and vasculature during normal development, the pulmonary vasculature is co-injured secondary to prematurity and associated treatment. For example, preterm infants with chronic lung disease have increased pulmonary arterial and venous smooth muscle, and infants born before 27 weeks gestation age often develop hypoplasia and dysplasia of the alveolar capillaries [196]. Thus, research in neonatal clinical care is currently focused on minimizing the long-term morbidity of lung injury while providing critical therapies, such as mechanical ventilation and supplemental oxygen [224].
Poor nutrition and environmental exposures such as maternal smoking during pregnancy have been associated with generalized poor growth and risk for prematurity. In addition, maternal smoking has been linked to long-standing respiratory disorders during infancy. These respiratory problems may improve in later childhood, but also are likely to persist into adulthood, becoming apparent as normal aging decreases lung function [225–227]. Both prenatal and postnatal secondhand smoke exposures are linked to structural changes in the developing lung, with altered airway geometry and size, increased wheezing with respiratory infections, as well as variable decreases in forced expiratory flows, possible increases in airway responsiveness and bronchospasm, and increased airway resistance (see [225] for a review).
Airway Anatomy
Neonatal airway anatomy differs from that of adult with significant implications for the anesthesiologist (see Chap. 5, Airway Management). With a relatively large head and prominent occiput, both flexion and hyperextension of the neck may obstruct the small, compliant airway (i.e., easily compressible) [228]. Because of the small diameter of the airways, flow may encounter greater resistance based on the presence of turbulent flow in the upper airways (R ∝ 1/r 5) and laminar flow in the lower airways (beyond the fifth bronchial division) according to Poiseuille’s law: R ∝ (ηL)/r 4, where R is airway resistance to flow; η is viscosity; L is length; and r is airway radius. That is, airway resistance varies inversely in proportion to the radius of the airway to the fifth or fourth power in different sections of the tracheobronchial tree. Notably, in the cricoid ring region of the upper airway, a 50 % decrease in the radius of the airway (e.g., airway edema with infection or trauma) increases the airway resistance and the work of breathing to the fifth power or by 36-fold.
Infants have traditionally been described as “obligate nasal breathers” because they often have difficulty maintaining adequate ventilation when the nasal passageways are obstructed secondary to congenital anomalies (e.g., bilateral choanal atresia), with inflammation or infection of the airways, which produce mucosal edema or secretions, and after certain therapeutic maneuvers (e.g., placement of an NG-tube). An alternative descriptor, “preferential nasal breathers,” has been proposed, since many neonates with obstructed nasal passages can transition to oral breathing, a process involving activation of the levator veli palatini and musculus uvulae [229]. Nonetheless, in the setting of nasal airway obstruction, oxygen desaturation may occur, and—even after a successful transition to mouth-breathing—respiratory failure due to fatigue may follow [230].
The laryngeal structures of the neonate differ from those of children and adults. Based on observations of castings of cadaveric airways, the shape of the newborn larynx has been traditionally referred to as “funnel shaped,” becoming more cylindrical (i.e., adultlike) with growth. However, recent reports of in vivo imaging have noted that the neonatal larynx is in fact cylindrical, similar to those of adults [231–233]. In sedated infants, the glottic opening appears to be the narrowest part of the juvenile upper airway, but the cricoid remains functionally the narrowest part of the airway. The confusion may be related to the failure to gate the MRI to respiratory phase when the glottis was filmed. While the rigid ring of cricoid cartilage is unyielding, the vocal cords can be opened gently, from the at-rest position, to accommodate rigid structures, like a tracheal tube [231]. In the neonate, the anterior larynx is more caudal than the posterior larynx, resulting in an elliptical shape, more narrow in the transverse plane than in the anterior–posterior dimension [232, 233]. Forcing a tight-fitting, circular tracheal tube through the subglottis can lead to excessive pressure in the anterior–posterior axis, resulting in mucosal ischemia, subglottic edema, short- or long-term stridor, and airway scarring or stenosis [231].
Other characteristics of the neonatal airway must be considered during endotracheal intubation [234]. The neonate’s neck is relatively short and the larynx located more cephalad (C3–C4) (of note, the larynx is more superior, not more “anterior”) compared with that in the adult (C4–C6). The narrow epiglottis may be omega or U-shaped and difficult to elevate directly with a laryngoscope blade. The relatively large tongue, soft submandibular structures that are prone to compression by a ventilator’s fingers, or cricoid pressure predisposes the neonate to upper airway obstruction. With less cartilage, smooth muscle, and contractile elements, the trachea is more compliant but with greater resistance to flow [235]. Because of a lack of development of the fascia that stabilizes the neck and immaturity of control of the upper airway muscles that stabilize the pharynx, the upper airway of the premature is especially prone to collapse [236].
As in the case of the upper airway, the lower airways are very compliant and readily collapsible, decreasing peak flows and increasing airway resistance and work of breathing. Wheezing may be audible but may or may not be associated with actual bronchospasm. Not surprising, since wheezing is associated with many etiologies other than bronchospasm in the neonate, response to bronchodilators is unpredictable [237].
Anatomy of Chest Wall and Mechanics of Breathing
The chest wall—consisting of the rib cage, abdomen (despite the term “chest wall”), and associated musculature—functions both as the respiratory pump and framework for the respiratory system. When compared with older children and adults, the respiratory pump is less efficient in the neonate due to anatomic, mechanical, and histologic factors [238]. The geometry of the pliable rib cage in the neonate is more horizontal compared with the angulated structure in the adult, potentially limiting the outward and cephalad expansion of the thoracic cavity during inspiration [239] (Fig. 2.12).


Fig. 2.12
Observed changes in configuration and cross-sectional shape of the thorax from infancy to early childhood. Upper panel: infantile and adult rib cage configurations. Lower panel: how rib growth at costochondral junctions and posterior rib angles could explain the observed changes in cross-sectional shape of the thorax (from Openshaw et al. [239])
The compliance (C CW) of the cartilaginous chest wall is much greater than lung compliance (C L) [240]. Although the pliable nature of the chest wall is advantageous during birth, in the postnatal period, this same characteristic introduces several disadvantages. Although C L primarily determines the compliance of the entire respiratory system (C RS) [241–243], the large C CW:C L ratio predisposes the neonate to a small resting lung volume [243]. Further, the compliant chest wall deforms inward during spontaneous inspiration, creating inefficient chest wall motion, significantly decreasing the mechanical efficiency of inspiration. That is, distortion of the chest wall wastes energy [240, 244, 245]. As the chest wall matures and stiffens (contemporaneously with the onset of the demands of upright posture), C CW decreases to approximately C L [240], perhaps mediated through mineralization of the rib cage and/or increased chest wall muscle tone, as suggested by studies in lambs [242, 246].
The respiratory muscles change in conformation, molecular biology, and function during development. During gestation, the respiratory muscles are trained by fetal breathing, so that at the time of term birth, they are ready to power respiration, although with a limited effort reserve [247]. The respiratory pump in the neonate does not tolerate a large respiratory load compared with the adult, and thus it is predisposed to failure [238, 239]. As with other muscle systems, the respiratory muscles, principally the diaphragm, can be characterized in terms of preload, afterload, and intrinsic properties of the fibers. The susceptibility of the neonatal diaphragm and intercostal muscles to respiratory fatigue often is logically attributed to the relative paucity of type I cells [248], p. 203]. That is, type I muscle cells are characterized by slow cycling, aerobic metabolism, resistance to fatigue, and seem well suited for respiratory cycling. The premature infant diaphragm may have less than 10 % type I cells; by term, the proportion increases to ~25–30 %, and through late infancy, the population of type I cells increases to the adult proportion of ~55 % [248], p. 203]. However, when other aspects of structure (e.g., myosin heavy chain isoform (MHC) expression, MHC protein content) and specific function (e.g., maximum specific force, twitch contraction times) were considered, the neonatal diaphragm was actually considered to be more resistant to fatigue [249, pp. 3159–239]. Developmental expression of distinct myosin heavy chain isoforms with less energy demands may actually protect the neonatal diaphragm from fatigue by decreasing energy demands ([249, pp. 3159–239], [250, pp. 3160–240]). Similarly, in a recent study of the diaphragm of the lamb over an extended developmental time period (mid-gestation to 2 months postnatal), the susceptibility to fatigue decreased in late fetal life but was highest at approximately 1 week after full-term delivery [251]. The susceptibility then decreased with increasing postnatal age. Of note, specific force increased twofold during the last 2 weeks before term and correlated with onset of fetal breathing movements. The improved maximum specific force correlated with the rise in MHC content. Similar to earlier studies, the increase in MHC 1 content correlated with increase in oxidative capacity. On the other hand, the in vitro changes in resistance to fatigue must be considered along with the intrinsic properties of the developing diaphragm, including decreased diaphragmatic thickness and less effective force-frequency length-tension, force-velocity functions [252, pp. 3166–241] that may increase the risk of respiratory failure [249, pp. 3159–239]. Clearly, the concept of susceptibility to diaphragmatic fatigue is complex and, in addition to developmental effects on maturation of this muscle, may be affected by the physiologic environment, including hormonal influence (e.g., cortisol, thyroid) and oxidative state (e.g., nutritional status, sepsis, acidosis). The respiratory pump may fail to produce sufficient work to maintain homeostasis, secondary to the intrinsic properties of the muscles of respiration or from unfavorable alterations in preload or afterload [253]. Conditions that affect intrinsic muscle function include metabolic derangements (e.g., electrolyte disturbances), hypoxemia, and shock [253]. Hyper-expansion of the lungs due to air trapping alters preload. Inherent inefficient chest wall mechanics (see above), increased upper airway resistance (due to airway size and propensity to collapse), and changes in lung compliance due to respiratory distress syndrome, pulmonary edema, and other lung disease increase afterload (i.e., work of breathing). The central and peripheral nervous system components that control the respiratory pump can also contribute to respiratory failure (see Control of Respiration, below). Respiratory failure in the neonate can present with respiratory distress (e.g., tachypnea, retractions, use of accessory muscles), hypercapnia/respiratory acidosis, hypoxemia, and/or apnea. In addition to appropriate interventions (e.g., supplemental oxygen, mechanical ventilatory support including continuous positive airway pressure, nutrition), methylxanthines, such as theophylline, are sometimes introduced to improve diaphragmatic function [254, 255].
Functional Residual Capacity
The quantity of gas remaining in the lungs in passive (i.e., with no activity of respiratory muscles) conditions is termed the functional residual capacity (FRC). The magnitude of the FRC results from the net effect of the competing forces of inward lung recoil and outward chest wall elasticity. In neonates, the transpulmonary (intrapleural) pressure at rest is 0 cmH2O, compared with −5 cmH2O in adults [256]. With a compliant chest wall, lung volumes at end-expiration during tidal breathing in the neonate approach FRC, creating a significant risk of small airway collapse [257], atelectasis, ventilation-perfusion mismatch, and hypoxemia. To compensate and to preserve FRC dynamically, neonates use several strategies: shortening exhalation via rapid respiratory rates [258], [259], “laryngeal braking” (auto-PEEP mediated by dynamic partial closure of the glottis) [247, 258], tonic activity of intercostal muscles to stabilize the chest wall [247], and, when lung volumes are reduced, grunting [260].
With limited respiratory reserve and a dependance on these dynamic alterations of exhalation to expand the end-expiratory volume above FRC, the neonate is at risk for ventilatory failure from conditions that increase respiratory work or impair neural control of respiration. Such conditions include general anesthesia (with or without neuromuscular blockade), REM (rapid eye movement) sleep [238, 261, 262], systemic infection, and shock. In addition to its effects on intercostal muscle function that contribute to paradoxical, inefficient respiratory movement, central nervous depression relaxes the muscles of the upper airway increasing airway resistance and respiratory work. Decreased FRC and the ensuing atelectasis inflict further demands on the diaphragm, as the reduced lung compliance increases the work of breathing, and energy is required to re-recruit alveoli [243]. Acute lung disease with decreased lung compliance, increased airway resistance (e.g., from airway edema), or decreased diaphragmatic function can exacerbate the effects of anesthesia, sleep, and various neonatal disorders (e.g., infection) and anomalies (e.g., pulmonary hypoplasia). Continuous airway distending pressure (e.g., CPAP) [263] will maintain FRC and mechanical efficiency during anesthesia and sleep in the setting of lung or other disease, including prematurity. Positive pressure ventilation can maintain and restore FRC, especially with PEEP (see Chap. 9, Neonatal Ventilation). A technique rarely used today is application of external negative extrathoracic pressure (−14 to −18 cmH2O), which increases FRC in premature neonates [264].
Pulmonary Function Testing
Some techniques for pulmonary function testing (PFT) in adults have been adapted to neonates and young infants. Equipment to measure PFTs in neonates must address the smaller tidal volumes and dead space without sacrificing precision or increasing resistance to airflow. Additional challenges include the need to sedate the infants and a paucity of normal reference data as a function of age (Table 2.1) and for various disease states. The techniques adapted to neonates and young infants include whole body plethysmography, gas dilution and occlusion techniques, esophageal manometry, weighted spirometry, and interrupter techniques (for reviews, see [265–268]). Because nasal airway resistance contributes as much as 50 % of total airway resistance, obstruction of the nasal passage by a nasogastric tube can increase airway resistance by 50 %. Therefore, during studies of pulmonary function, tubes should be placed in the smaller nostril to permit maximum flow via the larger passageway [269].
Table 2.1
Typical values of lung function in healthy individuals
Parameter | Preterm | Newborn | 1 year | 7 year | Adult |
---|---|---|---|---|---|
Body weight (kg) | 1 | 3 | 10 | 25 | 70 |
Crown-heel length (cm) | 35 | 50 | 75 | 120 | 175 |
Respiratory rate (min−1) | 60 | 45 | 30 | 20 | 15 |
Tidal volume (mL) | 7 | 21 | 70 | 180 | 500 |
Anatomic dead space (mL) | 3 | 6 | 20 | 50 | 150 |
Maximal flow at FRC (mL s−1) | 80 | 150 | 300 | – | – |
FRC (mL) | 25 | 85 | 250 | 750 | 2,100 |
Lung compliance (mL kPa−1) | 15 | 50 | 150 | 500 | 2,100 |
Airway resistance (kPa L−1 s) | 8 | 4 | 1.5 | 0.4 | 0.2 |
Specific compliance (kPa−1) | 0.6 | 0.6 | 0.6 | 0.7 | 0.8 |
Specific conductance (s–1 kPa−1) | 5 | 2.9 | 2.7 | 2.7 | 2.3 |
Static volumes and capacities [e.g., FRC and tidal volume (V t)] are generally similar to adult values when normalized for body mass. Anatomic dead space as a proportion of tidal volume is similar in the neonate and adult (i.e., about 25 % of V t). Although the additional dead space introduced by airway devices (e.g., LMA) may be negligible in the adult, it is more significant in the neonate, given their smaller anatomic dead space. Closing capacity in the infant (~35 mL/kg) can exceed FRC (~30 mL/kg), leading to airway closure.
Alveolar surface area (VA) is 2.8 m2 at birth, increasing to 75 m2 in adults. This increase is related linearly to body surface area [270]. However, since the metabolic rate and consumption of oxygen (VO2) per body mass is greater in the infant compared with the adult, the ratio of VA to VO2 is reduced, increasing the infant’s risk for impaired gas exchange, especially in the setting of underlying disorders that limit VA, such as pulmonary hypoplasia. Ventilation–perfusion (V–Q) mismatch (i.e., alveolar collapse leading to intrapulmonary shunting) commonly causes hypoxia and shunting that can be quantified by noting the difference between alveolar and arterial oxygen tension (which can also reflect diffusion failure).
V–Q mismatching is exaggerated in infants with lung disease [271]. The increased alveolar ventilation (V-dot A) in neonates (~136–168 mL/kg/min) is approximately 2–3 times that of adults (~60 mL/kg/min) [272–274] reflecting the greater metabolic demand for oxygen (VO2) (6–10 mL/kg/min vs. ~3.5 mL/kg/min for the resting adult) and greater production of CO2. Increased oxygen demand coupled with atelectasis and intrapulmonary shunting causes the rapid development of hypoxemia when apnea occurs (within ~30 s) [275], a finding with significant implications for induction of anesthesia (e.g., rapid sequence induction in the presence of a “full stomach”).
Minute ventilation increases with either a greater V t or respiratory frequency (ƒ) or both. For a given alveolar ventilation, the optimal ƒ and V t minimizes the expenditure of energy [276, 277]. Although V t per body mass in the neonate approximates that of adults, the normal respiratory rate is greater in the neonate (30–60 breaths/min) compared with the adult (18–22 breaths/min). That is, the work of breathing to achieve adequate minute ventilation in the healthy neonate is minimized at this increased respiratory rate [256, 278, 279]. Despite this strategy, the neonate (especially the premature infant) expends a larger portion of total body oxygen consumption on ventilation (i.e., the oxygen cost of breathing) than does the adult. This phenomenon is exaggerated in the setting of lung disease [280, 281].
In the neonate, increased alveolar ventilation and constant FRC compared with adults lead to a smaller FRC “buffer” [282]. However, changes in inspiratory gas composition (e.g., oxygen, anesthetic gases) will be reflected in changes in the alveolar and plasma gas concentrations more rapidly.
Pulmonary Surfactant
Endogenous pulmonary surface-active agent, or “pulmonary surfactant,” maintains lung volumes. This foamy, bubble-producing substance is a complex aggregation of macromolecules that reduces alveolar surface tension, decreases the tendency of alveolar units to become atelectatic, and increases pulmonary compliance [283, 284]. At approximately 20 weeks gestation, mechanical forces related to fetal breathing stimulate expression of genes that code for the expression of surfactant [285]. However, the quantity of surfactant is reliably sufficient to support spontaneous ventilation only after 32 weeks gestation, without medical intervention. In some premature infants (most commonly, <32 weeks gestational age), inadequate surfactant production manifests as respiratory distress syndrome (see below). Fetal lung maturity is estimated by the ratio of the lecithin to sphingomyelin surfactant components, in amniotic fluid (the L:S ratio). This ratio provides prognostic information of the neonate’s expected pulmonary status. An L:S ratio >2.0 is associated with a reduced risk of RDS, whereas a ratio <2.0 is associated with an increased risk of RDS [286]. Hereditary defects in surfactant structure or the surfactant metabolism cycle can cause fatal (e.g., hereditary SP-B deficiency) or chronic lung disease, such as protein alveolar proteinosis [208]. Surfactant impairment has been implicated in a number of lung diseases beyond the neonatal period, including the adult respiratory distress syndrome.
Type II pneumocytes serve several critical functions in alveoli including as the progenitors of type I pneumocytes (the major population of the alveolar epithelium) to repair the epithelium after injury and to produce and secrete surfactant [208]. Surfactant is stored within characteristic organelles termed lamellar bodies, which are released by merocrine secretion, eventually forming a monolayer over the alveolar surface, reducing surface tension at the alveolar air-water interface [287]. Various configurations of surfactant are observed, such as tubular myelin, droplets, and the monolayer film, each serving to support specific functions including spreading throughout the alveoli, forming a monolayer, acting as reserve surfactant when the monolayer is disrupted (e.g., by oxidation), resisting compression during exhalation, and rapid reabsorption [288]. The local environment (such as the changing physical forces during the respiratory cycle and calcium concentration) and the composition of various phospholipids and surfactant-associated proteins (SPs) that compose a particular surfactant molecule [208, 287] influence the phases of surfactant. Thus, a complex pathway of highly regulated recycling or degradation (by alveolar macrophages) has evolved to allow for the energy and substrate intensive activity of this critical molecule [208]. Approximately 85 % of the components of surfactant are recycled via reuptake into type II pneumocytes [289–292].
Surfactant is an emulsion of lipids (~90 %), proteins (~10 %), and carbohydrates [293]. Most lipids are phospholipids (PLs) including the phosphatidylcholines (PCs) such as dipalmitoylphosphatidylcholine (DPPC), which comprises 40–45 % (by mass) of mammalian surfactant [287]. A heterogeneous mixture of lipids is necessary to convey the necessary properties of rapid spreading over the alveolar (water phase) surface and resistance to compression [294]. A variety of functions have been identified for the surfactant proteins (SP-A, SP-B, SP-C, and SP-D) [295]. SP-B and SP-C are small, hydrophobic proteins that interact closely with the surfactant lipids to reduce surface tension and stabilize surfactant when exposed to the changing mechanical forces of the respiratory cycle [296]. SP-A and SP-D are larger proteins, members of the Collectins family that bind infectious particles in the lung, facilitating immune cell recognition and clearance of pulmonary pathogens. Not only are these proteins important for the innate immune system of the lung, but in mouse models, they modulate alveolar macrophage activity. SP-A and SP-D may regulate surfactant uptake, but the precise roles of these proteins in this capacity are still being elucidated [293, 297].
Surface tension as modeled as a sphere is described by Laplace’s law P = 2γ/r, where P is the intra-alveolar pressure or “collapse pressure” of the alveolus (i.e., the pressure necessary to counteract the contracting molecular forces produced at the air/fluid interface—the inward force acting to shorten the radius of the sphere, promoting collapse) [298]. This law states that the distending pressure is proportional to surface tension (γ) and inversely related the radius of the alveolus (r). During inspiration, alveolar radii generally increase due to forces generated by the respiratory pump, and therefore collapse pressure tends to decrease. At the end of expiration, significant distending pressure would be needed to prevent alveolar collapse without the effects of surfactant. In films lining the alveolus, surfactant can produce large surface pressures, decreasing the contracting force of surface tension. When alveoli are large, the surfactant monolayer is spread thinly over the alveolus, and because of the large radius, the effect of surfactant is not critical [299]. When alveoli are small (e.g., during exhalation), surfactant is compressed, further reducing surface tension in the setting of a small radius. Thus, surfactant acts to buffer surface tension over a large range of alveolar sizes.
However, alveoli do not operate in isolation and, instead, are organized into groups of acini [300]. The lung parenchyma, including the alveoli themselves, directly influences adjacent airways through traction [301]. At large lung volumes, such as those induced by positive pressure ventilation, the alveolar surface area to lung volume ratio is independent of surface tension (dependent instead on tissue interactions) [302]. In this case, 876, the beneficial effect of surfactant therapy may be masked by the artificially augmented lung volumes [287]. In the macro perspective, alveolar and airway collapse are counteracted by the outwardly acting elastic recoil of the lung and chest wall, as well as the active respiratory control mechanisms described above.
Respiratory Distress Syndrome
Neonatal respiratory distress syndrome (RDS), also known as hyaline membrane disease, results from insufficient surfactant production most often associated with prematurity, but can occur when surfactant release or synthesis is delayed, as with infants of diabetic mothers, or with secondary inactivation of surfactant, as in meconium aspiration syndrome. The clinical features of untreated RDS result from poor lung compliance, increased work of breathing, loss of FRC, V–Q mismatch, poor gas exchange, right-left shunting via the ductus arteriosus and/or patent foramen ovale, and lung injury from barotrauma and volumetric trauma, oxidative injury, and inflammation [303]. The presentation of RDS includes tachypnea or apnea, retractions, grunting, hypoxemia, and respiratory and metabolic acidosis. The typical appearance of RDS on chest radiograph is a diffuse pattern of reticulogranular, or ground glass, opacities and air bronchograms representing air-filled large airways surrounded by atelectatic alveoli [304]. Symptoms tend to worsen for several days after birth, followed by improved respiratory function as endogenous surfactant production increases. The infant with RDS is at risk of serious complications associated with positive pressure ventilation (e.g., chronic lung disease, pneumothorax, infection), comorbidities (such as sepsis), and prematurity [e.g., injuries to other organ systems including the brain (see Section “CNS”)]. Neonates may present in the immediate postpartum period, since effective surfactant function is critical to establishing a gas-filled FRC during the first few breaths after birth.
The severity of pulmonary complications associated with RDS, such as chronic lung disease, has been reduced by exogenous surfactant, either synthetic or animal derived, delivered via a tracheal tube [305]. Commonly, transient hypoxemia may follow bolus delivery of surfactant. Obstruction of the tracheal tube, pulmonary hemorrhage, and inadvertent volutrauma (after compliance has improved) rarely complicates surfactant administration [306]. Symptoms and radiographic findings generally improve within hours [304]. The optimal dosing regimen (e.g., prophylactic vs. early rescue therapy; number and timing of doses) has yet to be confirmed [306]. Currently, the standard regimen consists of two doses of betamethasone 24 h apart, within 7 days prior to delivery, for fetuses 24–34 weeks gestational age [307]. Other treatment protocols for RDS include early introduction of nasal CPAP (nCPAP) in the delivery room in an effort to avoid intubation and the potentially injurious effects of positive pressure ventilation [308].
Treating the preterm fetus in utero via maternally administered steroids (betamethasone) accelerates lung maturation and increases the production and release of surfactant [309]. Of note, administering antenatal steroids provides an additive effect to that of exogenous surfactant in reducing the pulmonary sequelae associated with RDS, as compared with surfactant administration alone. In addition, a reduced incidence of IVH seems to be associated with the combination [305].
Despite advances in nursery care over the past several decades, approximately 20 % of infants with RDS will develop chronic lung disease, specifically bronchopulmonary dysplasia (BPD), characterized by persistent need for oxygen at 28 days of life [310], with grading of BPD at 36 weeks of gestation (for infants born <32 weeks gestation). BPD in the age of surfactant therapy differs from classic or “old” BPD, which was common in relatively mature premature infants who had been subjected to high levels of positive pressure ventilation and oxygen therapy [311]. Old BPD is characterized by alternating sites of hyperinflation (presumably areas of lung with normal compliance exposed to positive pressure ventilation) and atelectasis, extensive fibrotic areas, and severe epithelial and endothelial lesions [312]. “New” BPD develops in infants treated with modern RDS therapy (e.g., “gentle ventilation”), is most commonly seen in ELBW infants, and has different pathologic findings including enlarged, simplified alveoli, with some interstitial thickening [313]. New BPD appears to be a developmental disorder that results from disruptions in lung growth caused by factors other than a deficiency of surfactant [314]. Although neonates with the new BPD may have a more benign pulmonary presentation in the nursery, many develop a non-asthmatic obstructive airway disease as infants or children which has the potential to complicate ventilatory support, anesthetic care, and the postoperative course [311]. Although the long-term prognosis of new BPD has not been completely defined, the early disruption in normal lung growth and development may predispose to decreased respiratory reserve that may lie dormant until later life, becoming apparent when added to the expected decline in lung function associated with the aging process, smoking, or other respiratory insult [315].
Oxygen Toxicity
Although an essential metabolic substrate for human life, oxygen can also be toxic to the neonate through the creation of reactive oxygen species (ROS) [316]. ROS act as second messengers, and transcription factors important in growth and development are critical for immune function and regulate vascular beds including the ductus arteriosus [317]. However, exposure to ROS can also be harmful, leading to the release of mitochondrial cytochrome C and other apoptotic factors, degrading signal transduction, directly altering proteins and impairing protein synthesis, modifying nucleic acids including DNA base and strand injury, and affecting cell growth and development. Several mechanisms (thiol compounds such as thioredoxin and glutathione, uric acid, bilirubin, anti-oxyenzymes) maintain reduction-oxidation homeostasis [316, 317].
Eukaryotic life has evolved mechanisms to manage hypoxic environments, but not hyperoxia, as the atmospheric concentration of oxygen throughout evolution has been similar to or less than the current concentration [318]. In addition, during normal fetal development in utero, the expected environment is one of physiologic hypoxia compared with postnatal life, including the nearly anoxic conditions of the zygote, to late gestation, in which the PaO2 reaches only 20–30 mmHg. Humans have evolved mechanisms to tightly regulate oxygen levels, both at the macro level (carotid body reflexes) and at the cellular/mitochondrial level, likely involving the hypoxia-inducible factor 1 (HIF-1) [318]. This protein complex interacts with erythropoietin and governs a range of responses to hypoxia including the shift from aerobic to anaerobic metabolism [319, 320]. Animal studies have demonstrated a role for HIF-1 in the activation of gene products important for angiogenesis (via VEGF), stem cells proliferation, and CNS and pulmonary alveolar development (via the related HIF-2α) [318]. Exposure to oxygen decreases HIF activity, affecting developmental signaling, and, in that way, impacting the growth trajectory of the premature neonate developing ex utero [318]. At birth, the infant’s oxygen tension rapidly increases and may reach extreme levels if the FIO2 is greater than 0.21. That is, the increase in pO2 arrives during a period of critical growth for the premature infant, who, if developing in utero would be in a “hypoxic” milieu.
The effect of oxygen in the pathogenesis of retinopathy of prematurity (ROP) has long been recognized secondary to the obvious impairment of visual acuity. Although factors other than hyperoxia contribute to both the development and severity of ROP, the role of oxygen has been linked to the effects of changes induced in VEGF levels in the developing retina. In addition to toxicity in the retina, recent research has highlighted the potentially deleterious effects of oxygen on other organ systems after only a brief exposure (e.g., during neonatal resuscitation). In multiple systematic reviews, resuscitation with 100 % oxygen caused oxidative stress in animals [321–324] and humans [325]; neurologic injury in animals [326] and humans [327]; inflammation in the lung, heart, and brain in animals [328–330]; increased pulmonary vascular resistance and smooth muscle reactivity in animals [331]; kidney and cardiac cellular injury in humans [332]; and increased neonatal mortality [327, 333, 334].
Clinical guidelines for neonatal resuscitation have changed radically over the past decade as international and national associations have reacted to the mounting evidence of toxicity associated with excessive oxygen administration. Recommendations for resuscitation now include using pre-ductal pulse oximetry to guide the administration of oxygen, recognizing the critical role of establishing adequate ventilation, not hyperoxia, in the transition to extrauterine life [335, 336]. For the first 5–10 min of life, the hemoglobin oxygen saturation of the healthy term neonate is frequently <90 % (Fig. 2.13). For this reason, if an infant responds to the initial ventilatory support, 100 % oxygen is best avoided. In most studies, premature infants who receive supplemental oxygen titrated to achieve oxygen saturations <93 % SpO2 have a reduced incidence of ROP and lung injury compared with those maintained at excessive oxygen saturations (e.g., 95–98 %) [338, 339]. Still, the optimal targets for SpO2 during long-term nursery care have yet to be clarified. Although the toxic effects of hyperoxia are now widely recognized, an ongoing study has suggested that the mortality in extremely premature infants is increased when the targeted SpO2 is 85–89 % versus 91–95 % [340].


Fig. 2.13
Pre- and post-ductal SpO2 levels in healthy term infants during the first 15 min after birth (median; IQR) Post-ductal SpO2 levels were significantly lower than pre-ductal SpO2 levels at 3, 4, 5, 10, and 15 min. *P ≤ 0.05. Pre-ductal SpO2 measured on right hand; post-ductal on one foot (from Mariani et al. [337]) lung early SpO2
Control of Ventilation
Immature central nervous system control of ventilation may play a role in neonatal apnea and bradycardia [341] as well as postoperative apnea [342]. The process of maturation of the respiratory control responses begins in fetal life but continues after birth. For example, as discussed above, fetal breathing plays a critical role in lung growth and training of the respiratory pump. Although some aspects of fetal respiratory behavior persist postnatally, the responses of the healthy term neonate to hypoxia and hypercarbia and other respiratory stimuli are more appropriate [343]. In early gestation, fetal breathing is continuous and apparently under control of the spinal cord; as development progresses, fetal breathing is controlled more centrally and, by the third trimester, is a complex behavior that varies with sleep stage (e.g., in fetal lambs, breathing is inhibited during non-REM sleep by brainstem inhibitory nuclei) [343, 344]. In animals, hypercapnia stimulates the depth of fetal breathing [345–348]. Similar responses are present in the human fetus after 24 weeks gestation [348]. As is the case in adults, reduced CO2 tension decreases fetal breathing. In contrast, hypoxemia also decreases fetal breathing. One hypothesis suggests that in the setting of limited access to oxygen, the fetus eliminates respiratory effort to decrease oxygen consumption when “breathing” does not contribute to gas exchange [343]. Both decreasing fetal breathing and gasping are signs of fetal distress, are a component of the obstetrical “biophysical profile,” and correlate with abnormal fetal well-being [349–351]. After delivery, inhibition of breathing movements is life-threatening and must be reversed as the neonate now depends on pulmonary gas exchange for survival. The exact mechanism by which these pathways are reversed is unknown [343].
Although more mature than in utero, the neonate’s responses to hypercapnia, hypoxemia, and afferent stimulation remain impaired, especially for preterm infants. The immature, central-chemoreceptor-mediated hypercapnic ventilatory response has a relatively flat slope in the neonatal period; with increased postnatal and gestational age, the slope increases toward adult values [352]. In animal studies, the immature ventilatory response to CO2 is reflected in the failure to increase respiratory rate, although both immature and mature animals increase tidal volume in response to hypercarbia [353] (see Fig. 2.14). The origin of the diminished response to hypercapnia in the premature infant has been attributed to dysfunction of the central nervous system [343]. However, premature infants with a history of apnea exhibit a blunted ventilatory response curve to CO2 with a slope that is even less than that exhibited by similar preterm infants without apnea [354–356].


Fig. 2.14
Lung response to CO2: effect of 5-min exposure to 5 % CO2 on
(a), tidal volume (V t) (b), and frequency (c) at 5 and 22–23 days of age in unrestrained rats. Effect of 5-min exposure to 5 % CO2 on
(a), tidal volume (V t) (b), and frequency (c) at 5 and 22–23 days of age in unrestrained rats. Values are means ± SE. Hypercapnia caused a significantly greater increase in
at 22–23 days compared with 5 days. Percent increase in V t was similar at the 2 ages. Frequency decreased significantly from baseline at 5 days, whereas it increased significantly from baseline at 22–23 days (used with permission from Abu-Shaweesh et al. [353])



The ventilatory response to hypoxia is also immature in the preterm infant. Hypoxic preterm infants and term babies less than 1 week of age respond to hypoxia with 1–2 min of increased ventilation. However, unlike adults and most term infants older than a few weeks who sustain this response, the ventilatory effort of premature infants and term infants during the first week of life then wanes to less than baseline, a phenomenon termed “hypoxic ventilatory depression” (HVD) [343, 357, 358]. The first phase of the response to hypoxia is likely mediated by peripheral chemoreceptor located in the carotid body as denervation of the carotid bodies in animals abolishes this reflex [358]. In animal studies, the initial increase in ventilation is characterized by an increase in tidal volume with a gradual decrease in respiratory rate. In the second phase, HVD, the increased tidal volume is preserved, but the respiratory rate continues to decrease, resulting in a net decrease in minute ventilation [359].
The mechanism of HVD is not fully elucidated [360] but is thought to be related to CNS descending inhibitory tracts as suggested by animal studies in which lesions in the pons resulted in less depression [361]. Many neurotransmitters have been implicated in this central response to hypoxia, including adenosine, endorphins, GABA, and an imbalance between neurokinin-1 and mu-opioid receptors, as pharmacologic modulation of these substances also resolves HVD [343, 362]. Further support for the central origin of HVD includes the finding that hypoxia, in near-term infants, shifts the CO2 response curve to the right and decreases the slope (i.e., hypoxia blunts the CNS response to hypercapnia) [363].
Reflexes mediated by afferents in the airway, lungs, and chest wall that regulate ventilation are also immature in the preterm and young infant. For example, in preterm infants, mechanical or chemical stimulation of the larynx decreases ventilation and, in extreme cases, produces apnea [364]. Based on data from animals, the mechanism is associated with superior laryngeal nerve inhibitory stimulation leading to either decreased respiratory center activity or enhancement of CNS inhibition/expiratory pathways [365, 366].
The Hering–Breuer inflation reflex, a respiratory control mechanism observed in neonates, especially the preterm [367] that consists of inhibition of ventilation by lung inflation, is mediated by the slowly adapting pulmonary stretch receptors (SARs), disappears during REM sleep, and fades during the first few weeks of life. The Hering–Breuer reflex may stabilize ventilation in face of changing elastic loads [368].
A closely related phenomenon, the paradoxical reflex of Head, occurs when lung inflation triggers a large inspiratory effort resulting in a large lung volume and provides a rare example of a physiologic positive feedback mechanism. The reflex is accompanied by tonic inspiratory contractions [369, 370], which can be elicited in neonates via a forced inhalational maneuver with positive pressure [371], and is mediated by the rapid adapting stretch receptors (RARs) (also responsible for so-called Hering–Breuer deflation reflex in which rapid deflation of the lungs stimulates inspiration). The paradoxical reflex of Head may play a role in establishing the FRC during the transition from fetal life [371] and/or maintaining the lung volume in the setting of the compliant chest wall of the neonate [372].
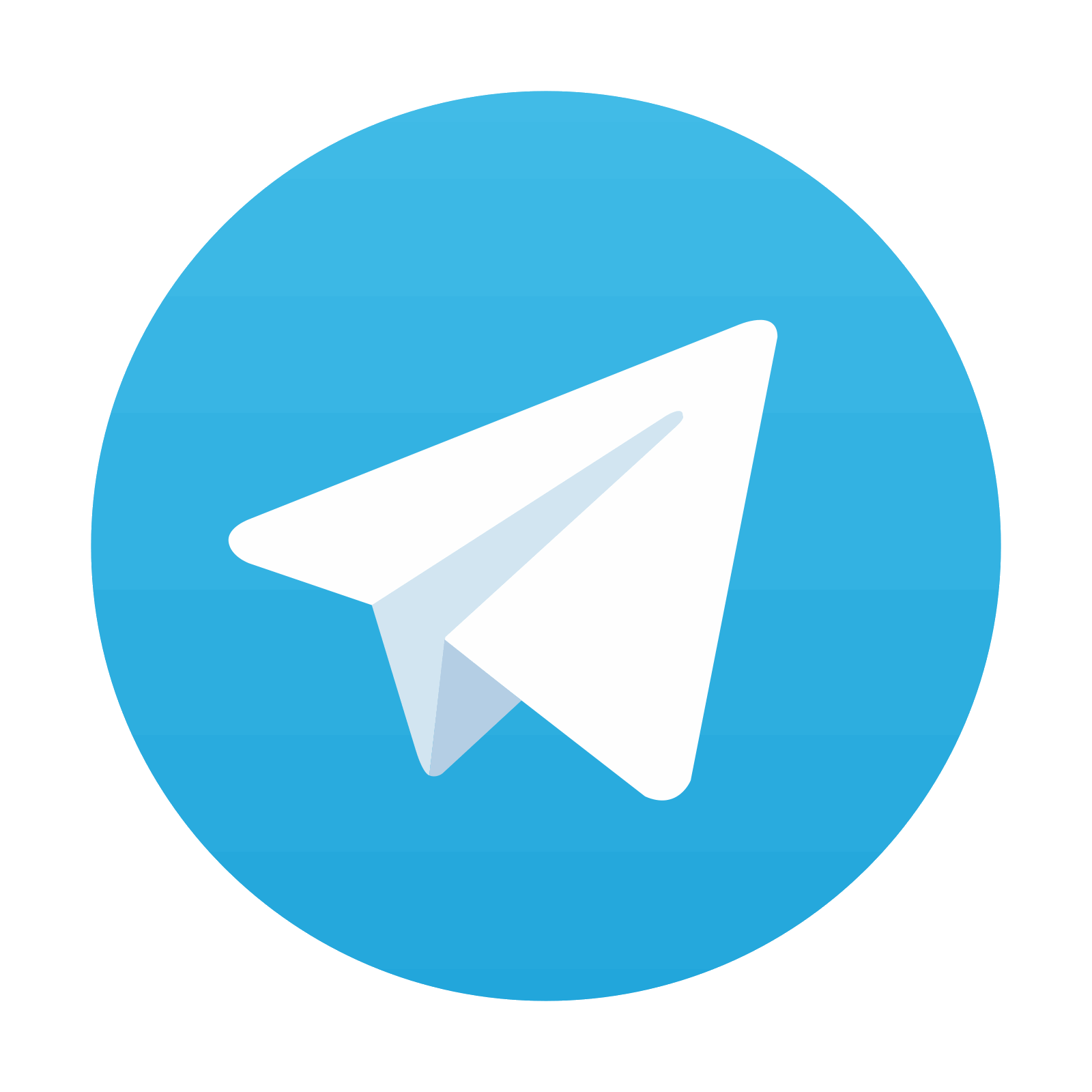
Stay updated, free articles. Join our Telegram channel
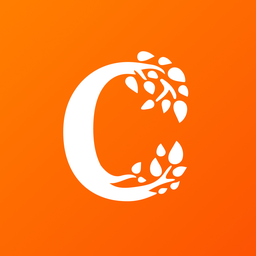
Full access? Get Clinical Tree
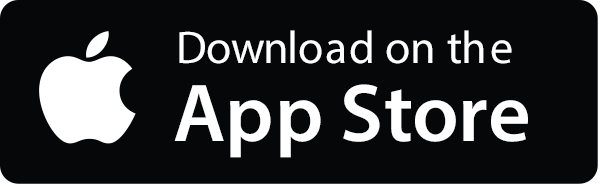
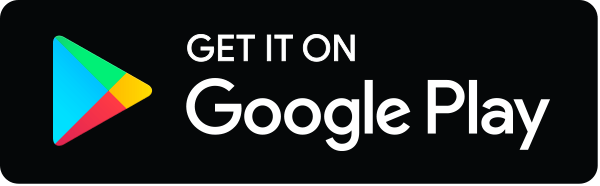