The goal of tracheal intubation is to provide a successful and definitive airway. Unfortunately, laryngoscopy and intubation can result in a cascade of physiological and pathophysiological reflex responses. These responses are initiated by stimulation of afferent receptors in the posterior pharynx supplied by the glossopharyngeal and vagus nerves. The central nervous system (CNS), cardiovascular system, and respiratory system all respond predictably to these afferent stimuli, and in selected patients the resultant physiologic manifestations may adversely affect the patients’ outcome. Though no data exist to suggest that patient outcomes are altered by attenuating the increases in intracranial pressure (ICP), stimulation of the autonomic nervous system with increases in the heart rate and blood pressure, and stimulation of the upper and lower respiratory tract resulting in increases in airway resistance, in light of the possible adverse effects in a compromised patient, it seems both reasonable and logical to attempt to attenuate these responses.
The CNS responds to airway manipulation by increasing cerebral metabolic oxygen demand (CMRO2) and cerebral blood flow (CBF). If the intracranial compliance is decreased (“tight brain”), the increase in CBF may increase the ICP further. This response is important in situations in which there is a loss of autoregulation such that blood flow to the brain, or regions of the brain, becomes pressure-passive (i.e., increases in blood pressure result in increases in ICP).
Laryngoscopy stimulates protective reflexes and predictably leads to cardiovascular and respiratory system responses mediated by the sympathetic nervous system. In children, this process is believed to be primarily a monosynaptic reflex promoting vagal stimulation of the sinoatrial node resulting in bradycardia. For further details see Chapter 3 “Preparation for Awake Intubation.” These responses may be detrimental in patients with myocardial ischemia (“tight heart”), known intracerebral or aortic aneurysms, major vessel dissection, or those with major vascular injuries. Hypertension may also lead to significant increases in ICP if autoregulation has been lost (e.g., acute severe head injury or intracranial hemorrhage).
The respiratory system may respond in three important ways to laryngoscopy and intubation: activation of the upper airway reflexes leading to laryngospasm; coughing; and bronchospasm (“tight lungs”). Laryngospasm, a forceful involuntary spasm of the laryngeal musculature, may produce difficulty with intubation as well as ventilation. Persistent and life-threatening laryngospasm is treated with a gentle continuous positive airway pressure with 100% oxygen, intravenous (IV) lidocaine (1.5 mg·kg−1), or if persistent, neuromuscular blockade (e.g., succinylcholine at 10% of the intubating dose). Negative intrathoracic pressure created by inspiration attempts against a closed glottis (laryngospasm) may result in negative pressure pulmonary edema (see Chapter 61).
Coughing may produce significant adverse effects in patients with increased ICP, an unstable cervical spine injury, or penetrating eye injuries.
Activation of the lower airway reflexes leads to an increase in airway resistance. This reaction is most often manifested by bronchospasm brought about by reflexes, irritants, or antigens. Whether mitigation of these reflexes improves patient outcomes is not known. However, it is known that pre-laryngoscopy administration of drugs is capable of mitigating these potentially harmful physiologic effects.
Current evidence that this symphony of adverse advents can be mitigated is most compelling for the IV administration of anesthetics, including lidocaine, opioids, muscle relaxants, and much less so for atropine, except in the case of pediatric patients. This chapter provides a general discussion of the appropriate pharmacological agents used in airway management and their relevant properties. It should be emphasized that the goal of this chapter is not to discuss the rationale of using a specific sedative or muscle relaxant in an anticipated difficult airway. Rather, this discussion will center on the use of drugs to facilitate tracheal intubation and mitigate adverse physiological consequences.
Pre-laryngoscopy agents (also known as “pretreatment agents”) are used to attenuate the adverse physiologic responses to laryngoscopy and intubation. Pretreatment agents fall into the following categories: beta-blockers, local anesthetics, opioids, and defasciculating agents. Ideally, all pretreatment agents should be administered shortly before (typically about 3 minutes) induction to synchronize the onset of peak drug effects of all drugs administered in the airway management sequence.
While short-acting beta-blockers, such as esmolol, have been shown to be beneficial in attenuating the sympathetic response to laryngoscopy,1 they are not effective in attenuating to any extent a rise in ICP. They potentially increase airway resistance, especially in patients with reactive airways disease. Furthermore, beta-blockers are also negative inotropes and in some clinical situations, particularly emergencies in which maximum cardiac reserve should be preserved, the combination of beta-blockers and the negative cardiovascular effects of most induction agents could be catastrophic.
Local anesthetics bind to closed, inactivated sodium channels and prevent subsequent channel activation. Sodium channels in the closed inactivated state are not permeable to sodium and therefore action potentials cannot be generated. Local anesthetic agents are a combination of a lipophilic benzene ring and a hydrophilic amine either linked by an amide or an ester bridge. Lidocaine is a low-potency, rapid-onset, and intermediate-acting amide local anesthetic. The literature is replete with articles that offer varying conclusions as to the efficacy of lidocaine when used in the pretreatment phase of tracheal intubation to attenuate various adverse effects of laryngoscopy and intubation.
Attenuation of the elevation of ICP related to airway manipulation is ascribed to lidocaine’s ability to increase the depth of anesthesia, decrease CMRO2 demand globally, decrease CBF, and increase cerebrovascular resistance. Patients with intracranial pathology may have an abnormal intracranial pressure–volume relationship, which can predispose them to abrupt, extreme, and prolonged elevations of ICP. Such increases can contribute to secondary injury of the brain, such as herniation of the brain and impaired perfusion leading to ischemia. Indirect evidence exists that lidocaine can attenuate the intracranial hypertensive response to laryngoscopy and intubation. For instance, intracranial hypertension associated with tracheal suctioning is suppressed by IV lidocaine.2–5 Intratracheal lidocaine is equally effective.4 Lidocaine (1.5 mg·kg−1) administered 3 minutes before intubation suppresses the cough reflex and attenuates the increase in airway resistance resulting from laryngoscopy and endotracheal intubation.
There is evidence that local anesthetic attenuates the hemodynamic response to laryngoscopy. Pretreatment with local anesthetics decreases the chance of arrhythmias and ischemic electrocardiogram changes following laryngoscopy.6 A systematic review of 37 trials found that IV lidocaine attenuates the hemodynamic response to laryngoscopy in all age groups.7
Lidocaine’s effect on antigen-mediated bronchospasm is more controversial. Lidocaine and other local anesthetics may lead to bronchoconstriction in patients with reactive airway disease when given via the inhalational route (see Chapter 3 for a full discussion of this evidence).8 In normal individuals, lidocaine does not alter airway resistance, and has been shown to produce mild bronchodilation.9 In three similar trials, Groeben et al.10–12 showed that IV lidocaine attenuated the response to an inhalational histamine challenge in patients with mild asthma. The inhalational administration of lidocaine to such patients, however, was met with short-lived initial increases in airway resistance.11 In a randomized controlled trial of 60 asthmatic patients, administration of IV lidocaine did not significantly reduce airway resistance after intubation.13
If pretreating is deemed necessary, IV lidocaine (1.5 mg·kg−1) 3 minutes before induction is advocated for adult patients with reactive airway disease (i.e., “tight lungs”) or those with elevated ICP (i.e., “tight brains”). The onset of action is 45 to 90 seconds after an IV bolus of lidocaine and the duration of effect is 10 to 20 minutes.14 Lidocaine has a wide safety margin, particularly at a dose of 1.5 mg·kg−1. The primary toxic effect is the development of seizures, which usually occurs at much higher doses. IV lidocaine at rates of 1.5 to 3.0 mg·kg−1·min−1 resulted in seizures in healthy volunteers once the total dose reached 6 to 8 mg·kg−1.15,16 The maximum dose for subcutaneous lidocaine is generally considered to be 4.5 mg·kg−1. plain or 7 mg·kg−1 with epinephrine.14,17 However, even healthy volunteers often experience some neurologic symptoms, such as perioral numbness/tingling, auditory disturbances, and dizziness, with IV bolus doses as low as 1 mg·kg−1.18
Opium, derived from the seeds of the poppy Papaver somniferum, is composed of more than 20 alkaloids, some of which serve as the foundation molecules for modern opioids. A German pharmacist isolated the first alkaloid from opium in 1806 and named it morphine after the Greek god of dreams, Morpheus.19 A broad definition of the term opioid includes all drugs, synthetic and natural, which interact with opioid receptors, whether endogenous, exogenous, agonist, or antagonists. Opioids are routinely employed during an anesthetic induction and with the advent of newer, more rapid-acting agents (such as remifentanil) they continue to play a role in airway management.
Opioids act throughout the nervous system, including the dorsal horn of the spinal cord (substantia gelatinosa), periaqueductal gray matter, and in the periphery. They inhibit presynaptic release and postsynaptic response to excitatory neurotransmitters, such as acetylcholine (ACh) and substance P, by altering the potassium and calcium conductance.19 As such, they are useful in attenuating adverse responses to airway manipulation in patients with “tight brains” and “tight hearts.”
Although highly selective, opioid effects typically involve complex interactions among various receptor sites. The list of opioid receptors continues to expand. Mu (µ), kappa (κ), and delta (δ) receptor classes and their subtypes have been firmly established.20 Most clinically useful opioids are highly selective µ agonists, which are responsible for the bulk of supraspinal and spinal analgesia.
Opioids are typically of low molecular weight but vary widely in their lipid solubility, percent of ionization, and degree of binding to proteins (Table 4–1). Commonly used opioids for induction (fentanyl, sufentanil, and remifentanil) are more lipid soluble, which accounts for their speed of onset of action. Redistribution is responsible for the termination of their CNS drug action. Metabolism of most opioids occurs through a two-stage hepatic process that generally results in an inactive metabolite. The exception is remifentanil, which contains an ester linkage and is rapidly hydrolyzed by nonspecific esterases in the blood and tissue.
Morphine | Fentanyl | Sufentanil | Remifentanil | |
---|---|---|---|---|
pKa | 7.9 | 8.4 | 8 | 7.2 |
% Ionization | 23 | 8.5 | 20 | 58 |
% Protein bound | 35 | 84 | 90 | 66 |
Rapid redistribution-t ½π (min) | 1.2–1.9 | 1.4 | 0.4–0.5 | |
Redistribution-t ½α (min) | 1.5–4.4 | 9.2–19 | 17 | 2–3.7 |
Elimination-t ½β (h) | 1.7–3.3 | 3.6–6.6 | 2.2–4.1 | 0.17–0.33 |
Peak effect (min) | 7 | 3–5 | 5–6 | 1–2 |
Duration (min) | 60–180 | 30–60 | 40 | 10–20 |
Induction dose(μg·kg−1) | 1–4 | 0.3–1 | 0.5–3 |
Physiologically, opioids exert their effect on all organ systems. Venodilation and depressed sympathetic reflexes typically result in a decrease in heart rate and blood pressure. Unlike some opioids (most notably morphine) fentanyl, sufentanil, and remifentanil do not release histamine. This is partly why they cause less hypotension than morphine. All opioids depress ventilation by blunting the carbon dioxide response at the respiratory center, raising the apneic threshold, and depressing the slope of the CO2 response curve (Figure 4–1).
FIGURE 4–1.
Carbon dioxide (CO2) ventilatory response curve. In healthy, awake controls increasing partial pressure of carbon dioxide in arterial blood (PaCO2) results in an increase in minute ventilation (V̇). Increasing plasma concentration of opioids shift the CO2 response curve to the right. High concentrations eventually result in depression of the slope of the curve.19

The primary reason to include an opioid in the intubation sequence is their ability to significantly attenuate the sympathetic response that occurs with manipulation of the airway. Unfortunately, the rapid administration of fentanyl and its derivatives has been associated with brief episodes of coughing and chest wall rigidity.24–26 Large induction doses of an opioid (e.g., sufentanil 3 μg·kg−1) can decrease pulmonary compliance in 50% to 86% of patients and potentially induce glottic closure interfering with ventilation of the patient.24–27 Doses of this magnitude are seldom if ever employed in emergency airway management, in or out of the operating room (OR).
The hemodynamic and intracranial responses to tracheal intubation are usually short lived. Therefore, the “ideal” choice of an opioid for tracheal intubation should be based on its pharmacokinetic and pharmacodynamic characteristics, namely, a rapid onset and a brief duration of drug effect. While there are currently many opioids available, the following discussion will be restricted to only those opioids with a rapid onset and short duration of drug effect.
Like meperidine, fentanyl is a phenylpiperidine-derived synthetic opioid agonist. It is roughly 100 times more potent than morphine. Fentanyl in large doses (10–30 µg·kg−1) causes chest wall rigidity in 35% to 85% of patients.24,26,28 This side effect is present in all age groups.29 Rigidity is a unique and idiosyncratic response to opioids and is probably related to the dose and speed of administration. Although the exact mechanism is unknown, an animal study demonstrated opioid-induced muscle rigidity coincident with activation of central µ receptors.30 Others have suggested mechanisms involving the cerulospinal noradrenergic system31,32 and the neurochemical system affected in Parkinson’s disease.33
Tagaito et al.34 found that increasing doses of fentanyl reduced the incidence of respiratory and laryngeal responses to laryngeal irritation under propofol anesthesia. Fentanyl is a short-acting opioid owing to redistribution of the drug, although accumulation with repeated dosing can limit its use. Peak drug effect occurs 3 to 5 minutes following IV administration with an equilibration half-time between the plasma and brain of 5 minutes.23 Doses recommended for attenuating the adverse effects of airway manipulation range from 1 to 4 µg·kg−1. One must be prepared to treat dose-related hypotension and respiratory depression that may occur with fentanyl administration.
Fentanyl may decrease airway reflexes associated with the placement of an extraglottic device (EGD); however, the doses required may interfere with spontaneous ventilation once the EGD is placed. Fentanyl 1 μg·kg−1, when given 5 minutes prior to placement of an EGD, has been shown to increase the rate of apneas and need for manual ventilation, while the frequency of laryngospasm and breath holding were similar to placebo.35 One study estimated that the ED50 of fentanyl is 0.5 μg·kg−1 and the ED95 is 7.5 μg·kg−1 for insertion of an EGD following propofol induction.36 Thus, the need for optimal conditions and the absence of airway reflexes must be weighed against the effects of large doses of opioids.
A thienyl derivative of fentanyl, sufentanil is 10 to 15 times more potent than fentanyl. Sufentanil is a highly specific µ agonist with similar pharmacokinetic properties as fentanyl. It is extremely lipophilic permitting rapid penetration of the blood–brain barrier and onset of CNS effects. Sufentanil 0.3 to 1 µg·kg−1, administered 1 to 3 minutes prior to intubation, will blunt the response to laryngoscopy. Rigidity has been reported with larger doses.28 The difficult ventilation associated with opioid rigidity may be due in part to vocal cord closure when administered in doses of 3 µg·kg−1.25,27
Remifentanil is a structurally unique opioid. It is a piperidine analog with a methyl ester side chain. The ester linkage renders it susceptible to cleavage by nonspecific plasma and tissue esterases.37 The rapid metabolism of remifentanil, rather than redistribution, is responsible for its ultrashort duration of action; and with limited redistribution, there is no accumulation. It is rapidly cleared from the plasma (3 L·min−1).28 Remifentanil is not, however, a substrate for pseudocholinesterase and therefore it is unaffected by pseudocholinesterase deficiency.
Remifentanil is a potent µ receptor agonist with a similar potency to fentanyl. Doses of 0.25 to 3 µg·kg−1 have successfully attenuated the response to laryngoscopy.38–43 At higher doses, centrally mediated depression of sympathetic tone and vagally induced bradycardia may occur. This bradycardia can be attenuated by the prior administration of glycopyrrolate or other antimuscarinics.19 Peak respiratory depression occurs 5 minutes after bolus dosing, and lasts 10 minutes after 1.5 µg·kg−1 and 20 minutes after 2 µg·kg−1.44 Remifentanil, like other opioids, may cause chest wall rigidity depending on the dose and speed of administration.45
The clinical utility of remifentanil is reflected in its distinctive pharmacokinetic characteristics. Its rapid onset, brief duration, and easily titratable nature have enhanced its profile for airway management, particularly in emergency settings, and for short surgical procedures. A small dose of remifentanil (0.25 µg·kg−1) can provide excellent conditions for laryngeal mask airway insertion with minimal hemodynamic disturbance.46 The trachea may be successfully intubated without muscle relaxants with the combination of remifentanil and an induction agent. Remifentanil, 2 to 4 µg·kg−1, in this setting, provides good to excellent intubating conditions.40,47–49 Remifentanil has been found to be superior to lidocaine and esmolol at attenuating the hemodynamic responses to intubation.50
The ideal induction agent would quickly render the patient unconscious, unresponsive, and amnestic in one arm/heart/brain circulation time. Such an agent would also provide analgesia, maintain stable cerebral perfusion pressure (CPP) and cardiovascular hemodynamics, be immediately reversible, and have few, if any, adverse side effects. Unfortunately, such an induction agent does not exist.
Most induction agents are highly lipophilic, and therefore have a rapid onset of effect within 30 to 45 seconds of IV administration. Their clinical effect is likewise terminated quickly as the drug rapidly redistributes from the CNS to less well-perfused tissues. All induction agents have the potential to cause myocardial depression and hypotension. These effects depend on the particular drug and the patient’s underlying physiologic condition. The faster the drug is administered, the larger the amount of the drug that will be delivered to those organs with the greatest blood flow (such as the brain and heart) and the more pronounced the effect. The choice of drug and the dose must be individualized to each patient to capitalize on desired effects, while minimizing adverse effects.
Anesthetic induction is intended to rapidly render the patient unable to appreciate, respond to, or recall noxious stimuli. It is at one end of a spectrum that runs from awake, alert, and oriented at one end to deep anesthesia and death on the other.
The induction agents include short-acting barbiturates: thiopental and methohexital; benzodiazepines: principally midazolam; and miscellaneous agents: etomidate, ketamine, and propofol. Opioids can function as anesthetic induction agents when used in very large doses (e.g., fentanyl 30 µg·kg−1), but are rarely, if ever, used for that purpose during routine or emergency intubation, so will not be discussed in this chapter.
All of the induction agents discussed in this chapter share similar pharmacokinetic characteristics. As mentioned previously, they are highly lipophilic, and therefore a standard induction dose of an induction agent administered to a euvolemic, normotensive patient will take effect within 30 seconds. The duration of observed clinical effect of each drug is measured in minutes and is due to the redistribution of the drug from the central circulation (brain) to larger, but less well-perfused tissues, for example, fat and muscle. The elimination half-life (t ½β, usually measured in hours) is characterized by each drug’s reentry from fat and lean muscle into plasma down a concentration gradient followed by hepatic metabolism, which precedes renal excretion. It generally requires four to five elimination half-lives to clear the drug completely from the body.
Because the target organ is the brain, and the desired effect is produced rapidly following bolus injection of the drug, dosing of induction agents in normal-sized and obese adults should be based on ideal body weight in kilograms. Hypovolemia results in the patient having a contracted central compartment; therefore, lower doses of induction agents are necessary to achieve adequate levels at the target organ (brain). Aging affects the pharmacokinetics of induction agents. In older adults, lean body mass and total body water decrease while total body fat increases, resulting in an increased volume of distribution, an increase in t ½β, and an increased duration of drug effect. Older adults are also much more sensitive to the hemodynamic and respiratory depressant effects of these agents, and consequently, most induction doses should be reduced.
Barbiturates are derived from barbituric acid, a cyclic compound obtained by the combination of urea and malonic acid. These agents act at the barbiturate receptor, which forms part of the GABA-receptor complex to enhance and mimic the action of GABA. In North America, use of barbiturates for airway management is very uncommon; however, they remain in common use in many countries around the world. The most commonly used barbiturates are thiopental and methohexital.
Thiopental, the prototypical barbiturate, undergoes hepatic oxidation and derivatives are excreted via the kidney. Methohexital shares similar clinical pharmacologic properties with thiopental although it is significantly less lipid soluble and is two to three times more potent than thiopental. The elimination of methohexital is three to four times faster than thiopental.51
Barbiturates have primarily been used as an induction agent for patients suspected of having increased ICP. Cerebral perfusion pressure is defined as the difference between the mean arterial pressure (MAP) and the ICP (CPP=MAP−ICP). The Monro–Kellie hypothesis states that blood, cerebrospinal fluid and brain tissue exist in equilibrium inside of a non-distensible cranial vault.19 The ability of barbiturates to decrease ICP stems from the cerebral vasoconstriction and subsequent decrease in CBF. The decreased systemic vascular resistance is not matched by the decreased CBF and so CPP is maintained and possibly increased.52 Methohexital is often used in those patients undergoing electroconvulsive therapy (ECT) because of its desirable rapid onset of amnesia and short duration of action.
Thiopental and other barbiturates are absolutely contraindicated in patients with acute intermittent porphyria, or variegate porphyria, as they can activate the enzyme responsible for precipitating an acute attack, which can be life-threatening.
In euvolemic, normotensive adults, a recommended induction dose of thiopental is 3 to 5 mg·kg−1. Apart from stimulating the release of histamine from mast cells, thiopental is a potent venodilator and myocardial depressant.53,54 Consequently, the dose must be decreased in patients with decreased intravascular volume, those with compromised myocardial function, and older patients. Thiopental should be avoided entirely in severely hypotensive patients. The onset of methohexital is more rapid and the duration of action shorter than it is with thiopental. The recommended induction dose of methohexital in the euvolemic, normotensive patient is 1.5 mg·kg−1.
The chief side effects of thiopental include central respiratory depression, venodilation, and myocardial depression. Thiopental causes a dose-related release of histamine (anaphylactoid, as distinct from anaphylactic, response), which in most situations is not clinically significant, but may exacerbate hypotension or bronchospasm in patients with reactive airways disease.54 Although barbiturates produce dose-dependent depression of ventilation centers, they do not completely blunt airway reflexes.55
There is a 10% to 20% incidence of nausea and vomiting that occurs during recovery from a barbiturate induction. Methohexital has a greater incidence than thiopental of twitching and hiccups (excitatory phenomena) that may be misdiagnosed as seizures.56
Although chemically distinct from the barbiturates, the benzodiazepines also exert their effects via the GABA-receptor complex. Benzodiazepines are allosteric modulators of the GABAA receptor; specifically, benzodiazepines bind to the benzodiazepine site on the GABAA receptor complex thereby increasing the receptor complex affinity for GABA.57 The benzodiazepines provide amnesia, anxiolysis, sedation, anticonvulsant effects, and hypnosis (sleep). This potent, dose-related amnestic property is perhaps their greatest asset.
The lipophilicity of the benzodiazepines varies widely. Greater lipid solubility confers a more rapid onset of action because of the brain’s high lipid content. The two benzodiazepines most clinically used during airway manipulation are midazolam and diazepam. Of the two, midazolam is the more lipid soluble. However, many studies have shown that the onset of effect is 2.5 times slower for midazolam than diazepam.58 Induction of anesthesia with midazolam alone has been described. With doses of 0.15 to 0.2 mg·kg−1 induction of anesthesia occurs after 1.5 minutes (0.3–8 minutes) with opioid premedication or 2 to 2.5 minutes without. Therefore, the time to clinical effectiveness of benzodiazepines is longer than any of the other induction agents, which hinders their role in emergency airway management. The termination of action of midazolam and diazepam is due to redistribution and subsequent hepatic metabolism via cytochrome P450–3A4 microsomal oxidation. Midazolam has one insignificant active metabolite and a t ½elim of 2 to 4 hours. Diazepam has two active metabolites, both of which can prolong its sedative effect but more importantly are metabolized and excreted more slowly than diazepam and account for its prolonged t ½β of 20 to 40 hours. The benzodiazepines do not release histamine, and allergic reactions are very rare. Clearance will be prolonged in older adults, critically ill patients, obese patients, or patients with renal disease or low cardiac output.59
The primary indications for benzodiazepines are anxiolysis, amnesia, sedation, and seizure management. Because of their dose-related reduction in systemic vascular resistance and direct myocardial depression, dosage must be adjusted in volume-depleted or hemodynamically compromised patients. Unlike the other induction agents, including those that cause hypotension, midazolam is generally under-dosed during emergency induction. The dose of midazolam for induction of anesthesia is 0.1 to 0.3 mg·kg−1.60–63 Doses of 0.15 to 0.2 mg·kg−1 are purported not to cause hypotension in normal individuals.59
Most often midazolam is used as an adjunct to other induction agents. Its amnestic effects may be advantageous during RSI, particularly is the case of hemodynamic instability where lower doses of induction agents will be used. Midazolam causes anterograde amnesia in a dose-dependent manner (2–10 mg IV); however, there is no evidence that it causes retrograde amnesia.64,65
Etomidate is a carboxylated imidazole derivative that is primarily an IV hypnotic agent used for the rapid induction of anesthesia. It is available in a 0.2% solution dissolved in 35% propylene glycol. It inhibits the reticular activating system, mimicking GABA at the GABA-receptor complex. Etomidate owes its rapid onset to its high lipid solubility and large nonionized portion at physiological pH.28 Its rapid redistribution leads to prompt awaking following administration. The elimination half-life of etomidate is 2 to 5 hours. It is rapidly hydrolyzed by hepatic metabolism and plasma esterases to water-soluble inactive metabolites excreted primarily by the kidneys.
Etomidate is a potent direct cerebral vasoconstrictor.66 It attenuates underlying elevated ICP by decreasing CBF and CMRO2. Its hemodynamic stability preserves CPP. The use of etomidate in patients with seizure disorders should be limited to the induction of anesthesia and not its maintenance. Etomidate has been shown to increase electroencephalographic activity in certain leads67 and is less effective than other agents in attenuating the motor activity associated with seizures.68 Etomidate has no analgesic properties.
The respiratory system may be centrally stimulated by etomidate, so administration is not usually associated with apnea unless opioids are coadministered.28,69 Etomidate lacks any direct bronchodilatory properties. Eames et al.55 prospectively demonstrated that 2.5 mg·kg−1 of propofol was superior to either 0.4 mg·kg−1 etomidate or 5 mg·kg−1 thiopental in decreasing mean airway pressure during bronchoscopy in 75 patients.
Etomidate is touted as the most hemodynamically stable induction agent.70–72 It mildly decreases the systemic vascular resistance but appears to have no direct effect on cardiac output or contractility. Gauss et al.73 conducted an echocardiographic assessment of the hemodynamics of various induction agents and showed that etomidate produced no changes in the hemodynamic variables measured.
Etomidate is associated with nausea and vomiting during recovery in 30% to 40% of patients undergoing general anesthesia. Pain on injection is common because of the propylene glycol solvent. The incidence can be as high as 80%.74 Myoclonic movements due to an imbalance of inhibition and excitation in the thalamocortical tract are common and have been confused with seizure activity.67 It is of no clinical consequence and generally terminates promptly. The occurrence of hiccups, usually during awakening, is highly variable, ranging between 0% and 70%.75
The most significant and controversial side effect of etomidate is its reversible blockade of 11-beta-hydroxylase. Etomidate causes reversible adrenocortical suppression by interfering with 11-beta-hydroxylation of 11-deoxycortisol, which prevents its conversion to cortisol. It decreases both serum cortisol and aldosterone levels. Single bolus induction doses of etomidate have transiently inhibited cortisol and aldosterone synthesis.76–78 Studies of elective surgical patients receiving etomidate showed a reduction in serum cortisol levels but no change in mortality.79 A retrospective study and an RCT of critically ill trauma patients also found evidence of adrenal suppression without a significant change in mortality.80,81 Adrenal suppression is of the greatest concern in septic patients. One systematic review including mainly RCT studies found an increase in mortality when a single dose of etomidate was used for septic patients.82 This is especially true to patients requiring multiple vasopressors and those with an intra-abdominal source of infection.83 However, several large cohort studies did not find a difference in mortality in septic patients who received a single dose of etomidate.84–86
Etomidate has been largely abandoned for most indications due to this reversible adrenal suppression. As per the discussion above, use of etomidate is not advised in septic patients and use in trauma patients is controversial at best. Furthermore, ketamine offers similar hemodynamic stability in these patients groups.81 There is some evidence that etomidate provides better quality seizures during ECT.87
As with any induction agent, dosage must be adjusted in hemodynamically compromised patients. In euvolemic and hemodynamically stable patients, the normal induction dose of etomidate is 0.2 to 0.4 mg·kg−1. In compromised patients, the induction dose should be reduced commensurate with the patient’s clinical status.
The use of etomidate to facilitate airway management in the patient with status epilepticus is not contraindicated, as it does depress the level of consciousness, and long-term medication with benzodiazepines or propofol ordinarily follows the intubation.
Ketamine, an arylcyclohexylamine, is a structural analog of phencyclidine, which may account for the psychomimetic side effects, such as hallucinations and nightmares. The compound has a chiral center producing stereoisomers and is commercially available as a racemic mixture. It is fairly lipid soluble and minimally protein bound accounting for its rapid onset (45–60 seconds). Ketamine is extensively taken up by the liver and metabolized by the cytochrome P-450 biotransformation system. The primary metabolite, norketamine, has roughly 1/5 the potency of the parent molecule. Its high lipid solubility produces rapid redistribution and short duration of effect. Rapid hepatic metabolism is responsible for the short elimination half-life of 2 to 3 hours. Ketamine has been touted as a “complete” anesthetic by many, as it provides analgesia, amnesia, and hypnosis (unconsciousness). Besides blocking polysynaptic spinal cord reflexes, ketamine is a noncompetitive antagonist of N-methyl-d-aspartate (NMDA) receptors at the GABA-receptor complex, promoting the inhibition of excitatory neurotransmitters in selected areas of the brain. An interaction between ketamine and a number of the opioid receptors may be partially responsible for its analgesic properties. Ketamine produces dissociative anesthesia by electrophysiologically separating the thalamus from the limbic system. Patients may appear conscious but are unable to respond to, and are unaware of stimuli. Ketamine centrally stimulates the sympathetic nervous system, which in turn releases catecholamines, augmenting the heart rate and blood pressure in those patients who are not catecholamine-depleted secondary to the demands of their underlying disease. Similar to most other anesthetics, however, ketamine in the face of catecholamine depletion causes dose-dependent myocardial depression.
Traditionally, ketamine’s direct stimulation of the CNS is thought to increase cerebral metabolism, CMRO2, and CBF, thus potentially increasing ICP in patients with CNS injury. These generalizations may not be valid. Albanese et al.88 studied the effects of ketamine (5 mg·kg−1) in eight patients with traumatic brain injury who were ventilated and sedated with propofol. There was a significant decrease in the ICP, while the CPP and middle cerebral artery blood flow velocity were unchanged. One systematic review comparing propofol, ketamine, etomidate, and midazolam for sedation of patients in the ICU with traumatic brain injuries found no difference in intracranial pressure or CPP.89 There is some evidence that the antagonist behavior of ketamine at NMDA receptors may have a neuroprotective benefit.90 Ketamine has been used to decrease the hemodynamic response to endotracheal suctioning in the ICU for traumatic brain injury patients.91 Ketamine is unlikely to cause a significant rise in ICP with induction and intubation, given that suctioning did not result in significant variation in MAP, CPP, or CBF velocity.91 Unfortunately, the evidence with respect to the use of ketamine for induction of anesthesia specifically in the patient with intracranial hypertension is lacking. However, it can be said that ketamine may be used in the sedated, mechanically ventilated patient with little concern for worsening intracranial hypertension.92–94 Ketamine’s long-term exclusion from the induction of patients with decreased intracranial compliance may be an overgeneralization.
A key feature of ketamine is its effects on the respiratory system. Despite inducing profound anesthesia, upper airway reflexes and central respiratory drive are preserved to a degree following ketamine administration; although with high doses, hypoventilation and apnea will occur. In addition, ketamine directly relaxes bronchial smooth muscle, producing bronchodilation. Ketamine has been successfully used to treat status asthmaticus and less severe bronchospasm.95–98 Hemmingsen et al.96 in a placebo-controlled trial showed that ketamine (1 mg·kg−1) successfully relieved bronchospasm in ventilated subjects.
With Its Unique Characteristics, What Is the Role of Ketamine as an Induction Agent for Airway Management?
Ketamine is the traditional induction agent for patients with reactive airways disease who require tracheal intubation. Because of its unique pharmacologic profile, ketamine may also be considered for induction in patients who are hypovolemic and for patients with hemodynamic instability due to cardiac tamponade or distributive shock. In normotensive or hypertensive patients with ischemic heart disease, catecholamine release may adversely increase myocardial oxygen demand. For patients with known ischemic heart disease being induced for coronary artery bypass grafting, ketamine was found to increase heart rate by an average 20 bpm and mean arterial blood pressure by an average 40 mm Hg. Systolic function, diastolic function, and stroke volume index were all reduced based on pre- and post-induction echocardiography.99 Therefore, ischemic heart disease and hypertension are relative contraindications to the use of ketamine for induction.
On the basis of the previous discussion, the use of ketamine in patients with elevated ICP remains controversial. Traditional teaching would have us avoid ketamine despite there being little to no evidence to support such a practice.
The induction dose of ketamine is 1 to 2 mg·kg−1. This dose needs to be adjusted for hypovolemic and/or hypotensive patients. Ketamine may be mixed with propofol as a 50:50 mixture (5 mg·mL−1 of each), “ketofol,” a combination that produces less hypotension, but more respiratory depression than propofol alone.100
Ketamine is commonly used in low- and middle-income countries for induction and maintenance of anesthesia. The scarcities of vasopressors, advanced airway equipment, and anesthesia providers with advanced airway training make the stable hemodynamic profile and ability to maintain airway reflexes very desirable. Despite paucities in other medications and equipment, ketamine is relatively available.101
Because of its stimulating effects, ketamine enhances laryngeal reflexes and increases pharyngeal and bronchial secretions. These secretions may precipitate laryngospasm and be bothersome during upper airway examination in the difficult airway patient or during procedural sedation. An antimuscarinic agent such as glycopyrrolate may be administered in conjunction with ketamine to promote a drying effect. The maintenance of airway reflexes does not negate the need for intubation and airway protection when faced with patients at risk for aspiration.
Five to 30% of patients will experience hallucinations or dreams on emergence from ketamine. They are more common in the adult than in the child and can be eliminated by the concomitant or subsequent administration of a benzodiazepine.92
Propofol (2,6-diisopropylphenol) is an alkylphenol derivative with hypnotic properties. Propofol is supplied in an emulsion of 10% soybean oil, 2.25% glycerol, and 1.2% egg lecithin.102 It is highly lipid soluble and hence rapidly acting. The initial redistribution half-life is 2 to 8 minutes. It is rapidly metabolized to inactive, water-soluble metabolites.
Propofol enhances GABA activity at the GABA-receptor complex. It decreases CMRO2 and ICP.103–105 Propofol causes a direct reduction in blood pressure through vasodilation and direct myocardial depression, resulting in a decrease in CPP, which may be detrimental in a compromised patient.106 Propofol has profound anticonvulsant properties.107 It is a potent depressor of ventilation, inhibiting hypoxic and hypercarbic ventilatory drive.
Propofol is an excellent induction agent in hemodynamically stable patients. Its potential for hypotension and reduction in CPP may reduce its role as an induction agent for rapid sequence intubation/induction (RSI). However, it remains a commonly used agent in this setting as the adverse hemodynamic changes and other pharmacological effects of propofol are very predictable. Altered dosing for the unstable patients or using it in conjunction with ketamine, “ketofol,” helps to attenuate the undesirable hemodynamic effects.
There are no absolute contraindications to the use of propofol. The company product monograph lists egg and soy allergies as contraindications due to the presence of 1.2% egg lecithin and 10% soybean oil.108 Similarly, there are concerns for patients with peanut allergies because of the cross reactivity between soy and peanut antigens. Nevertheless, propofol administration is likely safe in these patients since several studies have found that patients do not react to propofol, even if they are sensitized to egg, soy, or peanuts.109,110
The induction dose of propofol is 1 to 2 mg·kg−1 in a euvolemic, normotensive patient. Because of its predictable tendency to reduce mean arterial blood pressure, smaller doses are generally used when propofol is given as an induction agent for emergency induction.
The pain associated with the injection of propofol is comparable to that of methohexital, less than etomidate, and more than thiopental.19 This effect can be attenuated by injecting the medication through a rapidly running IV infusion in a large vein. Alternatively, pretreatment with a small dose of 1% lidocaine (4 mL)111 or mixing the propofol with a small amount of 1% lidocaine (2–4 mL) prior to injection112 have both been shown to minimize the discomfort. Propofol can cause mild clonus but to a lesser degree than thiopental, etomidate, or methohexital.67
Given the limitations of the currently available induction agents, there are ongoing efforts to develop the “ideal” induction agent. Many of these agents will likely not become widely used, however, being up to date with the development of new agents will help you to understand the limitations of our current agents and predict changes in future practice.
Remimazolam (CNS 7056) is a new ester-hydrolyzed benzodiazepine that has completed a Phase IIa trial.113 It has rapid onset and short duration of action. Its onset of action is 1.5 to 2.5 minutes compared to 5 minutes for midazolam.113 Its sedative effect lasts for 10 minutes in comparison to 40 minutes after administration of midazolam.114
JM-1232 (-) (also known as MR04A3 when administered as a 1% aqueous preparation) is an isoindoline-derivative benzodiazepine.114 It is chemically different from other benzodiazepines but is a full agonist of the benzodiazepine receptor. It has shown a favorable safety profile in humans and may have analgesic effects.115,116
As discussed above, etomidate has fallen out of favor due to propensity to cause adrenocortical suppression. Methoxycarbonyl-etomidate (MOC-etomidate) is a rapidly metabolized analogue of etomidate, which causes only very brief adrenocortical suppression.114 In a lipopolysaccharide sepsis model in rats, MOC-etomidate caused less adrenal suppression and lower mortality than etomidate.117 It shows similar hemodynamic stability as etomidate.114
Carboetomidate is an analogue of etomidate where the nitrogen atom on the imidazole ring has been removed, decreasing its ability to interact with 11-beta-hydroxylase (the enzyme responsible for adrenal suppression) by three orders of magnitude.114 In rat models of sepsis, carboetomidate causes less adrenal suppression and less proinflammatory cytokine production than etomidate.118
AZD3043 is an allosteric modulator of the γ-aminobutyric acid type A (GABAA) receptor. This is a member of the new class of agents referred to as “soft” drugs characterized by their rapid degradation to inactive metabolites. AZD3043 is rapidly hydrolyzed by esterases, including butyrylcholinesterases, present in blood and liver.119 AZD3043 induces anesthesia with good hemodynamic stability and minimal respiratory depression. In Phase I trials, boluses of AZD3043 were associated with a dose-dependent increase in heart rate and blood pressure. Apnea occurred at higher bolus doses but lasted a maximum of 97 seconds, not requiring assisted ventilation.120 Onset and recovery were both rapid, although dose dependent.120 Similar hemodynamic and respiratory stability was observed during infusions of AZD3043, along with a relatively short recovery period in doses high enough to achieve a bispectral index <60%.119,120
Neuromuscular blocking agents are the cornerstone of emergency airway management and are used to obtain total muscle relaxation to facilitate rapid endotracheal intubation while minimizing the risks of aspiration or other adverse physiologic events. Neuromuscular blocking agents do not provide analgesia, sedation, or amnesia, and an induction or sedative agent must be used in combination during RSI in patients who are not completely unresponsive. Similarly, appropriate sedation is essential when neuromuscular blockade is maintained for controlled mechanical ventilation following intubation.
In order to understand the pharmacology of neuromuscular blocking agents, it is important to understand their effects at the post-junctional, cholinergic, nicotinic receptors at the neuromuscular junction. Under normal circumstances, the neuron synthesizes ACh from choline and acetate and packages it in vesicles. Each vesicle contains 5000 to 10,000 ACh molecules. Calcium enters the nerve through channels that open in response to the action potential propagating the length of the nerve. Calcium permits the binding of specific proteins to the vesicles necessary for them to bind to the nerve end membrane. Binding produces fusion and the release of ACh. The ACh migrates across the synaptic cleft and attaches to nicotinic ACh receptors, promoting muscle fiber depolarization producing muscle contraction. ACh then detaches from the receptor to be eligible for reuptake into the nerve terminal or hydrolysis by acetylcholinesterase, which also resides in the cleft.
Neuromuscular blocking agents are either ACh agonists (depolarizers of the motor end plate) or antagonists (non-depolarizers of the motor end plate). The antagonists attach to the receptors and competitively block ACh from accessing ACh receptors. Because they are in competition with ACh for the motor end plate, they can be displaced from the end plate by increasing concentrations of ACh, the end result of reversal agents (the cholinesterase inhibitors), such as neostigmine and edrophonium, which inhibit acetylcholinesterase and allow ACh accumulation and the return of neuromuscular function.
In clinical practice, there are two classes of neuromuscular blocking agents: the competitive and noncompetitive (or depolarizing) neuromuscular blocking agents, of which succinylcholine is the prototype and the only one in common clinical use. The competitive or non-depolarizing agents are divided into two main classes: the benzylisoquinolinium compounds and the aminosteroid compounds. The benzylisoquinolines, d-tubocurarine, metocurine, atracurium, cisatracurium, and mivacurium share common properties. The aminosteroids, vecuronium, pancuronium, rapacuronium, and rocuronium also share common attributes that are distinct from those of the benzylisoquinolines.
The ideal muscle relaxant to facilitate tracheal intubation would have a rapid onset, a short duration of action, no significant adverse side effects, metabolism and excretion independent of liver and kidney function, immediate reversibility, and a low cost. Unfortunately, such an agent does not exist. Succinylcholine comes closest to meeting all these desirable goals. Despite the historic and well-known adverse effects of succinylcholine and the continuous advent of new competitive neuromuscular blocking agents, succinylcholine remains an essential drug in facilitating tracheal intubation.
What Are the Clinical Pharmacological Characteristics of Succinylcholine That Make It a Unique Neuromuscular Blocking Agent?
Succinylcholine is chemically similar to ACh. It consists of two molecules of ACh linked by an ester bridge and, as does ACh, succinylcholine stimulates the nicotinic and muscarinic cholinergic receptors of the sympathetic and parasympathetic nervous systems. Once succinylcholine reaches the neuromuscular junction, it binds tightly to the ACh receptors. ACh receptors related to neuromuscular transmission are located in three areas:
The presynaptic receptors, responsible for regulation of ACh vesicles release, generate an action potential along the neuron.
The postsynaptic receptors: the principle paralyzing component of succinylcholine occurs at the postsynaptic receptor. The resultant depolarization and subsequent desensitization to further stimulation produced by succinylcholine occurs because unlike ACh, succinylcholine is not rapidly hydrolyzed by acetylcholinesterase. Succinylcholine is resistant to degradation by cleft acetylcholinesterase and is susceptible to rapid hydrolysis by pseudocholinesterase, an enzyme of the liver and plasma not present at the neuromuscular junction. Therefore, diffusion away from the neuromuscular junction motor end plate and back into the vascular compartment is ultimately responsible for succinylcholine metabolism. This also explains why only a fraction of the initial IV dose of succinylcholine ever reaches the motor end plate to promote paralysis.
Extrajunctional receptors, although numerous, are generally not of clinical significance. In pathological states such as denervation injuries or severe muscle crush injury, these receptors become unregulated and proliferate. The depolarization of the extrajunctional receptors in large numbers can result in clinically significant hyperkalemia.
Succinylmonocholine, the initial metabolite of succinylcholine, sensitizes the cardiac muscarinic receptors in the sinus node to repeat doses of succinylcholine, which may then lead to atropine responsive bradycardia.
Succinylcholine remains the neuromuscular blocking agent of choice for emergency RSI because of its rapid onset and relatively brief duration of action. A personal or family history of malignant hyperthermia (MH) is an absolute contraindication to the use of succinylcholine. Patients judged to be at risk for succinylcholine-related hyperkalemia also represent absolute contraindications to its use. Under these circumstances, to facilitate tracheal intubation rapidly, a large dose of a competitive, non-depolarizing neuromuscular blocking agent should be used.121 Relative contraindications to the use of succinylcholine are dependent on the skill and proficiency of the airway practitioner and individual patient’s clinical circumstance (i.e., “context sensitive”; see Chapter 7). A patient who is predicted to be a difficult intubation, who is also predicted to be difficult or impossible to bag-mask-ventilate, should not receive any neuromuscular blocking agents unless one is “forced to act” (see Chapter 2) and is prepared to immediately perform a surgical airway.
In the normal-sized adult patient, the recommended dose of succinylcholine for intubation is 1 to 2 mg·kg−1. In a rare, life-threatening circumstance when succinylcholine must be given IM because of inability to secure venous access, a dose of 3 to 4 mg·kg−1 IM may be used.
The intubating dose is typically felt to be two to three times the dose that produces on average 95% decrease in twitch height of the adductor pollicis muscle (effective dose or ED95). The ED95 of succinylcholine is 0.3 to 0.6 mg·kg−1 in adults,122,123 0.5 mg·kg−1 in children, and 0.7 mg·kg−1 in infants and neonates.124 Therefore, an appropriate intubating dose of succinylcholine for a normal-sized adult is 1 mg·kg−1. For obese patients dosing appears to be more successful if the total body weight is used rather than ideal body weight.125 The average onset time is 1 to 1.5 minutes. Defasciculation with a non-depolarizing muscle relaxant (NDMR) will decrease the potency of succinylcholine by half. Therefore, if one intends to prevent fasciculations by pretreating with a non-depolarizing agent, a succinylcholine dose of 1.5 to 2 mg·kg−1 is required for intubation.123
Succinylcholine is a rapidly cleared by plasma pseudocholinesterase which is an enzyme manufactured in the liver. Once succinylcholine diffuses out of the synaptic cleft it is hydrolyzed to succinylmonocholine and then to succinic acid. The mean elimination half-life of succinylcholine is 43 seconds.126 The rate of metabolism determines the duration of action. A normal adult has a theoretical 8-minute apneic reserve of oxygen. The mean time to return of spontaneous ventilation after an intubating dose of succinylcholine (1 mg·kg−1) is approximately 5 to 8 minutes.127 Hayes et al.127 did, however, have 10% of their patients desaturate before the return of spontaneous ventilation. The airway practitioner still has to provide mechanical ventilation until respiratory muscle function returns. Doses less than 1 mg·kg−1 may not compromise intubation conditions and do not shorten the apneic period.128
The recognized side effects of succinylcholine include fasciculations, hyperkalemia, bradycardia, asystole, prolonged neuromuscular blockade, MH, and masseter muscle spasm. Each of these will be discussed separately.
Fasciculations are involuntary, unsynchronized muscle contractions caused by depolarization of ACh receptors. Fasciculations cause muscle damage that manifests itself as myalgia, increased creatinine kinase, myoglobinemia, and an increase in catecholamines leading to increases in heart rate and blood pressure. These uncontrolled muscle contractions increase oxygen consumption and carbon dioxide production, and can lead to increased cardiac output, and potentially CBF and ICP. The increase in oxygen consumption has been theorized to produce more rapid desaturation in patients where succinylcholine is employed compared to those where a non-depolarizer is used.129,130 In an animal study, the increase in ICP was found to be secondary to the increase in muscle spindle activation caused by succinylcholine-induced spindle depolarization.131 Lastly, fasciculations tend to increase intragastric pressure, though this is not clinically significant as it is offset by an increase in lower esophageal sphincter tone.
Virtually all patients receiving succinylcholine will experience fasciculations. Data from 52 randomized trials show that fasciculations occur in 94% (range 73%–100%) of patients receiving succinylcholine and myalgias in 51% (range 10%–83%) at 24 hours.132 However, 15% to 20% subjects undergoing surgery without being exposed to succinylcholine will suffer from postoperative myalgias.133 Not only is the link between fasciculations and myalgias controversial,132 there is also no evidence that the severity of fasciculations corresponds with more severe myalgias,134 nor does it appear to be a dose-related side effect. In fact, McLoughlin et al.135 suggest that increasing the dose of succinylcholine may decrease the incidence of myalgia, suggesting that the larger dose leads to a more synchronous contraction and a reduction in shearing forces.
Small doses of NDMRs given prior to succinylcholine have clearly been shown to decrease the incidence and intensity of fasciculations.136–141 A meta-analysis determined that atracurium, d-tubocurarine, gallamine, and pancuronium can decrease the frequency of fasciculations and myalgias.141 Traditionally, 10% of the intubating dose of an NDMR has been given to pretreat for fasciculations. However, at this dose, a significant number of patients experience weakness. The limited evidence that exists does not suggest a dose response effect, meaning that there is no advantage to increasing the pretreatment dose from 10% to 30% of the intubating dose.132 The timing of rocuronium administration (3 vs. 1.5 minutes) prior to the succinylcholine does not seem to decrease it effectiveness.140
Lidocaine is an alternative to NDMRs for defasciculation. At doses of 1.5 mg·kg−1, lidocaine effectively decreases the incidence of fasciculation and myalgia following the administration of succinylcholine.134,141 Lidocaine appears to work by preventing ionic exchange across the sodium channels.
Sodium channel blockers (lidocaine, phenytoin), rocuronium, and gallamine have all been shown to reduce succinylcholine-related myalgia with a number needed to treat (NNT) of 3 in randomized trials. NSAIDs have an NNT of 2.5 to reduce myalgia.132
Under normal circumstances, serum potassium increases minimally when succinylcholine is administered (0–0.5 mEq·L−1) due to depolarization of the myocytes. A pathological response to succinylcholine can occur, however, resulting in rapid and dramatic increases in serum potassium. These pathologic hyperkalemic responses occur by two distinct mechanisms: receptor upregulation and rhabdomyolysis. In either situation, potassium increase may approach four times the normal efflux of potassium, resulting in hyperkalemic dysrhythmias, or cardiac arrest.142 Two forms of post-junctional ACh receptors exist, mature (junctional) and immature (extrajunctional). Each ACh receptor is composed of five proteins arranged in circular fashion around a common channel. Both types of receptors contain two alpha subunits. ACh must attach to both alpha subunits to open the channel and effect depolarization and muscle contraction. When receptor upregulation occurs, the mature receptors at and around the motor end plate are gradually converted over a 4- to 5-day period to immature receptors that propagate throughout the entire muscle membrane. Upregulated receptors are characterized by low conductance and prolonged channel opening times, resulting in increasing levels of potassium, clinically significant dysrhythmias, and cardiac arrest.143 Most of the entities associated with hyperkalemia during emergency RSI are the result of receptor upregulation. Interestingly, these same extrajunctional nicotinic receptors are relatively refractory to non-depolarizing agents, so larger doses of vecuronium, pancuronium, or rocuronium will be required to produce paralysis.
Rhabdomyolysis is the other mechanism by which hyperkalemia may occur. It is most often associated with myopathies. In cardiac arrest situations related to rhabdomyolysis and continued loss of potassium, resuscitation seems to be less successful than in receptor upregulation. Gronert142 reviewed 129 cases of cardiac arrest from hyperkalemia; 57 were due to rhabdomyolysis and 72 due to upregulation. The mortality was higher in those cases of rhabdomyolysis (30%) compared to cases of upregulation (11%). Succinylcholine is a toxin to unstable membranes in any patient with a myopathy and should be avoided.
Receptor upregulation occurs in burn victims, patients that suffer denervation injury, and in patients with sepsis or widespread inflammation. In burn victims, extrajunctional receptor sensitization becomes clinically significant at 4 to 5 days post burn. It lasts an indefinite period of time, although the “at-risk” period is deemed to have passed at the point healing of the burned area is complete. It is prudent not to administer succinylcholine to post-burned patients if any question exists regarding the status of their burn. The percent of body surface area burned does not determine the magnitude of hyperkalemia; significant hyperkalemia has been reported in patients with as little as 8% total body surface area burn.144 Most emergency intubations for burns are performed well within the safe 4-day window after the burn occurs. There have been no reports of hyperkalemia in the first 24 hours post-burn. It seems rational to avoid the administration of succinylcholine until the burn has completely healed.
The patient who suffers a denervation event secondary to a lower motor neuron or upper motor neuron injury is at risk for hyperkalemia. Following lower motor neuron denervation injuries, patients exhibit a sensitivity to succinylcholine, which begins 3 to 4 days post injury143 and patients suffering from severe polyneuropathies display increased potassium release following the administration of succinylcholine.145,146 Upper motor neuron lesions, such as stroke and traumatic closed head injuries, display a similar sensitivity to succinylcholine, usually 3 to 5 days after the event.143 As long as any neuromuscular disease is active, one ought to expect that there will be augmentation of the extrajunctional receptors and risk for hyperkalemia with the use of succinylcholine. Congenital upper motor neuron and lower motor neuron lesions, such as cerebral palsy and myelomeningocele, do not exhibit an altered response to succinylcholine.147,148 The duration of the upregulation and altered response to succinylcholine in neuromuscular disorders is not clear. Upregulation has been observed 3 years following an injury and may last even longer in progressive disease types.143 Unlike fasciculations, the hyperkalemic response cannot be attenuated by administering defasciculating doses of NDMRs, and therefore, these specific clinical situations should be considered absolute contraindications to succinylcholine during the specified time periods.
Infection or inflammation can alter the neuromuscular junction response to muscle relaxants.143 This situation is complicated by the intensive care unit environment where total body disuse atrophy and chemical denervation of the ACh receptors can occur if muscle relaxants are chronically infused. Exaggerated hyperkalemic responses to succinylcholine have been observed in patients with life-threatening infections.149,150 The at-risk time period appears to be 5 days after the illness has begun and continues indefinitely as long as the disease process is active.
Succinylcholine is absolutely contraindicated in patients with inherited myopathies such as, Duchenne and Becker muscular dystrophies. The combination of the succinylcholine-induced contractures and the fragile muscle membrane of the myopathic patients predisposes them to rhabdomyolysis.151 In children up to 10 years of age, an elevated creatine kinase is a highly sensitive indicator of muscular dystrophy.152 Myopathic rhabdomyolysis and hyperkalemia-induced cardiac arrest is associated with a significant degree of mortality. The hyperkalemic efflux can be four times the expected normal response. Any inappropriate response by young males following the use of succinylcholine should alert the practitioner to the possibility of undiagnosed Duchenne muscular dystrophy.153
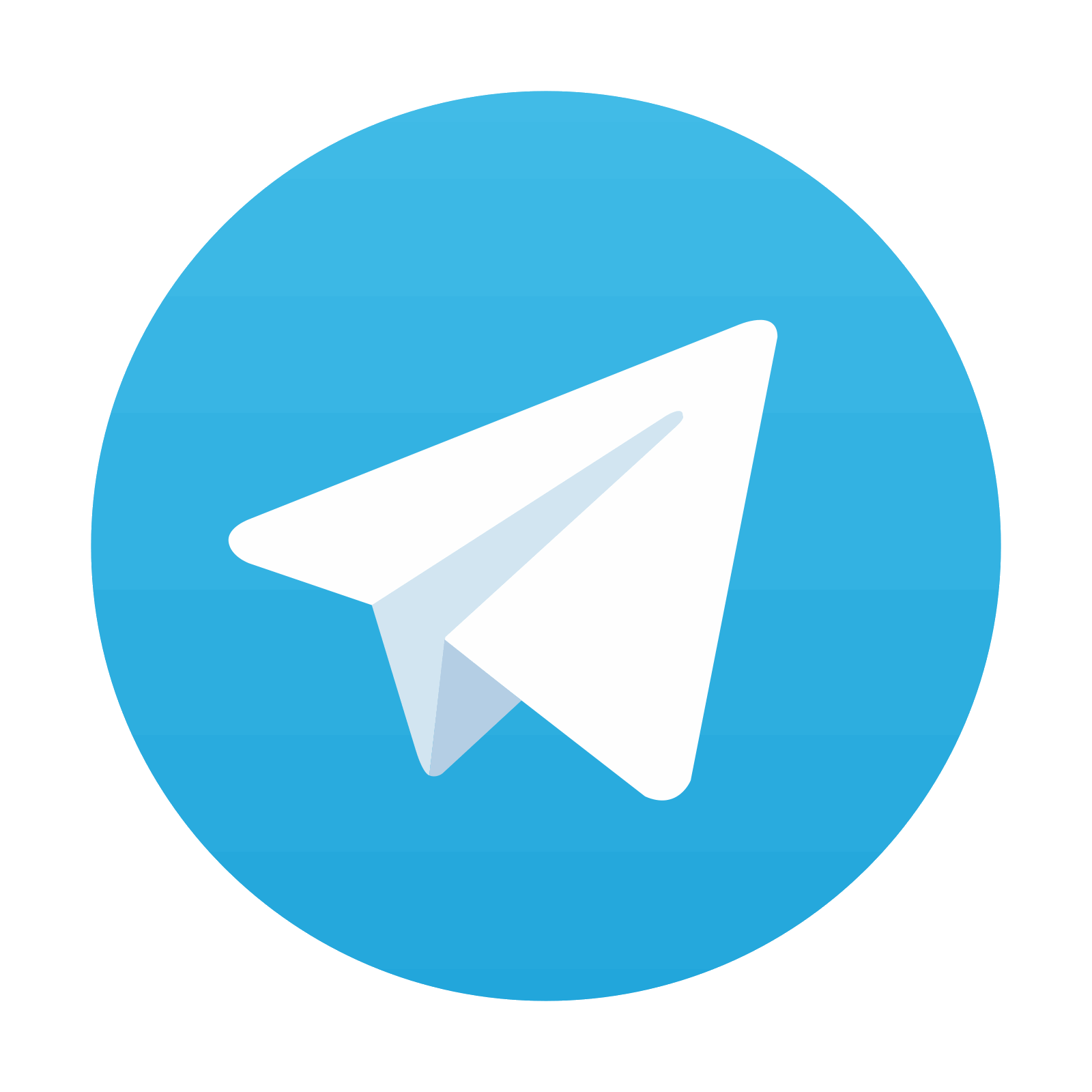
Stay updated, free articles. Join our Telegram channel
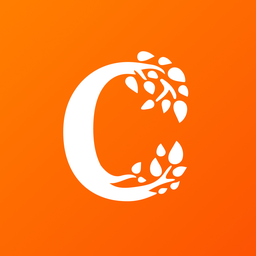
Full access? Get Clinical Tree
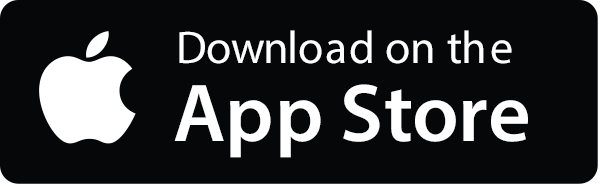
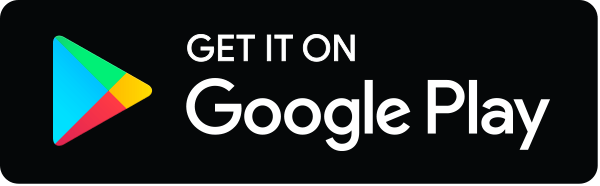