Pesticide Poisoning
William K. Chiang
Richard Y. Wang
A pesticide is as an agent intended for killing, preventing, repelling, or mitigating any pest. With the increasing use, environmental contamination and reports of epidemic pesticide poisoning are inevitable [1,2,3]. The health consequences from the long-term and low-level exposure to these chemicals, such as carcinogenesis [4,5], teratogenicity [6], fertility [7], and neurologic sequelae [8,9], may be significant and immeasurable. In many countries in which there are limited regulations on pesticide usage, pesticide ingestion is one of the leading forms of suicide, and pesticide exposure is a major occupational risk [10,11,12]. Even in the United States, pesticide exposures remain a major public health problem [13]. The World Health Organization estimated that accidental and occupational pesticide poisonings worldwide account for 1.5 million cases and 28,000 deaths annually [14].
This chapter focuses on selected pesticides that are most clinically important. Some of the common pesticides are provided in Table 141.1. Organophosphate insecticides are covered in Chapter 128. Further information on the identification and toxicity of pesticide products may be obtained from sources such as material data safety sheets, Hayes’ Handbook of Pesticide Toxicology, Farm Chemicals Handbook, and the pesticide label database (http://www.cdpr.ca.gov/docs/label/labelque.htm).
Organochlorines
Organochlorines are commonly used as insecticides, soil fumigants, solvents, and herbicides. Human toxicity can result from either acute or chronic exposure. Contamination typically occurs during production and application of these agents. Infants and toddlers are at risk for toxicity from bioaccumulation in foodstuffs, excretion in breast milk, and concentration in fetal tissues [15,16,17]. These toxicants can cause a variety of systemic manifestations, but are most notable for their central nervous system (CNS) effects. Organochlorines can be divided into four structural categories: dichlorodiphenyltrichloroethane (DDT) and related agents, hexachlorocyclohexanes, cyclodienes, and toxaphenes.
DDT is a well-known organochlorine. It was a popular insecticide in the agricultural industry during the 1960s. The many environmental concerns related to the use of DDT, including carcinogenesis, bioaccumulation, and other health risks to humans and animals, led to the banning of its use in the United States as of 1972. DDT is no longer being produced in the United States. Dicofol (a miticide) and methoxychlor are structurally related to DDT. The cyclodienes include chlordane, heptachlor, endrin, aldrin, and dieldrin. The use of several of these insecticides in the United States was discontinued between 1988 and 1990. Some of the other organochlorines that are structurally related to the cyclodienes include endosulfan, chlordecone, kelevan, and mirex. Endosulfan is considered highly toxic and is registered for agricultural, but not residential, use in the United States [18]. Mirex and chlordecone (Kepone) are no longer being used in the United States.
Pharmacology
The organochlorines are well absorbed from the gastrointestinal (GI) tract. For example, death can occur within 2 hours of intentionally ingesting endosulfan, and most deaths associated with chlordane have been from oral exposures in children. The serum half-lives of these chemicals are long, varying from days to months, because of their high lipid solubility. This allows these agents to be stored in fatty tissues (e.g., brain), with the resultant delay in total body clearance. The organochlorines are known to concentrate in breast milk and fetal tissue. At delivery, it has been shown that fetal blood and tissue had higher concentrations of lindane (γ-hexachlorocyclohexane, Kwell) than maternal samples [16,17]. However, teratogenic effects have not been demonstrated in the limited number of animal studies performed [19].
Organochlorines are metabolized by the microsomal enzymes in the liver. Toxaphene, chlordane, DDT, and lindane can induce microsomal enzyme activity and affect not only their own metabolism but also the effects of coadministered medications [20].
Chlordane has several metabolites, such as heptachlor, oxychlordane, and heptachlor epoxide. Most of the available information on chlordane and metabolite tissue distribution is from case reports of accidental and suicidal exposures. Depending on the source, the elimination half-life of chlordane varies from 21 to 88 days [21,22]. Most of the chlordane and metabolites are excreted by the biliary system. On absorption into the body, aldrin is rapidly metabolized to the epoxide derivative, dieldrin. Because very little of aldrin remains, its toxicity is attributed to dieldrin. Dieldrin is stored in fatty tissues, and its elimination half-life in humans is approximately 369 days [23]. Endrin, an isomer of dieldrin, is rapidly metabolized in both humans and animals, with an elimination half-life of 2 to 6 days [24].
Table 141.1 Common Pesticides | ||||||
---|---|---|---|---|---|---|
|
Table 141.2 Organochlorine Levels of Toxicity | ||||||
---|---|---|---|---|---|---|
|
Organochlorines have several mechanisms of action. They alter sodium- and potassium channel movement across the neuronal membranes and can be considered axonal toxins. With DDT, sodium ion transport is facilitated and potassium transport is inhibited. This results in the spontaneous firing and prolongation of action potentials and repetitive firing after a stimulus. DDT also inhibits Na+/K+ adenosine triphosphatase and calmodulin activities, which reduces the rate of neuronal repolarization. This may account for some of the neurologic manifestations such as paresthesias, thought disturbances, myoclonus, and seizures. Cyclodienes, hexachlorocyclohexanes, and toxaphenes manifest neurotoxicity by inhibiting γ-aminobutyric acid receptor function in the CNS [25]. In the limbic system, lindane can directly excite neurons and result in agitation and seizures [25,26]. Abnormalities in respiratory rate patterns can result from direct medullary toxicity or pulmonary aspiration. The level of toxicity of the various organochlorines can be categorized into high, moderate, and low (Table 141.2).
Clinical Toxicity
Poisoning can result from ingestion, dermal absorption, or inhalation. Inadvertent human exposures to aldrin and dieldrin have resulted from pesticide spraying, which causes dermal and inhalational absorption. The use of lindane in home vaporizers has resulted in significant inhalation toxicity [27]. Agents such as dieldrin, lindane, and Kepone have good dermal penetration. Workers who directly handled lindane had health complaints of headaches, paresthesias, tremors, confusion, and memory impairment [28]. Also, seizures have been reported in occupational surveys among sprayers and applicators of aldrin and dieldrin [29,30]. As little as two total body applications on two successive days of 1% lindane (Kwell), a common scabicide, resulted in seizures in an 18-month-old child [31]. The peak concentration of lindane occurs 6 hours after dermal application; thus, delayed and prolonged manifestations of toxicity may occur from dermal absorption. Dermatitis can occur from the topical exposure to dicofol and methoxychlor [24]. Intradermal and subcutaneous injections of these agents can result in chemical dermatitis and sterile abscesses [32]. Dicofol and methoxychlor have minimal toxicity. Human volunteers ingesting up to 2 mg per kg per day of methoxychlor for 8 weeks did not demonstrate any ill effects [33].
Seizures are the most prominent CNS effect of these agents. The seizures occur soon after exposure, may present without a prodrome, and can be quite protracted in frequency [24,34,35,36,37,38]. Late-onset seizures may result from delayed GI or dermal absorption. Acute exposures to DDT present initially with tremors, nausea, vomiting, muscle weakness, and confusion, which may progress to seizures [39]. Among the organochlorines, both psychomotor agitation and CNS depression have been described. Chlordecone, mirex, and endosulfan are more likely to cause tremors and agitation than seizures. Kelthane, perthane, methoxychlor, and lindane are more likely to cause CNS sedation than excitation. Endrin is considered one of the most toxic of the chlordienes, with reports of hyperthermia and decerebrate posturing [24]. In 1984, an outbreak of endrin toxicity from contaminated foodstuffs occurred in Pakistan, where seizures resulted in a 10% mortality rate [36].
Neurologic symptoms resolve quickly because of rapid distribution of the organochlorines from blood to lipid stores. Because redistribution back into the blood pool can occur at a later time, continual observation of the patient for delayed toxicity may be warranted. Some of the long-term CNS effects (i.e., thought disturbances) after significant exposures may be due to direct chemical toxicity or anoxic encephalopathy from sustained seizures [40]. Chlordecone is a recognized neurotoxin, causing peripheral neuropathies [41].
Nausea, vomiting, and diarrhea may occur after ingestions, especially if petroleum distillates are part of the preparation. Pulmonary aspiration of these agents can cause tachypnea and significant respiratory distress, with resultant pulmonary edema [40]. When dicofol is heated or comes in contact with an acid, it decomposes to hydrogen chloride, which causes respiratory irritation [24]. Hypersensitivity pneumonitis may result from inhalational exposures when the organochlorine is mixed with pyrethrins.
Cardiac dysrhythmias, including ventricular fibrillation, have been reported from organochlorine exposure [42]. Halogenated hydrocarbons sensitize the myocardium to catecholamines, which results in a variety of rhythm disturbances. Cardiotoxicity can be exacerbated by either stress-provoking events or the exogenous administration of catecholamines. In severely ill patients, other causes of cardiac dysrhythmias, such as hypoxia and acidemia, should be considered.
Significant elevations in liver enzymes were reported in a group of 19 workers with a 10-year lindane exposure [43]. Animal studies with acute oral exposures to lindane have demonstrated fatty degeneration and necrosis of the liver [44]. From the few reports of human exposures to chlordane, there is little evidence of hepatotoxicity from this agent [21,45,46]. Microsomal enzyme induction has been demonstrated in animals that were orally administered chlordane. Long-term exposure among 233 workers with aldrin, dieldrin, endrin, and telodrin for 4 to 12 years was not associated with any significant elevation of hepatic enzymes or hepatic enzyme induction.
Hematologic dyscrasias, including aplastic anemia, leukopenia, leukocytosis, granulocytopenia, granulocytosis, eosinophilia, thrombocytopenia, and pancytopenia, have been reported after repeated lindane exposures [27,47]. However, all of the involved preparations also contained benzene, which can account for such findings. Megaloblastic anemia and bone marrow depression have been associated with chlordane exposures. DDT and toxaphene are suspected human carcinogens [48,49]. The risks for aldrin and dieldrin as human carcinogens could not be determined by the International Agency for Research on Cancer because of insufficient human and animal data [48].
Diagnostic Evaluation
Serum and urine concentrations of these organochlorines are commonly measured by gas chromatography or mass spectrometry. In an obvious exposure, these measurements are academic and would not alter clinical management. There are no correlations between concentrations in body tissues and specific health effects. If the diagnosis is in doubt, these measurements can at least confirm or rule out the insecticide exposure. Although blood is commonly sampled for the detection of these chemicals, adipose tissue or human milk may be used as well [50]. The laboratory should be consulted regarding the availability of analytical methods for biological specimens other
than blood. An acute exposure can be determined by a quantitative comparison of parent compound to metabolite. Because DDT and aldrin are rapidly metabolized on systemic absorption, their elevated concentrations in the blood would support a recent exposure.
than blood. An acute exposure can be determined by a quantitative comparison of parent compound to metabolite. Because DDT and aldrin are rapidly metabolized on systemic absorption, their elevated concentrations in the blood would support a recent exposure.
Chlorinated hydrocarbons are radiopaque, and their radiopacity is directly related to the number of chlorine atoms per molecule. Thus, radiographs can assist in demonstrating aspiration pneumonia and gut burden.
Management
Rescue workers and health care providers must use proper equipment, such as gloves and gowns, to prevent unnecessary exposure to these chemicals when providing assistance to these patients [51]. Initial treatment of organochlorine exposure involves limiting further chemical absorption by the patient. The patient should be removed from the scene, disrobed, and thoroughly and repeatedly washed with soap and water. Washing should include hair and fingernails. The patient’s clothing and leather goods must be placed in a plastic bag and discarded because of the tenacious binding of these agents to leather. All wash water should be contained and discarded in a secure fashion.
The role of gastric decontamination depends on the clinical presentation. Immediately after an intentional ingestion and in asymptomatic patients without spontaneous emesis, gastric aspiration should be carefully performed with a small nasogastric tube. Activated charcoal should be administered soon after ingestion (preferably within 1 hour) because it can limit further gut absorption and enhance elimination by interrupting enterohepatic or enteroenteric circulation [27]. Also, cholestyramine may interrupt enteric circulation and enhance elimination. Chlordecone and chlordane undergo enterohepatic circulation, and cholestyramine is indicated in symptomatic patients. In a controlled trial, cholestyramine was administered as 16 g per day to symptomatic factory workers exposed to chlordane. After 5 months, chlordane fecal elimination was shown to increase by 3.3 to 17.8 times, with neurologic symptoms improving as concentrations declined. Milk- and oil-based cathartics should be avoided because their high lipid solubility can enhance gut absorption. Hemodialysis is not effective in enhancing elimination of these chemicals because of their high volume of distribution and protein binding [52]. Hemoperfusion is probably of no benefit [52].
Organochlorine-induced seizures are managed with benzodiazepines and barbiturates. Phenytoin has not been demonstrated to be more effective as an anticonvulsant than barbiturates and it may actually increase the incidence of these seizures [53,54]. For uncontrolled status epilepticus, muscle paralysis and general anesthesia may be necessary. Aggressive seizure control is warranted to limit further development of CNS damage, metabolic acidosis, hyperthermia, rhabdomyolysis, and myoglobinuric renal failure.
Respiratory distress due to bronchospasm is managed with humidified oxygen and nebulized bronchodilators. Parenteral administration of adrenergic amines is not recommended because it may potentiate myocardial irritability. Early administration of steroids and prophylactic use of antibiotics for pulmonary aspiration have not been demonstrated to improve patient outcome. The early use of antibiotics may predispose to the selective growth of other bacterial organisms.
After appropriate decontamination, asymptomatic patients with an oral exposure can be observed for 6 hours and then discharged if their clinical status remains unchanged. Patients presenting with cardiovascular, CNS, or persistent respiratory manifestations should be admitted for further therapy and observation.
Pyrethroids
Pyrethrum is a collection of naturally occurring insecticide esters from the chrysanthemum flower. The pyrethrin I ester has the greatest insecticidal activity and is subject to rapid environmental degradation. To enhance its effectiveness in commercial use, synthetic alternatives known as pyrethroids were developed that are more resistant to decay. These compounds are present in consumer products, from flea and tick removers for pets to topical pediculicides.
Pharmacology
The pyrethroids (including pyrethrins) delay closure of the sodium channel during the end of depolarization, with resultant insect paralysis. Piperonyl butoxide is commonly added to commercial preparations to inhibit insects’ ability to metabolize the pyrethroid and prolong activity. In mammals, these agents are relatively nontoxic because of the low concentrations and rapid mammalian metabolism. However, people who are allergic to ragweed may have hypersensitivity reactions to pyrethroids. The degree of this cross-sensitization has been reported to be as high as 46%. Pyrethroids have no effects on cholinesterase activity, and atropine and pralidoxime are not indicated in therapy.
Pyrethroids are readily absorbed from the GI tract. Dermal absorption varies depending on the type of agent and additive organic solvents. Systemic absorption is enhanced in the presence of petroleum distillates. These compounds are highly lipid soluble and largely metabolized by the mixed-function oxidase enzymes in the liver.
Clinical Toxicity
Poisoning from pyrethroids can result from inhalational, dermal, or oral exposures [42,44,55,56,57]. Nausea, vomiting, and diarrhea may occur after ingestion [44,57]. Neurologic manifestations and hypersensitivity reactions, including anaphylaxis, are the most common forms of systemic toxicity. Neurologic findings depend on the type and concentration of the pyrethroid and include paresthesias, muscle fasciculations, coma, and seizures [44,55,57]. Patients with an intentional ingestion of a mixture containing an organophosphate and a pyrethroid can present with predominant cholinergic manifestations [58].
Management
Treatment is very similar to that described for organochlorines (see previous discussion). GI decontamination may be appropriate, but there is no role for repeat-dose–activated charcoal and cholestyramine therapy because enterohepatic circulation has not been demonstrated for the pyrethroids. Hypersensitivity reactions, including anaphylaxis, should be managed with epinephrine, steroids, antihistamines, bronchodilators, and vasopressors, as indicated.
Anticoagulants
Bishydroxycoumarin (dicumarol), the first anticoagulant, was isolated as the hemorrhagic agent in sweet clover disease, a bleeding disorder that resulted from the ingestion of spoiled clover silage. Numerous congeners, such as warfarin (3-α-acetonylbenzyl-4-hydroxycoumarin), have since been synthesized and used as a rodenticide. Typically, for the bait to be effective, the rodent must consume it for 3 to 10 days; however, continuous feeding for 21 days may be necessary to achieve 100% mortality. As rodents became increasingly resistant, warfarin derivatives were introduced and have supplanted warfarin. These “superwarfarins,” or long-acting anticoagulants, include brodifacoum, difenacoum, and indanedione derivatives. The long-acting anticoagulants are 100 times more potent than warfarin and have a much longer half-life. Most anticoagulant rodenticide is packaged with cereal or other food products as bait, with the amount of rodenticide in the product varying from 0.025% to 0.005% per weight. Acute accidental or suicidal ingestion of a minimal amount of bait containing long-acting anticoagulants is unlikely to cause toxicity [59]. However, a “mouthful” of a long-acting anticoagulant ingestion in an adult human has been reported to cause significant coagulopathy [60,61,62].
Pharmacology
Warfarin and its derivatives are oxidized by mixed-function oxidases into inactive metabolites in the liver [63]. The plasma half-life of warfarin is 42 hours, with duration of action of 2 to 5 days [63]. The long-acting anticoagulants are concentrated in the liver and have extremely long half-lives; brodifacoum has a half-life of 120 days in dogs, 61 hours in rabbits, and 156 hours in rats [64,65,66]. The half-life of long-acting anticoagulants may be affected by the dose. The exact half-life of long-acting anticoagulants in humans is unknown, and because of significant interspecies variation, animal data cannot be extrapolated to humans. Case reports in human exposures have reported half-lives of 6 to 23 days for chlorophacinone and 16 to 39 days for brodifacoum [60,67,68,69,70]. Clinical coagulopathy may persist as long as 42 to 300 days [67,68,69,71,72,73,74].
These anticoagulants inhibit vitamin K 2,3-epoxide reductase and, to a lesser extent, vitamin K reductase. These enzymes are responsible for the cyclic regeneration of vitamin K [75,76]. Vitamin K is the active coenzyme responsible for activation of clotting factors II, VII, IX, and X, as well as anticoagulant factors protein C and protein S, by hepatic γ-carboxylation of the N-terminal glutamate residual of these proteins [75]. Once activated, vitamin K–dependent clotting factors can interact with calcium and phospholipids in the coagulation cascade [70]. Inhibition of vitamin K 2,3-epoxide reductase and vitamin K reductase depletes vitamin K and vitamin K–dependent clotting factors, resulting in coagulopathy and bleeding. The half-lives of vitamin K–dependent clotting factors are 7 hours for factor VII, 24 hours for factor IX, 36 hours for factor X, and 50 hours for factor II [63]. Because factor VII has the shortest half-life of the vitamin K–dependent clotting factors, increases in prothrombin time or international normalized ratio (INR) are not seen until 50% to 70% of factor VII is depleted. In a healthy person, this change occurs 24 to 48 hours after ingestion [59]. Clinical coagulopathy may not be evident for several days when the other vitamin K–dependent factors are also depleted, however [77,78].
Clinical Toxicity
The primary manifestation of poisoning is coagulopathy. The most common signs are cutaneous bleeding, soft-tissue ecchymosis, gingival bleeding, epistaxis, hematuria, and increased menstrual bleeding [61,79]. Gross hematuria, GI bleeding, hemoptysis, and peritoneal and diffuse alveolar bleeding may occur in patients with more serious poisoning [80,81,82,83]. Fatalities are uncommon and usually result from complications of intracranial hemorrhage [82,84].
Management
Gastric decontamination with activated charcoal should be initiated for acute ingestions. The most important laboratory studies are the prothrombin time and INR. Soon after an acute ingestion, values are expected to be normal; assays must be repeated at least 48 hours after exposure because of delayed coagulopathy [59]. Prophylactic vitamin K therapy can delay the onset of coagulopathy, but is not recommended as it may obscure the diagnosis and mandate prolonged coagulation profile monitoring, which might otherwise be unnecessary. Clotting factor analysis, particularly for factor VII, is a more sensitive and earlier indicator of coagulopathy [59]. Factor analysis does not offer more useful information in most patients with minimal ingestions, however. Occasionally, serum detection for warfarin and its derivatives has demonstrated unsuspected exposures in patients with coagulopathy of unknown cause [62,71]. In patients with coagulopathy, serial monitoring of warfarin derivative concentrations can assist in predicting the duration of coagulopathy and therapy [67].
The primary treatment of anticoagulant toxicity is vitamin K replacement [85,86]. Warfarin and its congeners have much less effect on human than on rat vitamin K reductase, thus allowing vitamin K rescue therapy for anticoagulant toxicity in humans. Because a single dose of vitamin K therapy cannot affect the prolonged toxicity of the long-acting anticoagulants, empiric vitamin K therapy is not recommended unless the patient has a coagulopathy. Vitamin K is not immediately effective in reversing coagulopathy; fresh-frozen plasma (FFP) administration is indicated in patients with significant bleeding diathesis (Table 141.3). Factor-specific concentrates have been demonstrated to decrease the time to correction of the INR in patients with a coagulopathy from warfarin toxicity faster than FFP [87]. The experience with these agents in the treatment of long-acting anticoagulant rodenticides, such as brodifacoum, is limited. Activated factor VII (FVIIa), FFP, and vitamin K have been used to treat brodifacoum toxicity [88,89]. In one of these instances, a product containing FVIIa and prothrombin complex concentrates (factor II, IX, and X) was used [88]. Some advantages of factor-specific concentrates over FFP include improved consistency in correction of the INR and decreased amount of fluid administered.
Only vitamin K1 (phytonadione) should be used because the other forms (K2, K3, and K4) are ineffective in the treatment of anticoagulant toxicity. Vitamin K1 can be administered orally, subcutaneously, intramuscularly, and intravenously. Intravenous administration has been associated with anaphylactoid reactions and death [90,91,92]. Furthermore, it offers no real advantage over other routes of administration. Intramuscular injection may cause hematoma formation in patients with coagulopathy. Subcutaneous administration of vitamin K1 is safe and effective. Oral vitamin K1 may be simpler and just as efficacious [93]. The oral vitamin K1 dose required to reverse coagulopathy is variable, but typically ranges from 100 to 300 mg per day, divided three to four times per day [61,66,80]. The amount
of vitamin K therapy must be titrated to clinical response, however. The duration of vitamin K therapy and coagulopathy is also highly variable, ranging from 40 to 300 days. When the patient’s INR has remained normal for several days after stopping the treatment, vitamin K therapy can be discontinued. The trend of the patient’s concentration of clotting factors during this period may assist the determination of this clinical endpoint. Various methods have been proposed to decrease the duration of coagulopathy, including administration of hepatic enzyme inducers such as phenobarbital [64,66]. There is no good evidence to support any of these therapies, however.
of vitamin K therapy must be titrated to clinical response, however. The duration of vitamin K therapy and coagulopathy is also highly variable, ranging from 40 to 300 days. When the patient’s INR has remained normal for several days after stopping the treatment, vitamin K therapy can be discontinued. The trend of the patient’s concentration of clotting factors during this period may assist the determination of this clinical endpoint. Various methods have been proposed to decrease the duration of coagulopathy, including administration of hepatic enzyme inducers such as phenobarbital [64,66]. There is no good evidence to support any of these therapies, however.
Table 141.3 Treatment Guidelines for Coagulopathy from Long-Acting Warfarin-Like Rodenticides in Patients with no Underlying Risks for Thromboembolism | ||
---|---|---|
|
Strychnine
The use of strychnine as a pesticide dates back to the sixteenth century, when an extract of the Filipino St. Ignatius bean (Strychnos ignatii) was introduced as a rodenticide in Europe. Strychnine was used as a tonic, cathartic, and aphrodisiac as late as 1970, and resulted in numerous deaths [94]. It is also found as an adulterant in illicit drugs, such as cocaine and heroin. The only “legitimate” uses of strychnine today are as a pesticide and in research study of neural transmission [94,95].
Pharmacology
Strychnine is rapidly absorbed through the nasal mucosa and orally in the small intestine. It undergoes hepatic oxidative transformation to unknown metabolites [96], and only 10% to 20% is excreted unchanged in the urine within 24 hours. The half-life of strychnine in humans is 10 to 16 hours, and the volume of distribution is 13 L per kg [97,98].
Strychnine competitively antagonizes postsynaptic glycine receptors at the spinal cord and, to a lesser degree, at the brain stem, cerebral cortex, and hippocampus [95,99,100]. Strychnine-binding sites overlap, but are distinct from glycine-binding sites at the glycine receptor [100,101]. Glycine receptors at the cerebral cortex and hippocampus are of a subtype insensitive to strychnine and are minimally affected [95]. The action of glycine is similar to that of γ-aminobutyric acid in that it enhances chloride ionic channel conduction, resulting in hyperpolarization of postsynaptic membrane and an increased threshold for neurologic transmission [95,102]. The highest concentration of glycine receptors is found at the ventral horn motor neurons in the spinal cord [102]. Glycine antagonism reduces neuromuscular inhibition, including reciprocal inhibition between antagonistic muscles, resulting in contraction of both flexor and extensor muscle groups [103]. The pharmacologic effect of strychnine is quite similar to that of tetanus toxin, which inhibits the release of glycine at postsynaptic neurons in the spinal cord [102,104].
Clinical Toxicity
The onset of toxicity is usually within 15 to 30 minutes of exposure. The lethal dose in adults is typically 50 to 100 mg, but it may be as little as 5 to 10 mg in children [94,105]. Diffuse muscle contractions and spasms are the primary manifestations of strychnine toxicity. Facial muscle spasms result in risus sardonicus (the “sardonic smile”) and trismus. Opisthotonos, abdominal muscle contractions, and tonic movements of the extremities may resemble convulsions. Because glycine has limited effects in the higher CNS centers, seizures are unlikely and mental status is normally preserved until the patient is hypoxic or moribund [94,105]. The extensor muscles appear to be more affected than the flexor muscles because they are the antigravity muscles and generally stronger [94,105]. Muscle contractions can be triggered or amplified by any stimulations, including auditory, tactile, and visual stimuli, and may lead to lactic acidosis, rhabdomyolysis, and hyperthermia [103,106]. Respiratory depression results from sustained chest and diaphragmatic muscle contractions and brain-stem depression. Death is related to respiratory depression, anoxia, and complications from significant muscle contractions [97,105]. The clinical manifestations of strychnine toxicity differ from tetanus infection in that the onset of symptoms in tetanus infection is more gradual and the duration of illness is more prolonged [104].
Management
Securing the airway, assisting breathing, and maintaining the circulatory system are the immediate goals in symptomatic patients. Electrolytes, acid–base changes, oxygenation saturation, renal function, urine output, and temperature must be monitored carefully in any symptomatic patient. GI decontamination should be performed in any case of suspected strychnine ingestion. Enhanced elimination by urinary manipulation has no effect because of minimal renal elimination [96]. Hemodialysis or charcoal hemoperfusion is ineffective because of the large volume of distribution.
Termination of muscle contractions prevents or reverses lactic acidosis, rhabdomyolysis, hyperthermia, and respiratory depression. Benzodiazepines are the initial agents of choice in attenuating musculoskeletal signs and symptoms [107,108,109]. Benzodiazepines enhance γ-aminobutyric acid effects in the spinal cord and may displace strychnine binding to glycine receptors [100,110,111]. Barbiturates also are reported to be useful in the treatment of strychnine toxicity. These agents may not be completely effective in patients with severe strychnine poisoning, however, and other agents such as propofol and adjunct nondepolarizing neuromuscular blockade may be required [98,105]. Strychnine toxicity usually resolves within
12 to 24 hours [96,103,112]. Supportive therapy should be continued until the patient is asymptomatic.
12 to 24 hours [96,103,112]. Supportive therapy should be continued until the patient is asymptomatic.
Sodium Monofluoroacetate
Sodium monofluoroacetate is frequently referred to as “compound 1080,” the number assigned to the compound during its initial development. It is the primary toxic constituent in the South African gifblaar (Dichapetalum cymosum), but it is also present in other plants in South America and Australia. Fluoroacetate is highly toxic to all mammals, and its use was banned in the United States in 1972 because of human fatalities and indiscriminate extermination of nontarget species. The congener sodium fluoroacetamide (compound 1081), also used as a pesticide, has mechanisms and effects similar to those of fluoroacetate. Prior to compound 1080’s ban in the United States in 1972, it was mostly used in livestock protection collars (tubular collars filled with pesticide, which is released when bitten by predators).
Pharmacology
Fluoroacetate appears to be minimally absorbed through skin but rapidly absorbed from the GI tract. It is metabolized to fluorocitrate in the tricarboxylic acid (TCA) cycle, with 12% of the ingested dose excreted in the urine [113]. In animals with relative resistance to monofluoroacetate, a hepatic defluorination system cleaves the carbon–fluoride bond to detoxify the compound [114].
Fluoroacetate is structurally similar to acetate and is incorporated into the TCA cycle with the assistance of acetyl coenzyme A. Fluoroacetate combines with citrate to form fluorocitrate in the TCA cycle [115]. Fluorocitrate inhibits aconitase and succinate dehydrogenase and disrupts the TCA cycle, halting cellular respiration and causing cell death [108,115,116]. Organs with high metabolic demands, such as the brain and heart, are immediately affected [117]. The lethal dose of sodium monofluoroacetate is 2 to 10 mg per kg [116].
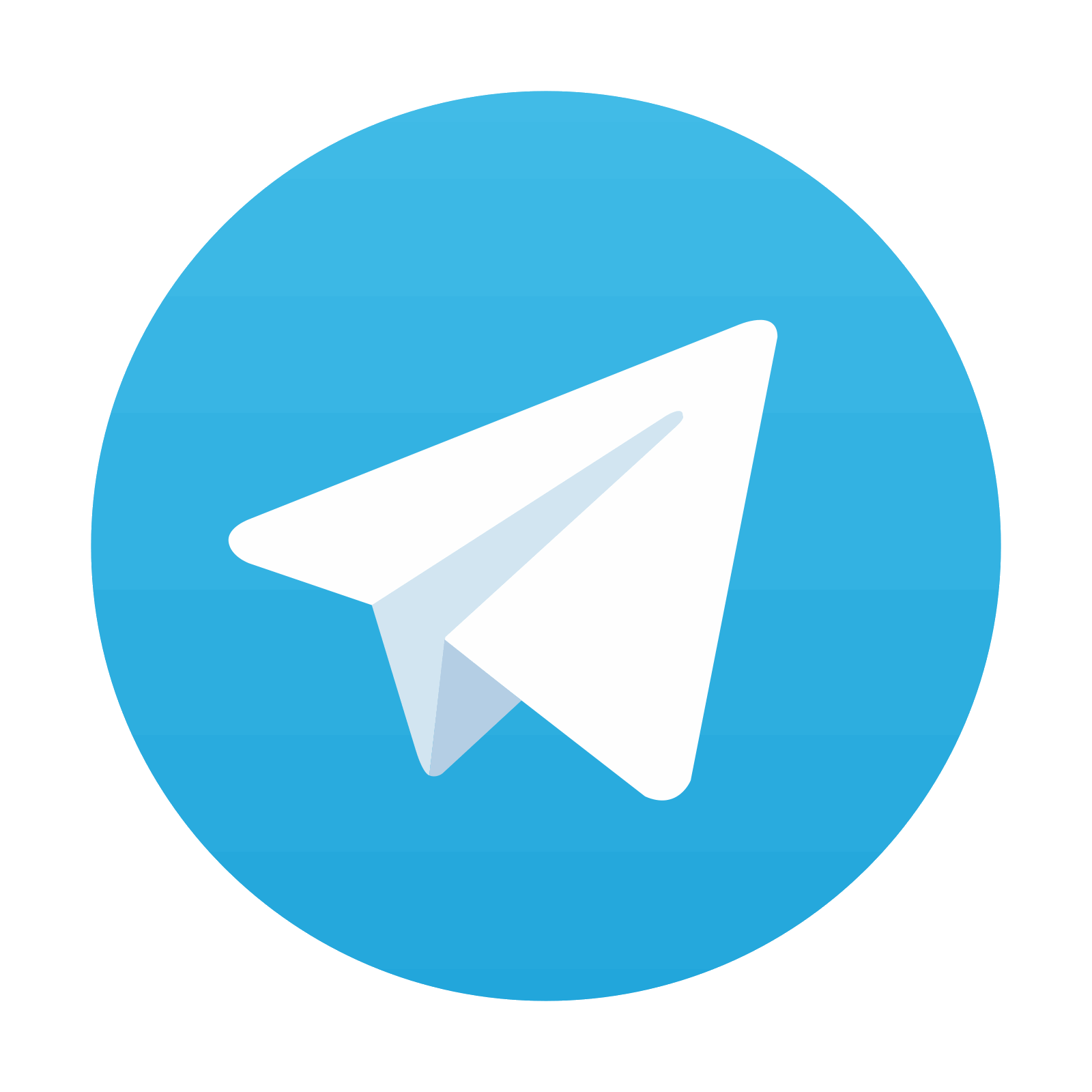
Stay updated, free articles. Join our Telegram channel
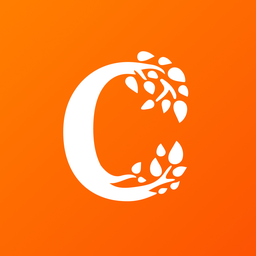
Full access? Get Clinical Tree
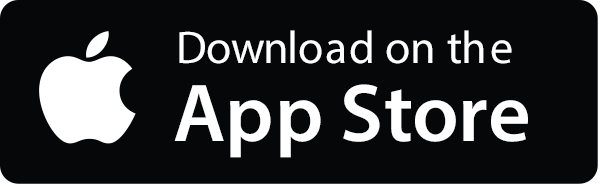
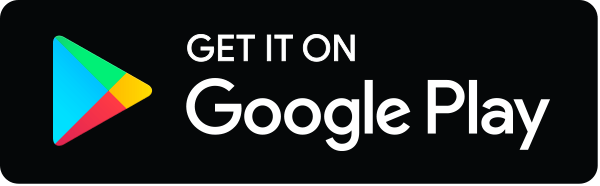