Chapter Outline
Intravenous Infusion Equipment 297
Anesthetic Gas Delivery Systems 297
Temperature Control 300
Equipment for Temperature Monitoring 300
Maintenance of the Normothermic State 300
Devices for Airway Heating and Humidification 301
Forced-Air Convective Patient Heating Systems 301
Radiative Patient-Warming Devices 301
Noninvasive Monitoring 301
Precordial and Esophageal Stethoscopes 301
Electrocardiography 302
Noninvasive Blood Pressure 302
Pulse Oximetry 302
Ultrasound Technology 302
Invasive Monitoring 302
Intraarterial Monitoring 302
Central Venous Pressure 303
Pulmonary Artery Pressure 303
Transesophageal Echocardiography 303
Routine and Special Airway Equipment 303
Mask 303
Oral Airway 303
Nasal Airway 304
Laryngeal Mask Airway 304
Endotracheal Tube 304
Laryngoscopes 305
The Lighted Stylet and Other Techniques 306
Respiratory Gas Monitoring 306
Specialized pediatric equipment is a recent phenomenon during the relatively short history of modern anesthesia. Parity of standards for pediatric and adult breathing systems was not established until 1963 by the American Society of Anesthesiologists and 1967 by the International Anesthesia Standards Committee.
Intravenous Infusion Equipment
Intravenous (IV) infusions are started for almost all anesthetized children, with the occasional exception of very short procedures such as myringotomy and tube placement or selected examinations under anesthesia. Intravenous administration sets most useful for pediatric patients usually have a method of quantifying and limiting the amount of fluid delivered; graduated drip chamber IV sets (“Buritrol”) are most commonly used. Pumps designed to deliver precise quantities of intravenous medications and provide continuous infusion for regional anesthesia are common in the pediatric operating room and are useful for total intravenous anesthesia techniques. Programmability of the pump for calculations in micrograms per kilograms per minute or conveniently available conversion tables are urged for practical daily use.
Intravenous filtering systems, whether for blood or air, should be readily available as the incidence of small ventricular septal defects or patency of the foramen ovale is not rare in the first year of life. Moreover, survivors of early cardiac surgery or children with palliated or unrepaired congenital heart disease with residual intracardiac communications may present for “routine” surgery. Small volume microaggregate filters have been developed but may lead to significant red cell destruction.
Anesthetic Gas Delivery Systems
Breathing Circuits
The circle anesthesia system or variations of Ayre’s T-piece ( Table 22–1 ) are the most common breathing circuits for pediatric patients ( Figure 22–1 ). For years, variations of the T-piece were recommended for children weighing less than 10 kg because of the decreased resistance to spontaneous breathing, the better “feel” of the rebreathing bag in the hand of the anesthetist, and the faster anesthesia induction and emergence times at higher fresh gas flows. Improvements in machine components such as lower resistance valves, reduced dead space at connections, improved CO 2 absorbent canister design, and the availability of capnography and changes in philosophy favoring controlled pulmonary ventilation in small children have rendered these arguments less durable. Likewise, less soluble inhalation agents such as sevoflurane make the influence of the breathing circuit less of an issue.
Advantages | Disadvantages | |
---|---|---|
Valveless, Variable F i co 2 Systems (Rebreathing and Non-Rebreathing) |
|
|
Circle Systems (0 F i co 2 Systems with valves) |
|
|

Normocarbia with a Mapleson breathing system is the result of a dynamic relationship between the fresh gas flow and the minute ventilation. When the fresh gas flow is high enough that rebreathing does not occur, the Paco 2 is determined by the minute ventilation. When the minute ventilation is significantly greater than the fresh gas flow, it is the fresh gas flow that becomes the principal determinant of the Paco 2 . Rapid respiratory rates may result in a higher F i O 2 because of the decreased time available for fresh gas inflow to wash out alveolar gas from the expiratory limb of the breathing system. Fresh gas flow rates for controlled and spontaneous ventilation with or without rebreathing have been validated with this system.
Bain and Spoerel’s modification of the Mapleson D circuit resulted in a coaxial breathing system with the fresh gas inflow hose within the exhalation limb. Verification of the integrity of the fresh gas inflow hose relies on the Pethick test, when a distended rebreathing bag collapses because fresh gas flow in the inner tube creates a Venturi effect in the outer tube. The Pethick test is not foolproof because the turbulence created by the rapid flow from the oxygen flush valve may not result in an adequate Venturi effect, and the bag may not collapse.
Cold, anhydrous anesthetic gases delivered to a breathing circuit adversely affect mucociliary transport and contribute to a higher incidence of tracheal tube obstruction because of accumulated dried or thickened secretions. Controlled partial rebreathing in Mapleson D or Bain systems can achieve an airway humidity of 24 to 26 mg H 2 O/L within 30 minutes without the use of external humidifiers or heat and moisture exchangers. Another alternative is low flow or closed circuit anesthesia using the circle absorption system (see Chapter 4 ).
Alveolar ventilation is influenced by the compression volume of the breathing circuit in relation to the tidal volume of the patient. Breathing circuits with large compression volumes will be “ventilated” far in excess of the volume delivered to the patient, which can become a significant consideration for small infants. Many pediatric circle systems are not only shorter, but also have a smaller radius of curvature of the tubing, which, according to LaPlace’s Law, renders them less distensible and thus further decreases compression volume.
Another important consideration for small children is the dead space of the breathing system. Dead space only exists when fresh and exhaled gases are mixed (i.e., at the Y-piece). Dead space no longer exists when fresh and exhaled gases are completely separated. The dead space of the Y-piece in a circle system can be decreased by the addition of a median septum ( Figure 22–2 ). The dead space of an elbow in a Mapleson D system can be decreased by the addition of a fresh gas delivery port within the elbow, such as the Norman elbow ( Figure 22–3 ).


Anesthesia Machines
There is no specific “pediatric” anesthesia machine; its design and checkout are identical whether used to anesthetize children or adults. Many anesthesia machines are equipped to provide air or nitrogen through the addition of a compressed air flowmeter and cylinder yolk for those circumstances when nitrous oxide-oxygen mixtures or 100% oxygen are to be avoided, for example, when anesthetizing premature or expremature infants, for prolonged abdominal surgery or procedures with a higher risk of accidental air embolism such as craniofacial reconstruction.
The proper size rebreathing bag for children may be calculated as a volume approximately equal to the patient’s vital capacity, or three times the tidal volume. The same calculation is applicable for the doubly open (Boothby-Lovelace-Bourbillion) rebreathing bags used on the Jackson-Rees modification of the Ayre’s T-piece.
Anesthesia Ventilators
Current anesthesia machine ventilators are typically pneumatically and electronically powered, ascending bellows, time-cycled, constant flow, and electronically controlled. The lungs of most children can be ventilated very well with such ventilators, notwithstanding differences in compressible volume of the ventilator bellows and breathing circuit. The availability and skilled application of appropriate clinical and instrument monitoring are crucial. The use of a pediatric bellows (300 mL maximum tidal volume) will lessen the compression volume of the bellows itself (4.5 mL/cm H 2 O compared with 1 to 2.5 mL/cm H 2 O).
Controlled ventilation is now much more precise for small patients. Machines now adjust for fresh gas flow and circuit compliance; recent models also allow sampling of the tidal volume measurement at the airway rather than at the expiratory valve, allowing for a better estimate of true tidal volume. Previously, tidal volume was usually measured at the expiratory valve. Thus the measured volume was not only the exhaled gas but also the gas compressed in the circuit during the previous inspiration.
Pediatric anesthesiologists have traditionally preferred pressure-controlled ventilation. Using a preset pressure and assessments such as ETco 2 and chest wall excursion, the anesthesiologist was assured of adequate ventilation with a reduced risk of barotrauma. Stayer et al found that flow generated on inspiration did not reach the set peak pressure when using short inspiratory times in a ventilator without constant pressure or piston-driven bellows. In addition, changes in chest wall compliance can greatly influence delivered tidal volume during pressure controlled ventilation, therefore, close attention must be paid to changes in ETco 2 and chest wall excursion.
Newer ventilators adjust for fresh gas flow and circuit compliance. In Drager machines the fresh gas is not continuously supplied during the expiration phase and is decoupled from the patient by a valve. Therefore, fresh gas flow does not influence the tidal volume during the inspiratory phase. Additionally the Drager Apollo ventilator measures the compliance of the breathing circuit during the initial check and compensates for it so as to deliver accurate tidal volumes. The Aisys by General Electric also measures circuit compliance and allows for compensation during the initial check. Some older ventilator models such as the Datex-Ohmeda Smartvent and the GE Avance do not have circuit compliance compensation but allow for flow sensors at the inspiratory valve, thus offering better volume control and measurement. If the ventilator has a decoupling mechanism and circuit compliance compensation, the anesthesiologist should feel comfortable using volume-controlled ventilation since this newer generation of ventilators is less influenced by fresh gas flow and circuit compliance. For the compliance compensation to be accurate, however, machine checkout must occur with each new circuit placed on the machine. Even with the new advances, examination of chest wall excursion, ETco 2 and blood gas analysis remain the gold-standard tools for assessing the adequacy of pulmonary ventilation.
Temperature Control
Equipment for Temperature Monitoring (also see chapter 19, patient warming devices )
Body temperature may be measured at a variety of sites. The skin temperature reflects the extent of peripheral perfusion rather than the core temperature. The temperature of the pulmonary artery’s blood can generally be regarded as the core temperature, although even the pulmonary artery may not provide “accurate” core temperature measurements during a thoracotomy or a sternotomy, especially when cold cardioplegia solutions are administered. Also, during a rapid blood transfusion, the pulmonary artery’s temperature may not reflect the core temperature of vital organs. Temperature measured at the distal third of the esophagus, the nasopharynx, and the tympanic membrane correlates well with pulmonary artery blood temperature. The temperature measured at the nasopharynx and tympanic membrane correlates well with temperature at the hypothalamus, and these measurements have special applicability during periods of decreased perfusion when induced hypothermia may be used protectively such as low-flow cardiopulmonary bypass and deep hypothermic circulatory arrest. The nasopharyngeal temperature is less accurate as a measure of brain temperature. The axilla may be a particularly important monitoring site for patients with suspected malignant hyperthermia because it drains blood from several large muscle masses. The rectal temperature is not as accurate a measure of a patient’s core temperature as was once thought since the probe may be insulated by feces. Also, cooler blood returning from the patient’s lower extremities may alter the measured rectal temperature. Conversely, heat-producing organisms in the gut may artifactually increase the rectal temperature. Sublingual sites are subject to the temperature-altering effects of the patient’s respiratory gas flow and any liquids that have been consumed. Urinary temperature correlates well with the core temperature when the flow of urine is high, but when the flow of urine is low, the cool blood returning from the lower extremities may lower the temperature.
Maintenance of the Normothermic State
Thermostat Control of the Ambient Operating Room Temperature
A neutral thermal environment is that temperature range at which a patient’s oxygen consumption and heat production at rest is minimal, yet the core temperature remains normal. As the environmental temperature decreases below this range, the body’s homeothermic strategy is to increase oxygen consumption and produce heat. If the operating room can be warmed while anesthesia is being induced, it will limit redistribution hypothermia. The operating room may then be cooled once the patient is adequately covered with drapes and blankets and rewarmed at the procedure’s end. When the operating room’s temperature is greater than 21° C, most adult patients will not become hypothermic. Infants and neonates, however, may require ambient room temperatures that are higher, approximately 26° C, to remain normothermic.

Intravenous Fluid Warming
The infusion of cold intravenous fluids, blood, or blood components may contribute substantially to the development of hypothermia. The temperature drop attributable to such infusions depends upon the volume of the infusate, the size of the patient, the initial temperature of the patient and the infusate, and the time over which the infusion is administered.
In an effort to prevent hypothermia, blood may be warmed before rather than during a transfusion. This is referred to as pretransfusion warming . This may be accomplished by placing the blood in a temperature-regulated warm water bath, by mixing the blood with saline that has been brought to normal body temperature, by wrapping the blood in a temperature-controlled warm water mattress or warm air blanket, or by placing prefilled syringes in an infant incubator. These methods must be used with great caution since blood may be contaminated by pathogens or damaged by overheating. In-line warming devices heat the fluids by passing them through modified intravenous tubing placed in either a water bath or between two electrically heated metal plates. The degree of heat transfer to the fluid is dependent upon the fluid’s flow rate, the initial fluid temperature, the warmer’s temperature, and the heat transfer capabilities of the plastic bags and tubing. Some in-line warming devices improve the heat transfer by using a countercurrent mechanism. There is a risk of conducting an electrical current through the warming fluid’s path when using water bath fluid warmers and so these devices should be properly isolated electrically. Dry-heat fluid warming devices consist of two heated plates through which a plastic bag of varying design is placed. The blood or other fluid to be infused runs through channels in the plastic bag, and heat is transferred to it from the metal plates.
The Prevention of Patient Heat Losses Via The Respiratory System: The Effect of the Breathing System
As water evaporates, cooling occurs. Such evaporative cooling of an anesthetized patient’s airways may be prevented by the humidification of the inspired gases; however, this will have a minimal effect on the body temperature of an anesthetized adult or a large child. The major reason for warming and humidifying the inspired gases delivered to anesthetized patients is to prevent the desiccation of the airway’s epithelium and the inspissation of secretions and to preserve normal mucociliary function. Since lower fresh gas flows may be used with a semiclosed circle anesthesia system rather than with the Mapleson system, heat and moisture may be better preserved in the former (see Chapter 4 ).
Devices for Airway Heating and Humidification
Active Airway Warming Systems
The active heating and humidification of respiratory gases may not prevent cooling of an adult patient’s body, but there may be an advantage to such heating for preserving normothermia in small infants and neonates. However, anesthesia circuit leaks, inadvertent hyperthermia, and bacterial contamination concerns have made kettle type of heating systems largely obsolete.
Passive Airway Warming and Humidification Systems
Passive airway warming and humidification systems are variously referred to generically as “heat and moisture exchangers” (HMEs) or “artificial noses” and are constructed of materials such as wool, foam, or methylcellulose, which are usually contained within a clear plastic cylinder. Condensation of expired water vapor occurs as the exhaled, humidified gases contact the cooler membrane’s surface. As the water vapor changes its phase from a gas to a liquid, heat is transferred to the membrane’s surface. During the subsequent respiratory cycle, the heat is transferred from the membrane to the inspiratory gas, vaporizing the water. The result is the partial conservation of the heat and moisture of the patient’s respiratory gases. For maximum efficiency, passive airway heating and humidification devices should be inserted while the patient is still relatively warm.
The Use of Blankets for Passive Patient Warming
Ordinary cloth blankets provide a means of passive thermal protection for patients by decreasing radiative and convective heat losses. Furthermore, they may reduce conductive heat losses if placed between the patient and a colder surface such as the operating room table. The effectiveness of a blanket in the prevention of a patient’s heat loss is directly proportional to the amount of the body’s surface area that is covered. An important strategy in conserving a child’s body heat is to cover the head with a blanket or a hat since the head may account for as much as 50% of the body’s total heat loss. If a hat is placed on a patient’s head, however, care must be taken to assure that the nares and mouth are not covered since exhaled gases may accumulate under the hat if a cuffless endotracheal tube is used. The accumulation of gases, such as oxygen and nitrous oxide that support combustion, under plastic or paper drapes, may increase the risk of a catastrophic fire, especially if lasers are used.
Blankets for Active Patient Warming
Thermal water mattresses may be used to either warm or cool a patient. They may be helpful in cooling a patient who has been inadvertently overheated, is febrile, or has developed malignant hyperthermia. For the maintenance of a patient’s body temperature, heated water mattresses are usually set to a temperature of 38° to 40° C. Heating blankets are most effective when they are placed on top of the patient where they can decrease convective heat losses. Burns may occur if a heating blanket is left in contact with the patient’s skin for a prolonged period of time, especially if there is poor blood flow to that area of skin. A layer of protective cloth, such as a cloth blanket or a sheet, should be used to diffuse the heat and to insulate the patient from direct contact with the warming element.
Forced-Air Convective Patient Heating Systems
Forced-hot-air convection is the most effective way of preventing the heat losses of an anesthetized patient. These devices can also effectively transfer heat and warm a hypothermic patient. The heat is initially transferred convectively from the air to the surface of the body, and then to the core by the patient’s own blood flow. The blanket is composed of polyethylene bonded to a tissue paper laminate. The warm air circulates in tubes formed by the interface of the plastic and paper. The tissue paper laminate contains slits through which the heated air may escape. A thermostat controls the temperature of the heated air circulating in a forced air patient heater. A forced air patient warming device can also be used to cool a patient by directing ambient operating room air over the patient at a high flow rate. Burns are rare with these devices, nevertheless, care should be taken to prevent the wrong side of the heating blanket from contacting the patient. Also, if the perfusion of the skin is limited (such as in a hypovolemic patient or a patient with minimal subcutaneous tissue), the heat may not be adequately dissipated. Heavy blankets should not be placed on top of a forced-air heating device’s covers since they may direct a jet of warmed air into direct contact with the patient’s skin.
Radiative Patient-Warming Devices
Infrared radiation is helpful when the patient is undraped, during the surgical preparation and the emergence from anesthesia. In the postanesthesia care unit, radiative heaters may also reduce shivering. Care must be taken to prevent burns by keeping the heating element at least three feet from the patient. Radiative heating lamps should be kept clear of plastic intravenous fluid bags and other combustible materials.
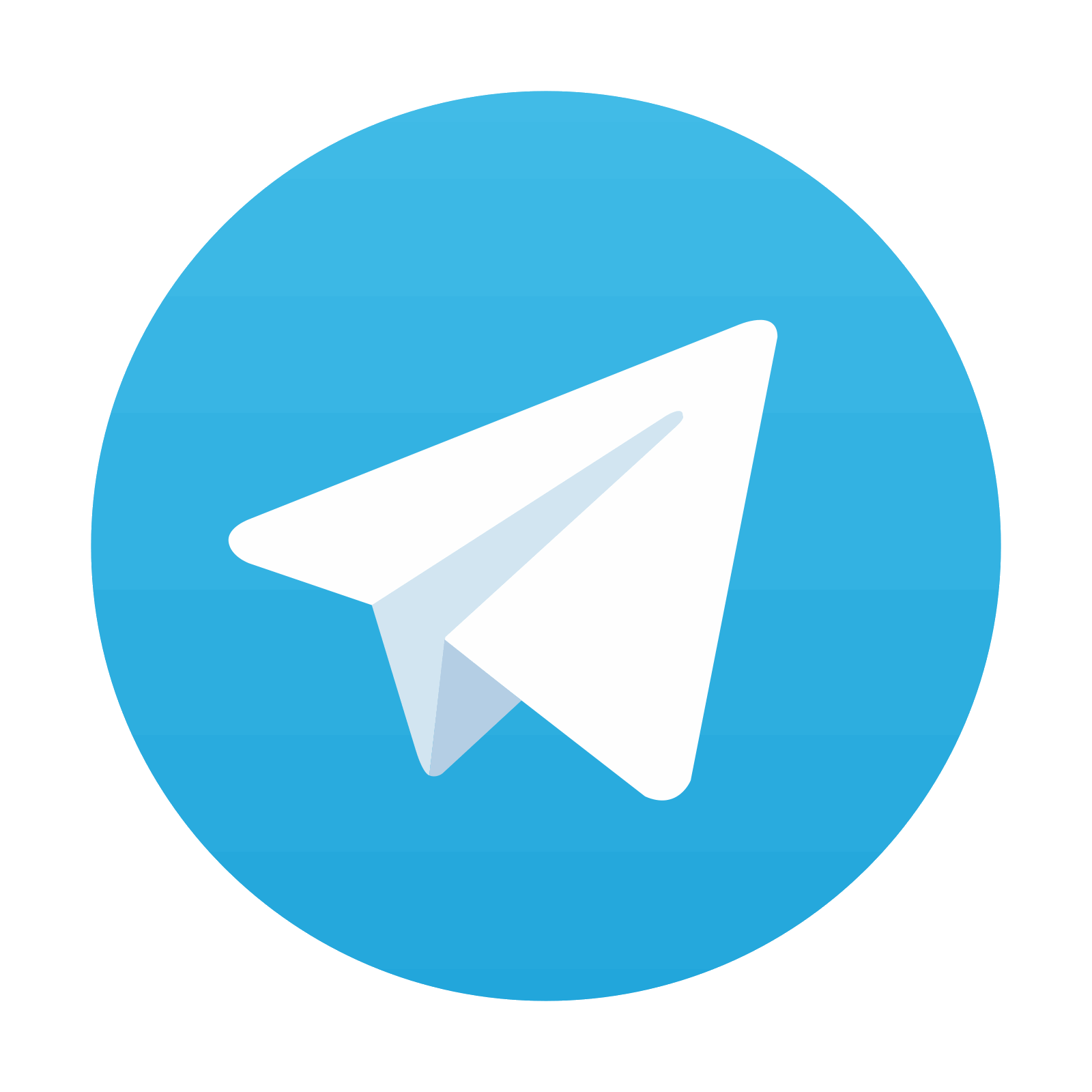
Stay updated, free articles. Join our Telegram channel
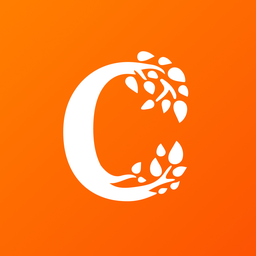
Full access? Get Clinical Tree
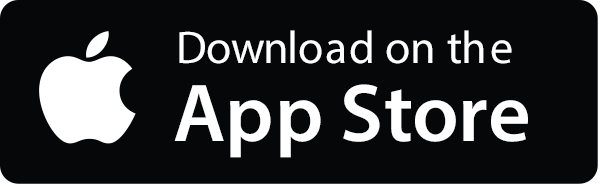
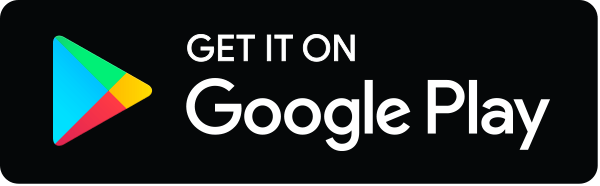