Key points
- •
Erector spinae, lumbar multifidus, quadratus lumborum, and psoas major are commonly investigated paraspinal muscles.
- •
Magnetic resonance imaging and computed tomography provide high-quality muscle images for the quantification of muscle morphometry, while ultrasonography is a low-cost noninvasive method capturing both static and dynamic muscle images.
- •
Decreased cross-sectional area and/or increased fatty infiltration of paraspinal muscles are likely to be associated with some spinal degenerative features (e.g., degenerative discs and facet joint osteoarthritis).
- •
Muscle atrophy or increased intramuscular fatty infiltration of lumbar multifidus are significantly associated with LBP/LBP-related disability although the relationship between other paraspinal muscles and LBP/LBP-related disability remain uncertain.
- •
Preliminary studies showed that certain trunk exercises (e.g., motor control exercises) can increase the cross-sectional area of erector spinae or lumbar multifidus. However, increased muscle size may not necessarily improve LBP symptoms.
- •
While paraspinal muscles are closely related to LBP, it remains unclear the optimal type and dosage of exercise for improving paraspinal muscle morphometry or clinical outcomes.
Introduction
As the lumbar spine is inherently unstable [ ], lumbar paraspinal muscles and abdominal muscles play an important role in maintaining dynamic stability and functional mobility of the lumbar spine (see Chapter 1 ) [ ]. A growing number of studies have shown that altered activity and morphology of paraspinal muscles are closely associated with low back pain (LBP) [ ] and lumbar pathologies (e.g., lumbar spinal stenosis [ ], disc herniation [ ], scoliosis [ ], degenerative lumbar kyphosis [ ]). Of the various posterior back muscles the erector spinae, lumbar multifidus, psoas major, and quadratus lumborum are commonly thought to be related to LBP based on their proximity to the lumbar spine.
Although electromyography (EMG) is the gold standard for evaluating back muscle activity [ ], both surface EMG and needle EMG are not commonly used in clinical practice. Surface EMG is affected by the cross-talk of adjacent muscles overlying the deep paraspinal muscles [ ], while needle EMG is an invasive technique that is not used routinely [ ]. Given that cross-sectional area (CSA) and density of muscles on medical images are closely related to muscle function and performance [ ], various noninvasive imaging techniques (e.g., ultrasonography, magnetic resonance imaging (MRI), and computed tomography scan (CT)) have been developed to assess the function of back muscles indirectly with high reproducibility [ ].
This chapter aims to provide a brief overview of the following: (1) the anatomy and function of the four muscles; (2) measurement of muscle morphology; (3) relations between the imaging findings of the muscles and LBP, LBP-related disability and lumbar pathologies; (4) clinical implications; and (5) future research.
Anatomy and function
Erector spinae
The intermediate layer of the intrinsic back muscles consists of the erector spinae (ES) muscle group, which is primarily responsible for the extension and lateral flexion of the trunk and neck (see Chapter 1 ). The ES consists of three thin columns of muscle: iliocostalis, longissimus, and spinalis, each covered in thoracolumbar fascia and distinguished by their points of attachment ( Table 15.1 ). The muscle groups extend caudocranially from the common origin (sacrum, pelvis, or spinous and transverse processes of T1 to L5) to the ribs, thoracic transverse processes, cervical spinous or transverse processes, or base of the skull. Specifically, they are found within grooves between the spinous processes and the angle of the ribs on each side of the vertebral column, coursing deep to the serratus posterior, rhomboids, splenius cervicis, and splenius capitis muscles [ ]. Only those muscles related to the lumbar function are discussed in detail in this section.
Muscle | Superior attachment | Inferior attachment | Function |
---|---|---|---|
Iliocostalis lumborum |
|
|
|
Longissimus thoracis |
|
|
|
Spinalis thoracis |
|
|
|
Lumbar multifidus |
|
|
|
Quadratus lumborum |
|
|
|
Psoas major |
|
|
|
The iliocostalis group is the most lateral of the ES ( Fig. 15.1 ) and consists of three muscles: iliocostalis lumborum, iliocostalis thoracis, and iliocostalis cervicis. Iliocostalis lumborum is the most inferior, originating from the ES aponeurosis and transverse processes of L1-L4 and traversing superiorly to the posteroinferior surfaces of the angles of ribs 3–6. Bilateral activation of the iliocostalis lumborum extends the thoracolumbar trunk, while unilateral activation mediates lateral flexion. Superior to the iliocostalis lumborum is the iliocostalis thoracis, which spans from the angle of ribs 7–12 to the superior borders of the angle of ribs 3–6 and the transverse process of C7. This muscle is also activated to extend and laterally flex the spine, instead solely acting within the thoracic region. The third and most superior muscle is the iliocostalis cervicis that mainly extends and laterally flexes the cervical spine. Each muscle of the iliocostalis group is innervated by the dorsal ramus of its respective underlying spinal nerves [ ].

Medial to the iliocostalis is the longissimus muscle group composed of the longissimus thoracis, longissimus cervicis, and longissimus capitis. Longissimus thoracis, the largest of all erector spinae muscles, primarily originates from the ES aponeurosis, though many fibers additionally stem from the transverse processes of the lumbar vertebrae. The superior attachment of the longissimus thoracis demonstrates a unique anatomic pattern. The upper fascicles insert into the tips of the transverse processes of T1-T4, while the lower fascicles demonstrate a bifid insertion to both the transverse processes and adjacent ribs of T5-T12 [ ]. Overall, this muscle serves in extension and lateral flexion of the thoracolumbar spine. Innervation is supplied by the lateral branches of the thoracic and lumbar posterior primary divisions of spinal nerves [ ]. The longissimus cervicis is located cranial to the longissimus thoracis, while longissimus capitis is the most superior of the longissimus group. They act together to control neck and head movement.
The third and most medial portion of the ES muscles is the spinalis group. Spinalis thoracis acts to extend or laterally flex the thoracic spine, while spinalis cervicis and spinalis capitis work together to extend, ipsilaterally flex, and/or rotate the neck [ ].
Of note the ES aponeurosis, often referred to as the common tendon, is a point of attachment for all inferior ES muscles. Attached to the lumbar spinous processes, supraspinous ligament, median sacral crest and dorsal iliac crest, the ES aponeurosis is found just superficial to the sacrum and blends with the thoracolumbar fascia covering affiliated muscles [ ].
Lumbar multifidus
The lumbar multifidi (LM) originate from the posterior sacrum, ES aponeurosis, posterior superior iliac spine, sacroiliac ligaments, and the mamillary processes of lumbar vertebrae ( Table 15.1 ) [ ]. They connect between two and four adjacent lumbar vertebrae, inserting at the base of the spinous processes. The muscle is innervated by the medial branch of the dorsal ramus of the spinal nerve of the same segmental level. In addition to having high muscle mass and very short muscle fibers (a low fiber length-to-muscle length ratio), the LM in the lower lumbar spine are characterized by a CSA over twice the size of any other muscle in the lumbar region [ ]. Muscle fascicles in LM have almost identical sarcomere length throughout the entire muscle length (the muscle fiber is approximately 21% of the muscle length) [ ]. Notably, those muscle fibers originating from the L1-L3 levels are significantly longer than those from T12, L4, and L5. The short muscle fibers indicate that the muscle is functioning on the ascending and plateau regions of the sarcomere length-tension curve, implying that as multifidus sarcomere length increases, muscle force also increases. Thus, the muscle becomes intrinsically stronger (a greater restoring/stabilizing force) as the spine moves into a forward flexion, a posture known to increase intradiscal pressure and trigger LBP in some people [ , ].
The architecture of LM enables packing of an enormous number of muscle fibers into a confined space along the lumbar spine. Such unique fiber arrangement enables LM to generate a large compressive force over a short distance for stabilization rather than mobilization of the spine [ ]. Notably, it provides optimal stabilizing joint forces to the spine throughout the full range of motion of the body. Functionally, when bilateral LM are activated, they extend the lumbar vertebral column, while unilateral contraction laterally flexes and contralaterally rotates the lumbar spine. Biomechanical research has estimated that LM as a whole can generate more than twice the amount of extension force than can be generated by any of the other lumbar extensors [ ]. LM work closely with transversus abdominis and pelvic floor muscles to maintain the stability of the lumbar spine prior to arm and leg movements.
Quadratus lumborum
The quadratus lumborum (QL) is a paired posterior abdominal wall muscle with its origin on the iliac crest and insertion on the transverse processes of the L1-L4 vertebrae and 12th rib ( Table 15.1 ) [ ]. Its location deep in the abdominal wall often places it among the back muscle grouping. The QL comprises three layers with muscle fibers that have different vectors: a thin superficial layer comprising iliocostal and iliothoracic muscle fibers; an intermediate layer comprising lumbocostal muscle fibers; and a posterior layer consisting of lateral iliocostal and medial iliolumbar fibers [ ]. These many vectors allow QL to serve a variety of functions. With ipsilateral contraction, it performs lateral flexion of the vertebral column and elevates the ilium, while bilateral contraction extends the vertebral column. The QL provides vertical stabilization of the pelvis, lumbar spine and lumbosacral junction. It additionally assists the diaphragm in inhalation and fixes the 12th rib during forced expiration. In coordination with the LM and ES muscles, the QL helps to create an antagonist force to the muscles of the abdomen [ ]. Although involved in several movements and stabilization of the lumbar spine, the magnitudes of the compressive forces exerted by QL on the lumbar spine, the extensor moment, and the lateral bending moment are each no greater than 10% of those exerted by ES and LM [ ].
Psoas major
The psoas major (PM) muscle consists of a superficial and a deep layer ( Table 15.1 ) [ ]. The superficial layer originates from the lateral sides of vertebrae T12-L4 and from the four adjacent intervertebral discs (L1/2-L4/5). The deep layer originates from the transverse processes of L1-L5 [ ]. A lumbar plexus separates the two layers [ ]. The PM runs inferiorly and joins iliacus muscles to form the iliopsoas, which runs across the iliopubic eminence and through the muscular lacuna to insert into the lesser trochanter of the femur. The muscle is innervated by the anterior rami of L1-L3 spinal nerves and can serve as a major hip flexor. The PM muscle fascicles from the vertebral body and intervertebral discs act as trunk lateral flexors, while muscle fascicles originating from transverse processes contract bilaterally to extend the trunk from a supine position [ ]. PM also serves as an intersegmental extensor in the mid lumbar region and a stabilizer by compressing the lumbar spine [ ]. The PM muscle is usually considered with iliacus muscle because it merges with iliacus as it passes through the pelvis and inserts into the femur. These muscles together form the iliopsoas muscle. As the iliopsoas muscle crosses the hip joint the contraction of the iliopsoas muscle causes hip flexion and external rotation.
Assessments on medical images
Different imaging modalities have been used to evaluate paraspinal muscle morphometry. Both MRI and CT scans provide 3-dimensional images of paraspinal muscles, allowing measurement of CSA, thickness, and volume of muscle (see Chapter 5 ). Images are taken with the patient remaining motionless in a decubitus position. In addition to the traditional T1- and T2-weighted MRI images [ ], various new MRI sequences (e.g., DIXON, mDIXON, IDEAL, FatSep, or WFS) have been developed to better distinguish fat and muscle tissues on medical images [ , ]. However, these new MRI sequences have yet to be used in routine clinical practice.
The CSA and fat infiltration rate are the standard parameters for evaluating paraspinal muscle morphometry on axial MR and CT images [ ]. To measure the CSA of a given muscle, the innermost or outer fascial boundary of the target muscle is traced manually using free (e.g., Image J or MiPAV), commercialized (e.g., OsiriX) ( Fig. 15.2 ), or customized software ( Fig. 15.3 ) [ , , ]. The software then reports the CSA size. However, as the total CSA of a muscle only represents the muscle size without considering the muscle composition, some researchers have suggested that the measurement of functional CSA (i.e., fat-free muscle mass) provides a better estimate of lean muscle and contractibility [ ]. As fatty tissues and lean muscles have different signal (pixel) intensity, functional CSA can be estimated manually by tracing the CSA of fatty tissues or by using various signal intensity threshold techniques of computer software to include only pixels in the lean muscle tissue range within the total muscle CSA [ , , , ]. In addition to the threshold method, intramuscular fatty infiltration can also be estimated by using the muscle-fat index [ ]. The index is the ratio of the average signal intensity of lean muscle tissue in a user-defined region of interest (ROI) of a given muscle to the average signal intensity of a user-defined intermuscular fat ROI. A lower ratio indicates more intramuscular fatty infiltration [ , ].


Although both manual and threshold approaches have been used in prior research to estimate lean muscle CSA or the extent of intramuscular fatty infiltration, even the latest muscle composition evaluation software requires the operator manually to define the ROI [ ]. That different researchers used diverse software programs to measure total or functional CSA of paraspinal muscles (e.g., built-in software of an MR scanner [ ], customized computer software [ , , ] , open-source software [ , , ], commercialized software [ ], computer-aided drafting software [ ], and picture archiving and communications systems workstations) [ , ], may partly explain some discrepancy in findings.
Additionally, the density of paraspinal muscles (in Hounsfield units) can be measured on CT images of the lumbar spine. Muscle density represents quantity and size of muscle fibers, as well as the general packing of contractile tissues [ ]. To estimate muscle density, a predetermined number of ROIs are selected from different locations of the target muscles on CT axial images. The average density is then calculated [ ]. Prior research has reported acceptable intra- and interobserver reliability for muscle measurement using CT and MRI [ , ].
Unlike MRI or CT, bright-mode ultrasonography captures 2-dimensional axial or sagittal images of paraspinal muscles at a lower resolution but with the benefit of dynamism ( Fig. 15.3 ). It is difficult to quantify intramuscular fatty infiltration using ultrasonography because of the difficulty in precisely distinguishing muscle, fat, and fibrosis on axial ultrasound images [ ]. Despite this disadvantage, ultrasonography is a reliable low-cost non-invasive technique for capturing real-time dynamic images of paraspinal muscles (especially LM) during contraction in a clinical setting [ , , ]. Specifically, an operator instructs a prone-positioned patient to lift up the contralateral arm and leg to induce automatic submaximal contraction of LM, while the corresponding parasagittal ultrasound images or videos of LM muscle at rest and during contraction are captured [ , , ]. The LM muscle thickness at a given lumbar level is measured from the inside edge of the echogenic connective tissues overlying LM muscle to the corresponding facet joint. By measuring the muscle thickness at rest and during contraction, the percent change in muscle thickness can be used as a surrogate to estimate the contractibility of a muscle [ ]. Theoretically, a stronger muscle contraction results in a greater change in muscle thickness during contraction.
Despite the advances in technology, multiple operational parameters can affect the quality of images acquired by different imaging techniques. The field strength of MRI, sequence type, acquisition time, acquired voxel dimensions and slice thickness can affect the quality of the resulting images. The method for defining the ROI can affect the measured morphometry and segmentation of paraspinal muscles on MR or CT images. The borders between LM and ES can be difficult to discern on axial images [ , ] especially with CT, given its lower resolving power in differentiating soft tissues compared to MRI [ ]. Similarly, muscle morphometry of LM as measured using 2-dimensional ultrasound may be influenced by multiple factors (e.g., the placement and contact pressure of an ultrasound transducer, patient position, vertebral instruction, types of contraction, the arm lift height during a contralateral arm lifting test, muscle extensibility, fiber orientation, and adjacent ES contraction) [ , ]. Therefore, it has been suggested that only the muscle thickness change measured during the isometric contraction of a prime movement task should be considered as muscle activity [ ]. Further, if a patient has hypertrophied erector spinae, the ultrasound transducer may easily slide during a contralateral arm lifting task. In short, standardized imaging, measurement procedures and reporting are needed to improve the reproducibility of findings and to enable comparison across studies.
Factors affecting muscle morphometry other than low back pain
A number of factors influence the morphometry (e.g., density and CSA) of paraspinal muscles. As is true for all skeletal muscles, older age, physical inactivity, and poor nutrition are associated with muscle atrophy characterized by a reduction in the size and number of muscle fibers [ , ]. Lower body mass index (BMI) is also associated with decreased CSA of ES, although muscle composition does not appear to be related [ ]. Similarly, age and sex affect the composition of paraspinal muscles [ , ]. Older age is associated with increased intramuscular fatty infiltration [ , ], and females generally exhibit smaller muscle volume and fiber size with a higher proportion of fatty infiltration of muscle compared to males [ ]. This trend is especially noted in lower spinal levels [ ]. In general, increased fatty infiltration is associated with greater muscle weakness, poorer function, decreased mobility, and potential spinal pathology [ , ]. Such changes in paraspinal muscle morphometry often do not affect both sides equally, and results in muscle asymmetry [ ]. Further, older age and handedness are also related to asymmetry in ES morphometry [ ].
Relations between muscle morphometry and other spinal degeneration
Given the critical role of the paraspinal muscles in stabilizing and mobilizing the spine, muscle degeneration (manifested as decreased CSA and/or increased intramuscular fatty infiltration) may be related to spinal degeneration [ ], although the precise nature of the relationship remains unclear (see Chapter 9, Chapter 10, Chapter 11, Chapter 12, Chapter 13 ). Decreased CSA and increased fatty infiltration of paraspinal muscle are associated with disc herniation [ , ], radiculopathy [ ], degenerative lumbar scoliosis [ , ], kyphosis [ ], and sway-back posture [ , ]. Increased fatty infiltration in the ES, specifically, is correlated with decreased intervertebral disc height and an increased risk of Modic change [ ]. Similarly, atrophic changes in the QL are seen in monosegmental intervertebral disc degeneration, although these changes are accompanied with unilateral atrophy of LM, ES, and PM muscles [ ].
The CSA and density of various lumbar paraspinal muscles are related to degenerative lumbar spinal stenosis (LSS) [ ]. Based on the findings of 345 people with and without LSS, Abbas et al. found that the mean CSA and density of ES and PM muscles at the L3 level in males with LSS were significantly greater than healthy counterparts after accounting for age and BMI [ ]. Similarly, the mean CSA of ES at the L3 level in females with LSS was significantly larger than that in healthy controls. Both males and females with LSS had significantly higher muscle density of ES, PM, and LM muscle at the L3 level than healthy controls after adjusting for age and BMI. Interestingly, as compared to the muscle density of ES or PM muscle at the L3 level, LM at the L3 level had the greatest between-group difference in muscle density [ ]. These observations contradicted previous findings that CSA of paraspinal muscles at the L5-S1 level of patients with LSS was significantly smaller than asymptomatic controls due to muscle denervation and/or disuse [ ]. This discrepancy may be due to increased activation of LM, ES, and PM at the L3 level to compensate for stenosis-related paraspinal denervation [ , ], LM muscle atrophy [ ], and instability at the lower lumbar level [ ].
Intramuscular fatty infiltration of LM is associated with facet joint degeneration in the lumbar spine. One study involving 150 individuals undergoing CT scanning of lumbar or abdominal regions showed that greater LM fatty infiltration was associated with more severe facet joint osteoarthritis [ ]. Similarly, another study involving 160 patients with acute (<30 days) or chronic nonspecific LBP (>3 months) also found that the degree of LM fatty infiltration was significantly associated with facet joint osteoarthritis at the L3-4, L4-5, and L5-S1 levels [ ]. Additionally, greater CSA asymmetry was associated with more severe facet joint degeneration at L5-S1 [ ]. These findings substantiate the notion that LM degeneration is closely related to the degeneration of other spine phenotypes.
Asymmetry in ES morphometry is related to disc height narrowing [ ]. A greater asymmetry in CSA of ES heightens the risk of altered facet orientation and tropism [ ]. As facet joints play a role in stabilizing the spine during compression, extension and rotation, facet tropism (i.e., asymmetry between right and left facet joint angles at the same vertebral level) permits improper sagittal rotation of the spine. Such disturbance in mechanical equilibrium may be linked to spinal pathologies including facet joint osteoarthritis [ , ], disc degeneration (disc narrowing) [ ], and degenerative spondylolisthesis [ , ]; all of which may contribute to LBP.
Greater anterior or lateral displacement of PM from the intervertebral disc at the L4/5 level is associated with more severe adult spinal deformity. Specifically, greater anterior displacement of the posterior border of PM110 from the tangent line of the posterior L4/5 intervertebral disc is associated with worsening of the sagittal alignment parameters (posterior tilt, pelvic incidence an lordosis) in older adults (see Chapter 16 ). Greater anterior displacement and lateral displacement of PM from the lateral border of intervertebral disc are associated with greater coronal Cobb angle of kyphoscoliosis. As early degenerative change in spinal alignment usually starts in the sagittal plane, the increased lumbar kyphosis disrupts fascicles of PM originating from the transverse process, leading to anterior displacement of PM. Likewise, more severe lumbar kyphoscoliosis is associated with increased apical vertebra translation and rotation, which increases tension force on the concave side at the L4/5 intervertebral disc level and disturbs the medial attachment of PM to the vertebral body and disc at the same level [ ].
Relationship between paraspinal muscle morphometry and LBP/LBP-related disability
Low back pain is the chief musculoskeletal disorder affecting 65%–85% of the general population at some point in life [ ]. Some studies suggest that paraspinal muscles (e.g., LM and ES) play a crucial role in the etiology, prognosis, and management of patients with LBP [ ]. The CSA and fat composition of paraspinal muscle have been reported to be related to LBP/LBP-related disability [ ], although inconsistent findings have also been reported [ ].
Compared to healthy individuals patients with LBP exhibit reduced CSA in paraspinal muscles, although this finding is more pronounced in the LM than ES [ ]. A recent systematic review with 15 articles on ES found conflicting evidence regarding the relation between the CSA of ES and LBP. Up to 8 included studies suggested no association between CSA of ES and LBP [ ]. The same review also concluded that there was insufficient evidence to support a significant relation between the fatty infiltration of ES and LBP [ ]. Many studies had moderate or high risk of bias [ ], necessitating future well designed longitudinal studies to clarify the role of ES morphology in the development of LBP.
Similarly many studies have investigated the relationship between LM morphometry (e.g., size or asymmetry) and LBP in different samples (e.g., ballet dancers, ballroom dancers, elite cricket players, patients with chronic LBP) [ , ]. Compared to healthy individuals, patients with acute or chronic LBP exhibit on average smaller functional CSA of LM at the lower lumbar levels [ , , , , ]. After adjusting for body size, the extent of muscle atrophy is related to the site of pain [ , , ] and the isometric voluntary contraction force [ ]. Patients with LBP or leg pain demonstrate focal LM atrophy as compared to asymptomatic counterparts [ ]. Increased fatty infiltration in LM is noted among people with LBP/LBP-related disability [ , ] or structural abnormalities of the lumbar spine [ , , ], even when total CSA is within normal limits [ , ]. Hyun et al. found that compared to healthy individuals, functional CSA of LM at the lower lumbar levels was significantly smaller in patients with disc herniation or unilateral radiculopathy. The decreases in muscle size may be attributed to LBP, disc herniation, and/or immobilization. Those with unilateral radiculopathy had significantly greater localized LM atrophy on the involved side (greater muscle asymmetry) [ ] than those with disc herniation, which might be ascribed to focal muscle changes after root denervation. A systematic review involving 14 studies related to LM concluded that the CSA of LM was negatively related to LBP [ ], and baseline CSA of LM in males predicted their LBP within the ensuing 12 months. However, the same review found conflicting evidence regarding the cross-sectional or temporal relation between fatty infiltration of LM and LBP.
In addition to total CSA and functional CSA of LM, patients with LBP (including chronic LBP) have been shown to manifest reduced thickness change of LM muscle during contraction as measured by ultrasonography compared to healthy individuals. [ , ]. However, research has also reported no significant difference in percent thickness change of LM during contraction between people with and without LBP [ ]. Interestingly, Wong et al. found that LBP patients who responded positively to spinal manipulation displayed significantly greater percent thickness change of LM at L4/5 and L5/S1 levels during contraction immediately after treatment compared to nonresponders [ ]. Similarly, Koppenhaver et al. found that compared to nonresponders, LBP patients who responded to dry needling displayed significantly greater increases in percent thickness change of LM at the L4/5 level during contraction 1 week after treatment [ ]. However, a systematic review concluded that there was conflicting evidence regarding the role of baseline thickness change of LM during contraction in predicting LBP after conservative treatment [ ]. Another systematic review also concluded that it was unclear whether temporal change in LM thickness characteristics were related to the corresponding changes in LBP-related clinical outcomes [ ].
A systematic review found conflicting evidence regarding the association between morphometry of QL and LBP. In particular, three studies reported a significant negative relation between CSA of QL and LBP, while five included studies showing no significant association between the two parameters [ ]. No prior study has investigated the relationship between intramuscular fatty infiltration of QL and LBP [ ]. The inconsistent relationship between QL morphometry and LBP does not exclude the muscle from contributing to the pathophysiology of LBP; however, it points to the pressing need for longitudinal studies.
The relation between PM atrophy and LBP is unclear. Kannaz et al. noted PM atrophy in patients with chronic LBP [ ]. Barker et al. found coexisting atrophy of LM and PM in patients with chronic LBP [ ]. Dangaria and colleagues also found a significant decrease in total CSA of PM in patients with disc herniation although it was unclear whether the asymmetry was due to atrophy on the sciatic side or hypertrophy on the other side [ ]. However, Danneels found no significant difference in total CSA or functional CSA of PM between people with and without chronic LBP [ ]. Likewise, Mayer et al. found no significant difference in CSA of PM between patients with and without spinal surgery [ ]. A recent systematic review identified 15 studies investigating the relation between total CSA of PM and LBP [ ]. These 15 studies adopted longitudinal ( n = 3), case-control ( n = 6), and cross-sectional ( n = 6) study designs with moderate to high risk of bias. They revealed conflicting evidence regarding the relation between total CSA of PM and LBP.
The relation between LBP and paraspinal muscles appears to be muscle specific. Gildea et al. revealed that ballet dancers with LBP had smaller LM CSA in the lower lumbar levels than asymptomatic counterparts, but not in the ES, QL or PM muscles [ ]. Danneels and coworkers found that compared to asymptomatic controls, chronic LBP patients demonstrated significantly smaller functional CSA of LM at the L4 level, but no between-group difference was noted in the lumbar ES or PM muscles [ ]. Similarly, Bouche et al. found that after normalizing the functional CSA of paraspinal muscles to the CSA of the L3 vertebral body, the normalized functional CSA of LM from L3 to L5 levels of lumbar discectomy patients with postoperative pain were significantly smaller than those of postoperative asymptomatic counterparts [ ]. However, only the normalized functional CSA of ES at the L3 level in painful patients was significantly smaller than pain-free patients [ ]. Interestingly, the functional CSA of PM in pain patients was not significantly different from pain-free patients [ ]. However, Arbanas et al. found that patients with LBP displayed significantly larger CSA of PM at the L3-4 and L4-5 than healthy controls [ ], suggesting that PM hypertrophied to stabilize the lumbar spine. These observations indicate selective atrophy of deep stabilizing muscles in patients with LBP.
Collectively, although some studies suggest that people with LBP [ , , , , ], disc pathology [ , ], degenerative kyphosis [ ], and spinal stenosis [ ] had significantly smaller CSA or lower muscle density of LM, PM, or ES at lower lumbar levels [ , , ], others reported no association between total CSA, asymmetry or muscle thickness of LM [ , , ] and LBP [ , , ]. Such inconsistency may be ascribed to the following: (1) multifactorial causes of LBP; (2) individual variation in muscle condition (e.g., physical fitness); (3) different imaging methods; and (4) divergent muscle parameters (e.g., total CSA, functional CSA, muscle asymmetry, density) at different lumbar levels or locations.
Exercises for restoring morphometry and their effects on LBP
Although most cases of LBP are resolved without intervention, chronic LBP may require paraspinal muscle stabilization and strengthening exercises to restore muscle morphometry and alleviate symptoms. Participation in an exercise program leads to increased muscle strength due to neural drive [ , , ], increased muscle density due to muscle fiber hypertrophy [ ], and increased CSA [ ]. While isometric exercises can enhance core stiffness [ ], incorporation of dynamic exercises into the rehabilitation regimen allows greater dynamic muscle strength gains [ ] and can be gradually modified to increase in intensity as rehabilitation progresses [ ].
For the ES, a recent electromyography study by Catalayud et al. found the greatest activation was achieved during the “squat” and “bird-dog” (from hands and knees, simultaneously raise contralateral arm and leg) exercises. Both exercises were well-tolerated by patients with LBP and can be easily customized to suit individual ability [ ]. The restoration of ES morphometry is an important element of rehabilitation because paraspinal muscle strengthening can lower the risk of LBP recurrence [ ].
Muscle strengthening increases the CSA and density of LM muscles [ , , ]. Many randomized controlled trials have been conducted to compare the effects of different exercises (e.g., motor control exercises [ ], McKenzie exercise [ ], general back exercises [ , ]) on improving the morphometry of LM muscles in patients with chronic LBP. Of various exercises, motor control exercises have shown significantly larger increases in the CSA of LM as compared to no treatment [ , ] or physiotherapy alone [ , ]. Likewise, compared to McKenzie exercise, motor control exercise induces a significantly greater increment in contracted muscle thickness of LM muscles as measured by ultrasonography (a proxy indicating an improvement in muscle contraction) [ ].
Different combinations of motor control exercise and conservative interventions had different effects on the CSA of LM [ , , ]. Interestingly, although several back muscle exercises have shown significant post-intervention increase in resting thickness of LM (a potential indication of muscle hypertrophy) [ , , ], no specific exercise has been found to be better than others. As the effects of muscle strengthening are affected by the frequency, intensity, and compliance of exercises, the inconsistent results may be ascribed to factors other than the type of prescribed exercise alone.
While one study found that LBP patents who showed segmental hypertrophy of LM after motor control exercises reported a 50% decrease in pain [ ], only a paucity of studies directly investigated the relationship between post-treatment change in morphometry and LBP or LBP-related disability. Two studies showed post-treatment increase in the resting thickness or CSA of LM to be unrelated to LBP intensity or LBP-related disability [ , ]. Another longitudinal study of fighter pilots found that baseline CSA of PM and paraspinal muscles (LM and ES) was unrelated to LBP at 5-year follow-up [ ]. However, as these studies had a small sample size [ , ], future studies are warranted to clarify the relation between baseline or change in morphometry of LM and clinical outcome.
Resistance training and motor control exercises have been shown to be a critical component for increasing the stability of the lumbar spine [ ]. General rehabilitation exercise for the trunk muscles has been shown to increase the CSA of all trunk muscles (except the QL at L1 and L2 levels) without generating potentially harmful compressive forces through the spine [ ]. The QL can be targeted directly by exercise that maintain the spine in a relatively neutral position. Imai et al. compared five exercises thought to target QL and PM (i.e., elbow-toe bridge, hand-knee bridge, elbow-knee bridge, side bridge, and knee raise) in healthy individuals. Side bridge exercise was found to cause the greatest recruitment of the QL [ ], while elbow-toe bridge exercise elicited the greatest PM contraction in healthy individuals. Future studies should determine whether the same effects would be applicable to patients with LBP.
Clinical implications from the relationship between paraspinal muscles and LBP
The existing literature presents conflicting evidence regarding the relationship between the muscle morphometry of ES, PM, or QL (including CSA, fatty infiltration, or stiffness) and LBP, as well as contrasting findings in LM [ , , , ]. However, as the weakness of back muscles is a known risk factor for LBP recurrence [ , , ] and improved back extensor strength is associated with reduced LBP intensity [ ], it may well be that improving the activation and strength of paraspinal muscles by therapeutic exercise is beneficial for symptoms. Clinicians may wish to check for muscle atrophy alongside the routine evaluation of disc herniation on MRI and should educate patients with LBP regarding the importance of performing back strengthening exercises and adopting an active lifestyle to reduce the risk of back muscle weakness or disuse atrophy.
As posterior paraspinal muscles play an important role in the mobility and dynamic stability of the lumbar spine, it is important to preserve these muscles during standard posterior midline surgical approaches. Some open surgical procedures can disrupt the neurovascular supply to paraspinal muscles, remove the spinous processes alongside posterior paraspinal muscles, and compress muscles after prolonged retraction. The consequence of this disruption is evident in the histological and biochemical changes to back muscles [ , ], significant functional deficit [ , ], and chronic/regional back pain syndrome [ ]. Therefore, minimally invasive spine surgery may be considered as an alternative to open surgical procedures to minimize damage to back extensor muscles [ , , ]. Future studies should also investigate whether protecting back extensors during minimally invasive surgical procedures yields better clinical outcomes.
As an early detection of abnormalities in muscle morphometry or function allows early preventive intervention (e.g., lifestyle modification, adjusted training, or appropriate therapeutics), novel MRI sequences can be used. Chemical shift encoding-based water–fat MRI or DIXON MRI can be used effectively to assess fatty infiltration in paraspinal muscle, which is highly related to muscle strength [ ]. By incorporating new MR and conventional MR sequences, clinicians may begin to detect muscle abnormalities relatively easily and promote early treatment.
Future research
There is limited evidence regarding the role of altered morphometry of various paraspinal muscles in the development or prognosis of LBP, so future large-scale prospective studies are warranted to determine whether muscle changes are a consequence of spinal degeneration or a cause/result of LBP. Additionally, prospective studies should investigate whether baseline characteristics of paraspinal muscles in people with and without LBP can predict the trajectory or chronicity of LBP. Researchers may also adapt advanced deep learning and machine learning tools to investigate the relative contributions of different muscle characteristics to various spinal pathologies [ ].
While the causes of LBP are multifactorial, the quality and volume of paraspinal muscles may be affected by multiple factors (e.g., age [ , ], gender [ ], BMI, physical activity level, degeneration of other spinal structures [ , , ], and spinal curvature [ , ]). Large-cohort prospective studies should be conducted adopting standardized methods to establish representative normative morphometry data by demographic group. The findings could be used for the following: (1) identify abnormality or degenerative change in paraspinal muscles in people with or without LBP; (2) explore whether changes in morphometry of paraspinal muscles are related to the severity of lumbar symptoms; and (3) evaluate the efficacy of various rehabilitative exercises on muscle morphometry and LBP.
Additionally, it remains unclear whether certain types and/or regimens (i.e., frequency, duration, and intensity) of exercises would optimize the strength of various paraspinal muscles. Future research should determine the effectiveness, if any, of different therapeutic exercises in improving paraspinal muscle function and clinical outcome among patients with LBP. Advanced MR sequences (e.g., chemical shift encoding-based water–fat MRI) may be used to detect subtle post-treatment change in intramuscular fatty infiltration [ ]. Subgroup analyses may, if adequately powered, determine differential responses of patient subgroups to various exercises so that ultimately personalized treatment regimens may be prescribed.
There is at present no consensus regarding a standardized imaging procedure and/or method for quantifying lumbar paraspinal muscle morphometry/quality. Prior research used manual, semiautomatic, or automatic segmentation methods to define intramuscular fatty infiltration [ ], making comparison of results among studies difficult. As the modeling of muscle activity or tensile properties also relies on accurate measurements of fat and muscle tissue, it is paramount to adopt a standardized assessment protocol to deepen the understanding of the effects of intramuscular fatty infiltration on health. An international consortium of multidisciplinary clinicians and scientists should be formed, therefore, to establish standardized methods for quantifying muscle composition and morphometry using different types of images (e.g., MR and CT images) so that meaningful comparisons of lumbar paraspinal muscle quality across studies can be conducted.
References
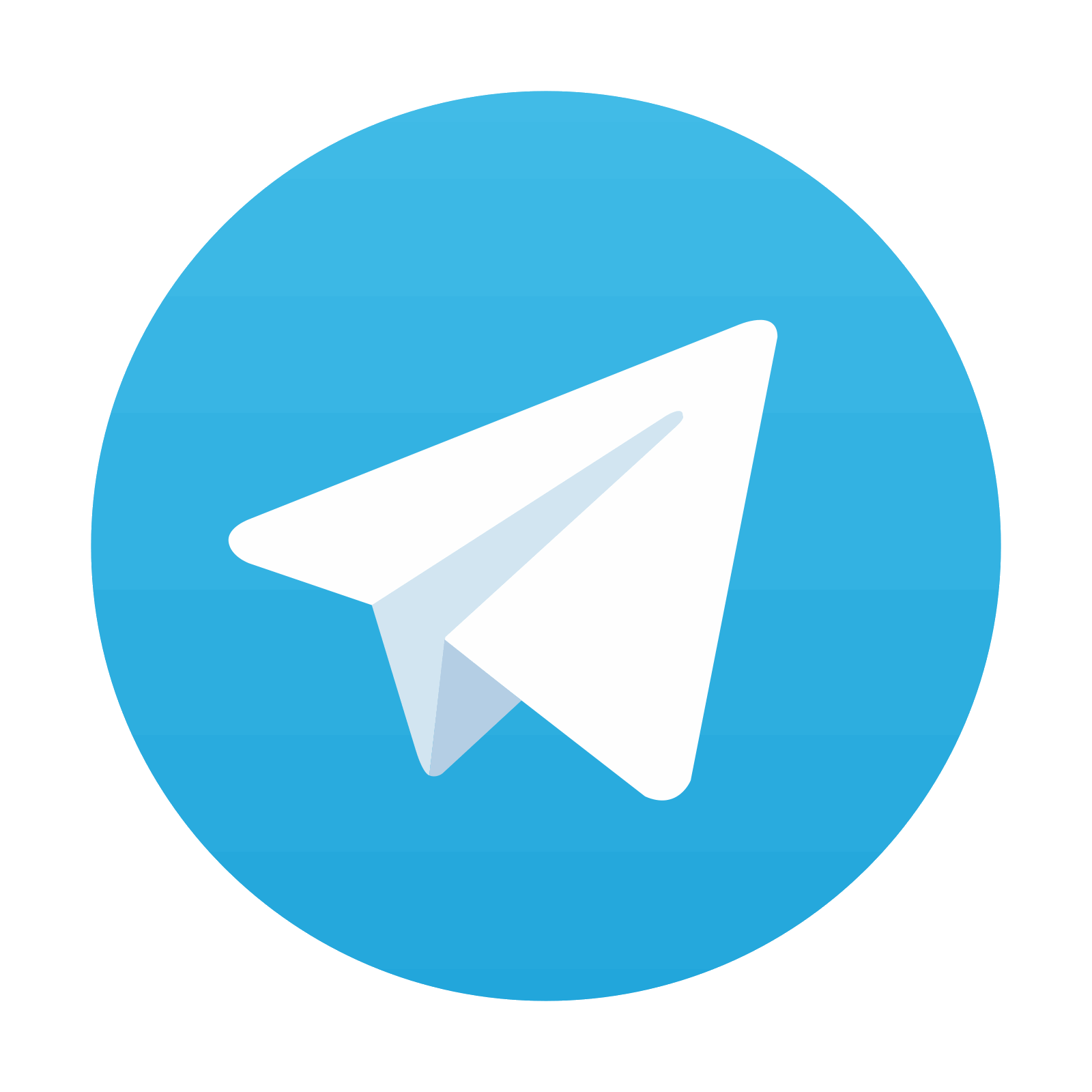
Stay updated, free articles. Join our Telegram channel
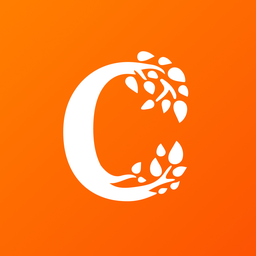
Full access? Get Clinical Tree
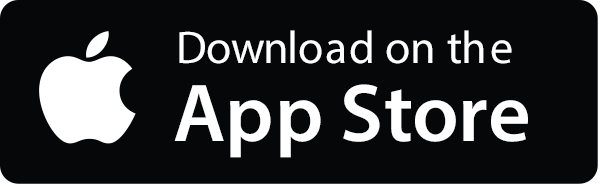
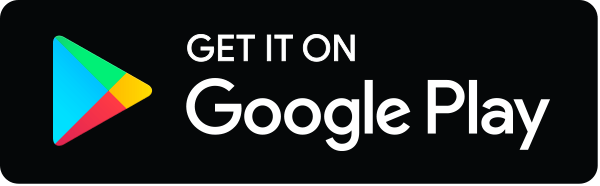
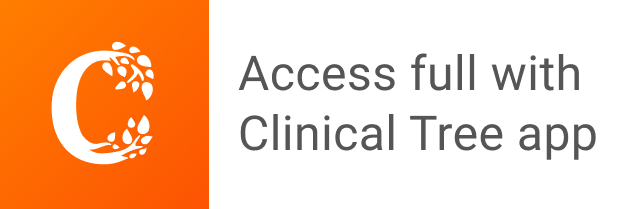