Fig. 27.1
Effects of pain and concurrent cognitive demand on SI activity. (a) Event-related average for primary somatosensory/motor cortex. Shaded gray area shows the duration of the condition. The SI/MI region whose activity is plotted is shown in axial brain image. (b) Modulation profile for SI/MI. This plot shows the influence of pain, cognitive load, and the interaction of pain and cognition. For example, looking only at the cyan line (T0, or minimal cognitive load), increasing pain (P2 (moderate pain) vs. P1 (mild pain) vs. P0 (non-painful stimulation)), activity in this region increases. This effect is significant across all task difficulty levels (T0–T3 (where T3 represents the highest cognitive demand)). However, the asterisks indicate that compared with P2T0, both P2T1 and P2T3 have significantly (P < 0.01) reduced activity. In other words, SI/MI moderate pain-related activity is significantly reduced by the easy (T1) and difficult (T3) cognitive tasks. Beta values are created from the general linear model analysis and represent the estimated fit of the data to the predictive model (from Seminowicz and Davis (2007) (Cerebral Cortex), used with permission)
Immersive virtual reality (VR) distraction is one particularly intriguing application of distraction that is designed to provide multisensory input (e.g., visual, aural, tactile) while blocking visual and aural input from the immediate environment. Several studies have suggested that immersive VR is effective in reducing clinical pain reports in both experimental settings and with patients undergoing painful procedures for burn injuries (Malloy and Milling 2010). New work is just beginning to explore the neural correlates associated with the use of immersive VR for pain reduction.
In one such study, Hoffman et al. (2007) assessed the impact of immersive VR on both subjective pain ratings and fMRI pain-related brain activity in response to thermal pain stimulation in healthy volunteers. This study compared three experimental conditions to examine the analgesic effects of immersive VR: immersive VR distraction alone, opioid alone, immersive VR distraction + opioid, and no treatment. Results indicated that use of both immersive VR distraction and opioid medication either alone or in combination generally resulted in lower subjective pain scores and lower pain-related brain activity compared to no treatment. Specifically, compared to the no treatment condition, immersive VR distraction alone significantly reduced pain-related brain activity in the insula, thalamus, and SII, while opioid alone reduced pain-related brain activity only in the insula and thalamus. When comparing the immersive VR distraction + opioid condition to other groups, the combined condition produced lower pain-related brain activity in the ACC and insula compared to the no treatment group, lower levels of pain-related brain activity in the SII compared to both the opioid alone group and the no treatment group, and lower levels of brain activity in the thalamus compared to the immersive VR distraction alone group and the no treatment group.
The study by Hoffman et al. (2007) found that participants in the immersive VR distraction + opioid group demonstrated reduced subjective (i.e., pain report) and objective (i.e., pain-related brain activity) pain markers compared to participants in the opioid alone group. The results of this study are important because they suggest that patients undergoing a painful medical procedure may receive additional pain relief from the use of distraction techniques such as immersive VR. This could be particularly important in instances where the traditional use of pain medications (i.e., opioids) for acute pain relief is not possible or desirable or is not providing adequate pain relief. The use of VR may be particularly useful because past work has shown that it has positive effects across the lifespan (i.e., both in children and adults). While this work was done in healthy volunteers in a laboratory setting, it would be interesting to understanding how this distraction technique would impact brain activity in patients with clinical pain or in patients undergoing a painful medical procedure.
In another recent study, Richter et al. (2010) employed a unique study design to examine brain activity during attention and distraction in response to pain stimuli. The aim of the study was to investigate unique brain activation in response to pain-related words compared to negative, positive, and neutral words during two attention tasks (i.e., imagination, distraction). These investigators aimed to determine if brain activity in response to pain stimuli is distinct from a general negative response and/or increased arousal. Of particular relevance to attention and distraction as pain modulators, participants’ brain activity was measured in response to paying close attention to the meaning of the words and when distracted from the meaning of the words. In the attention task, participants were asked to imagine a situation or sensation associated with the particular word. In the distraction task, participants were distracted from the word itself by being instructed to count the vowels of the words and be prepared to report them after the task. When participants were asked to focus their attention on the meaning of the words, increased brain activity was found in the DLPFC, inferior parietal gyri, and precuneus when processing pain-related words compared to the other words. When participants were asked to use a distraction task (count the vowels in the words), decreased activation was demonstrated in the dorsal anterior cingulate and increased activation was demonstrated in the subgenual ventral anterior cingulate when processing pain-related words compared to the other words. The results suggest that activations of pain-related brain regions are strongly modulated by attention and distraction processes and that the processing of pain-related words is specific to pain stimuli and not simply a result of negative response or increased arousal.
These results provide additional information regarding brain activation involved in the phenomena of attention and distraction to pain. The investigators suggest that their results indicate that the perception of pain-related words changes the central nervous processing associated with the cognitive dimensions of pain. This processing may be of particular importance for the effects of distraction in individuals facing acute pain due to medical procedures and/or chronic pain due to a chronic condition. The results support the notion that the words used by an individual can impact the pain experience. For example, how patients and/or healthcare professionals talk about pain may have the potential to increase or decrease patients’ levels of pain. An interesting direction for future research would be to examine how common types of pain-related language (e.g., support, reassurance, instructions, descriptions) used by health professional, caregivers, and even the patient influence pain-related brain activity in patients dealing with pain. If pain words have a clear effect on pain-related brain activity, protocols could be developed to coach individuals to alter how they talk about pain language so as to reduce negative effects.
Effect of Expectation and Hypnosis
Expectation. Heightened pain expectation, or anticipation of pain, is in and of itself adaptive in that people can remove themselves from harm’s way. However, heightened pain expectation can also result in increased fear and anxiety that are not only distressing, but can lead to more distress and to more pain (Crombez et al. 1999). Patients receiving palliative care have often experienced a myriad of painful medical interventions and may be particularly vulnerable to the deleterious effects of pain expectation. Empirical work has shown that high pain expectation or anxiety before a medical procedure can lead to increased postprocedural pain (Schupp et al. 2005). High levels of pain expectation can also be problematic when they lead to behavioral avoidance of situations associated with pain (Vlaeyen et al. 1995). Investigators have begun to use innovative methods of research to examine differences in brain activity in response to expectation of pain and actual pain stimuli.
In a study using fMRI, Ziv et al. (2010) assessed brain activity before and during cued or uncued thermal pain applied to the wrist. These investigators were particularly interested in examining the differential brain activity in the amygdala and hippocampus to cued and uncued pain stimuli. They also examined the relationship between anxiety-related personality traits and differences in pain reports to cued and uncued pain stimuli (i.e., pain expectancy scores) and how these two constructs (i.e., anxiety, pain expectancy) were associated with increased brain activity. Twelve individuals (seven female, mean age = 25) received a sequence of twelve intense pain stimuli. Half of the stimuli were cued with the words “Get Ready” imaged in red on a computer screen and half of the stimuli had no cue. Participants rated the pain intensity of the thermal stimuli using a 10-point visual analog scale (VAS) after each stimulus. This study had a number of interesting findings. First, on average, when the pain stimuli were preceded by a cue, noxious stimuli were experienced as more painful relative to unexpected (i.e., no cue) noxious stimuli of the same intensity. Second, the investigators found that the amygdala was more active during trials in which a cue preceded delivery of the pain stimulus and that hippocampal activity was increased (compared to baseline) during both cued and uncued trials.
Third, participants who scored higher on the personality trait of anxiety showed increased activity in the amygdala when expecting pain (i.e., after the cue and prior to the stimulus application). Participants who had higher pain expectancy scores (i.e., the difference in pain report to cued and uncued noxious stimuli) demonstrated increased levels of hippocampal activity when expecting pain and during the pain stimuli. These findings support the notion that the amygdala plays a role in the “fight or flight” response to dangerous stimuli, in this case pain. Findings also suggest that the hippocampus is involved in both the expectancy of pain and appraisal of stimuli value.
In another study, Wise et al. (2007) examined the influence of midazolam (i.e., a short-acting anxiety medication) on brain activity associated with pain expectancy and pain. They hypothesized that brain activity associated with the anticipation of pain would be reduced when participants were administered midazolam (compared to administration of saline) but that the brain activity associated with pain stimuli would not change. To examine this, they conditioned healthy volunteers to expect either a painfully hot stimulus or a nonpainful warm stimulus on the basis of two different visual cues. As predicted, results of this work found that midazolam administration did modulate the brain activity associated with anticipation of pain. Three specific brain regions – contralateral aINS, anterior cingulate, and ipsilateral SII – associated with pain anticipation showed decreases in activity when anticipating pain under midazolam application, but not under the control condition (i.e., saline administration). Midazolam application did not have a significant effect on pain-related brain activity. Participants receiving midazolam compared to saline did, however, rate the pain stimulus as significantly lower. This suggests that midazolam produced some analgesic effect evidenced by pain report, but that it did not alter brain activity related to the stimuli. This is a very important finding because it suggests that pharmacological agents can have differential neural effects on pain and on the anticipation of pain.
Hypnosis. Hypnosis for pain has been used in both acute and chronic pain settings to produce an analgesic effect (Barber 1996; Crawford et al. 1993). It has been shown to be beneficial for managing pain due to a number of medical conditions including sickle cell disease, advanced cancer pain, osteoarthritis pain, and disability-related pain (Dinges et al. 1997; Elkins et al. 2004; Gay et al. 2002; Jensen et al. 2005). Hypnosis for pain usually includes two phases: (1) patients are led through an induction phase which includes imaging a sequence of calming statements or images (e.g., “everything is just right,” or a peaceful green meadow) and (2) patients are given suggestions of experiencing less pain, less stress, more energy, and more control (Keefe et al. 2010).
In an interesting study design, Derbyshire et al. (2004) compared brain activity across three different conditions: (a) noxious stimuli application, (b) hypnotic suggestion of a painful state in the absence of noxious stimuli (i.e., hypnotically induced pain), and (c) imagined pain. Participants reported pain ratings for the noxious stimuli application and hypnotically induced pain in the absence of noxious stimuli using a verbal rating scale where 0 = no pain and 10 = maximal pain. Average pain rating following the noxious stimuli was 5.7 (range 3–10) and during the hypnotically induced pain was 2.8 (range 1–9). Brain activity in response to noxious stimulus application and hypnotically induced pain was similar – that is, the thalamus, ACC, midanterior insula, and parietal and prefrontal cortices showed similar activity in the two conditions. Imagined pain did not involve the same degree or pattern of activation as the other two conditions (i.e., noxious stimulus application, hypnotically induced pain). These results suggest that brain activation is similar in response to both actual noxious stimulation and suggestion of noxious stimuli. They are particularly important because they suggest that actual noxious stimulation is not necessary to activate pain-related brain regions.
Abrahamsen et al. (2010) explored whether hypnotically induced hypoalgesia or hyperalgesia would modulate the pain experience and associated brain responses in patients with a common chronic pain condition (i.e., temporomandibular disorder (TMD)). They investigated whether individual susceptibility to hypnosis measured by changes in perceived pain intensity and unpleasantness would correlate with brain activity during hypoalgesia and hyperalgesia. In this study, 19 patients were divided into three different experimental conditions: hypnotic hypoalgesia, hypnotic hyperalgesia, and a control condition (i.e., patients in normal alert state). In all three conditions, patients were subjected to repetitive pin-prick stimuli of identical intensity. The results of this study demonstrated that hypnotic modulation can increase or decrease patients’ perception of pain and unpleasantness of painful stimuli and that these changes are associated with distinctly different brain activation patterns. Specifically, when compared to the control condition, painful stimulation during hypnotic hyperalgesia was associated with increased activity in right posterior insula and BA6 and left BA40 and hypnotic hypoalgesia was only associated with activity in right posterior insula. These findings indicate that hypnotic hypoalgesia produces significant decreases in brain activity in response to pain stimuli in patients with a chronic pain condition. An interesting feature of this study is that it was conducted in a population of persons suffering from chronic pain. This contrasts with most imaging studies of hypnosis that have relied on samples of healthy volunteers. Understanding how hypnosis is related to pain-related brain activity in patients with chronic pain conditions is particularly important as hypnosis may be a promising treatment to decrease the negative influence of pain.
Effect of Emotions
There is growing evidence that negative emotions, particularly anxiety and depression, are related to increased pain and that increased pain can lead to increased emotional distress (Fishbain et al. 1997; Fishbain 2002; Mee et al. 2006). Fully understanding the relationship between pain and emotional distress is important so that efforts can be made to interrupt a negative cycle of increased pain and emotional distress. Several common brain regions have been implicated in both pain and emotion (e.g., ACC, insula). However, the interrelationship of brain activity between pain and emotions is just beginning to be explored and explained.
Ploghaus et al. (2001) conducted one of the earliest studies using imaging to investigate the effects of anxiety on pain. In this study, participants were presented with a visual signal, which was always followed by a mild thermal nociceptive stimulus, thus making this particular visual signal induce low anxiety. A second signal was presented, which was usually followed by the same mild thermal nociceptive stimulus, but occasionally was followed by a very intense thermal noxious stimulus, resulting in a cue producing high anxiety. A manipulation check to ensure that the conditions produced the expected anxiety levels was completed in a separate group of participants; anxiety was significantly higher in the condition expected to produce higher anxiety. The investigators cited the importance of obtaining anxiety ratings in a separate group as conscious self-assessment of both processes in the same subject might lead to hypothesis-driven correlations. Imaging results found that pain-related brain activity in the entorhinal cortex was increased by expectation-induced anticipatory anxiety of pain stimuli. Increased activation in the entorhinal cortex was related to activity in the cingulate cortex and the insula (i.e., other pain-related areas). The results of this study suggest that anxiety can modulate activity in pain-responsive areas of the brain. Patients receiving painful medical treatments (e.g., bone marrow transplants, chemotherapy) may experience increased brain activity in response to environmental cues (e.g., hospital parking lot, hallways), which signal pending treatment. Future studies should focus on understanding the neural correlates of interventions, which produce anxiety responses related to impending painful events.
In a recent study, Roy et al. (2009) combined fMRI of the brain with recording of a spinal nociceptive reflex to explore the effects of emotion on pain. Participants underwent trials where two noxious electrical stimuli were applied as they viewed pleasant, unpleasant, or neutral images. Standardized images from the International Affective Picture System were used to evoke emotion (Lang et al. 1998). Several interesting findings were noted. First, when participants were viewing unpleasant compared to pleasant images, their report of pain during the electrical stimulation was significantly greater. Second, emotions induced by either pleasant or unpleasant images modulated patients’ response to pain in the right insula, paracentral lobule, parahippocampal gyrus, thalamus, and amygdala (i.e., decreased activity in response to pleasant, increased activity in response to unpleasant). Third, when patients were viewing unpleasant compared to pleasant images, larger brain activations in the paracentral lobule, bilateral parahippocampal gyrus, and right ipsilateral insula were seen in response to the electrical stimulation. Finally, activity in the thalamus, amygdala, and several prefrontal areas was associated with the modulation of the spinal reflex responses. In sum, these results demonstrate that emotions modulate patients’ pain perception, brain activity in response to pain, and spinal nociceptive processes related to pain.
Giesecke et al. (2005) examined the relationship between depressive symptoms and experimentally induced pain in patients with fibromyalgia. These investigators found that depressive symptoms were positively related with experimentally induced pain activation of affective brain areas. Higher levels of depressive symptoms were associated with activation of the amygdala and contralateral aINS. Interestingly, patients’ rating of their fibromyalgia pain was associated with activation of the contralateral aINS, anterior cingulate cortex, and PFC. These findings provide evidence of a close relationship between pain and emotion at a cortical level.
The results of these studies are particularly important as strong emotions are likely to emerge in acute and chronic pain contexts. Future work should consider the relationships between pain-related brain activity and emotions in the context of acute pain (e.g., medical procedures) and chronic pain (e.g., diseases like arthritis and cancer). The findings from these imaging studies confer with clinical research suggesting that efforts to decrease negative emotions and increase positive emotions may have a positive impact on patients’ pain and overall well-being. A better understanding of how emotions impact patients (vs. healthy experimental subjects) facing acute and chronic pain can help direct the application of pain interventions.
Effect of Cognition
Over the past decade there has been growing interest in the effects of cognition on pain experience. One of the strongest cognitive predictors of pain is pain catastrophizing. When faced with pain, persons who catastrophize tend to focus on pain sensations, magnify how threatening pain is, and negatively evaluate their own ability to deal with pain.
To date, three imaging studies have examined how pain catastrophizing relates neural responses to pain stimulation. Gracely et al. (2004) conducted an imaging study of pain catastrophizing in fibromyalgia patients who were exposed to painful pressure stimulation. The fibromyalgia patients were divided into two categories (high vs. low) based on their level of catastrophizing. When compared to those in the low pain catastrophizing group, patients who scored high on pain catastrophizing showed increased activation in a number of brain regions related to pain: (1) areas related to sensory aspects of pain (SII), (2) areas involved in the anticipation of pain (cerebellum, medial frontal gyrus), (3) areas related to behavioral responses to pain (premotor cortex), and (4) brain regions related to attention and emotional aspects of pain (ACC). In a study conducted in healthy volunteers, Seminowicz and Davis (2006) examined how pain catastrophizing related to brain activation in response to pain. Brain imaging data were collected under two conditions: (1) during exposure to mild, electrical pain stimulation, and (2) during exposure to more intense, electrical pain stimulation. The level of pain catastrophizing was found to be correlated with brain activation patterns during both mild stimulation, with catastrophizers showing higher activity in brain regions related to vigilance and attention; and intense stimulation – where catastrophizers showed an impaired ability to activate areas of the brain responsible for descending modulation of pain (Fig. 27.2). The third and most recent study (Lu et al. 2010) used 3T-fMRI to examine the effects of pain catastrophizing on placebo analgesia in health volunteers undergoing lab-based esophageal pain stimulation. This study found no evidence of a relationship between pain catastrophizing and the activation of brain regions related to placebo effects. Taken together, the results of these studies provide some initial support for the notion that catastrophizing may be related to patterns of brain activation during pain stimulation.
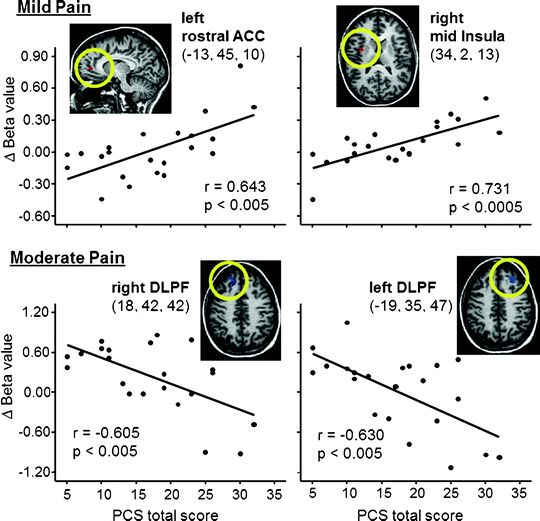
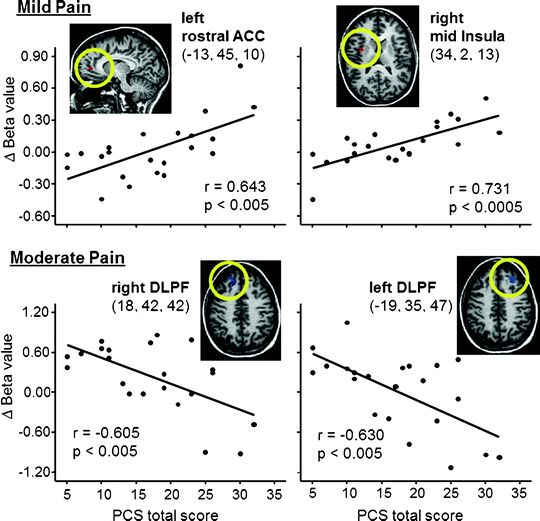
Fig. 27.2
Correlations between pain catastrophizing scale (PCS) scores and brain activity evoked by mild pain (top) and moderate pain (bottom). With mild pain, catastrophizing was associated with increased activity in rACC (a.k.a. pregenual ACC) and mid insula, areas considered to be involved in the affective component of pain, while with moderate activation catastrophizing was associated with a lack of activation of DLPF, which is thought to be involved in descending modulation of pain. ACC Anterior cingulate cortex; DLPF dorsolateral prefrontal cortex. Talairach coordinates shown (from Seminowicz and Davis (2006), used with permission)
Clinical observations suggest that a variety of cognitive behaviors can influence pain. For example, individuals having chronic pain often report that thinking about or imagining themselves engaged in a painful movement can increase their pain. Similarly, Gustin et al. (2008) found that persons with a complete spinal cord injury who were unable to physically move their ankle, reported increased pain when asked to imagine making movements of the ankle. More recently, these investigators (Gustin et al. 2010) conducted an fMRI study to examine the neural correlates of pain evoked by such movement imagery. A sample of 11 patients with complete thoracic level spinal cord injury and neuropathic pain experienced below the level of their injury and 19 controls were given 7 days of movement imagery training in which they were asked to imagine right ankle flexion and dorsiflexion movements 3 times daily for 8 min while listening to a recording of a car accelerator respectively accelerating and decelerating. All participants then underwent fMRI while imagining the ankle movements. Nine of the eleven patients reported increased pain with the imagined movements. During the movement imagery, both the patients and the controls showed increased activity in the supplementary motor area and cerebral cortex. However, the spinal cord injury patients showed significantly greater activity in perigenual anterior cingulate cortex and right dorsolateral frontal cortex. Interestingly, the level of activation in these areas was correlated with ratings of pain provided during the movement imagery. These findings are intriguing in that they provide evidence that a cognitive task can evoke pain and associated activation of areas of the pain neuromatrix.
Seeking social support from a loved one is one of the most common ways that people can cope with pain. Younger et al. (2010) recently used fMRI to examine the effects of viewing pictures of a new romantic partner on experimental pain. A sample of 15 undergraduates, all of whom were within the first 9 months of a romantic relationship, were exposed to moderate and high thermal pain stimulation under three different conditions: (1) while viewing pictures of their romantic partner, (2) while viewing pictures of an equally attractive friend, and (3) while engaged in a word-association task designed to distract them from pain. Results showed that viewing pictures of the romantic partner (but not the friend) decreased pain ratings. The word distraction task, which involved silently thinking of as many word associations that they could, also reduced pain ratings. Analysis of the fMRI data suggested that the mechanisms for pain relief differed for the viewing romantic partner vs. the distraction task. Specifically, viewing pictures of the romantic partner led to increased activation of areas of the brain involved in the reward system (i.e., head of the caudate, nucleus accumbens, lateral OFC, amygdala, and DLPFC) whereas the distraction task did not increase activity in these areas. These findings suggest that the pain relief that comes from viewing pictures of a new romantic partner can produce reductions in pain by activating reward systems. Future studies should examine whether thinking of a loved one or a romantic partner with whom one has been in a long-term relationship may foster pain relief through similar mechanisms, though as the authors point out more complex motivational, evaluative, emotional, and memory processes may be involved in such a case.
Pain Imaging in Patients
While pain neuroimaging over the last 2 decades has given us a general understanding of pain-related brain activity, one of the great challenges in the field is to understand how the central nervous system gets restructured as a result of suffering from chronic pain. Here, we focus on four areas of research which have addressed this issue.
Structural Analysis (T1, DTI)
Probably the most frequently reported neuroimaging studies comparing people with chronic pain and healthy controls is structural MRI, gray matter differences (VBM) or CTA. While the brain regions involved are somewhat variable, overwhelmingly, the results of these many studies indicate gray matter density loss in multiple brain areas is a common consequence of chronic pain (see reviews by May (2008) and Schweinhardt et al. (2008)); in particular, these studies implicate the cingulate and insular cortices, as well as prefrontal and parietal regions. Studies have also reported that decreased gray matter density or cortical thickness in patients is reversible with effective treatment (Gwilym et al. 2010; Rodriguez-Raecke et al. 2009; Seminowicz et al. 2010). A longitudinal study in a rat model of neuropathic pain reported prefrontal cortical volume decreases several months after the injury, and this morphometric change coincided with the onset of anxiety-like behaviors (Seminowicz et al. 2009). Thus, the evidence indicates that chronic pain causes brain atrophy, and that relief from chronic pain can restore normal brain anatomy. Furthermore, affective changes associated with chronic pain are likely partly responsible for these brain changes.
A few studies to date have examined the structural connectivity changes with DTI. Geha et al. (2008a) reported aberrant connectivity patterns from the anterior insular cortex in people with chronic regional pain syndrome compared to healthy controls, as mentioned in section “Diffusion Tensor Imaging.” Other groups have shown altered white matter tracts in migraine (Moulton et al. 2011), CPSP (Seghier et al. 2005), and fibromyalgia (Lutz et al. 2008; Sundgren et al. 2007). This technique seems particularly helpful when used in combination with fMRI or VBM, where one can show a relationship between abnormal structure or function, and connections to or from that region.
Resting State fMRI
In resting state fMRI, subjects are usually asked to lie awake and relaxed in the scanner, with eyes open or closed. This technique is useful for assessing functional networks in the resting brain, i.e., without any task imposed. Several resting state networks have been identified, including a sensorimotor network (Biswal et al. 1995), a default mode network (DMN; Greicius et al. 2003; Raichle et al. 2001), which is a set of regions normally deactivated during task execution (i.e., areas that are active at rest), and several others (De Luca et al. 2005).
Two anti-correlate networks were identified by Fox et al. (2005), and called the task-positive network, which resembles the set of regions normally activated by performance of a cognitively demanding task, and a task-negative network, which resembles the DMN. Seminowicz and Davis (2007) reported that this task-positive network could be enhanced in healthy individuals when pain was evoked with electrical nerve stimulation, suggesting that pain acts as an additional cognitive load (Fig. 27.3). Patients with chronic low back pain have reduced deactivations in task-negative areas (Baliki et al. 2008), suggesting that these areas might be less active at rest, perhaps because of hyperactivity of task-positive regions. Mantini et al. (2009) supported these findings, reporting that in healthy individuals pain evoked with electrical nerve stimulation led to reduced DMN activity. Thus, chronic and experimental pain can modulate brain activity at rest, and this effect might be explained by the effects of pain on cognitive networks.
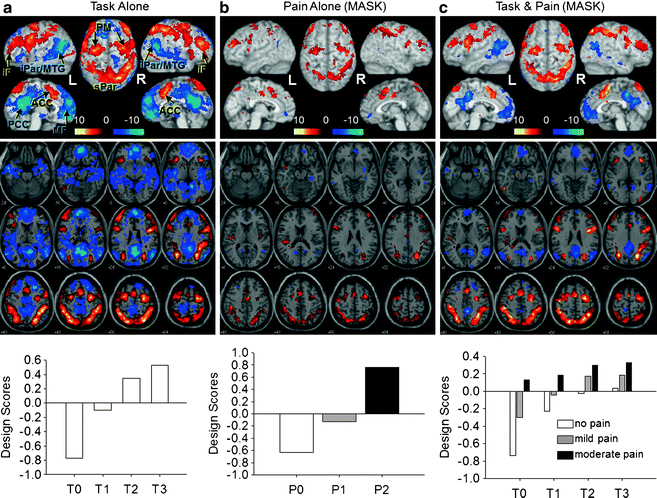
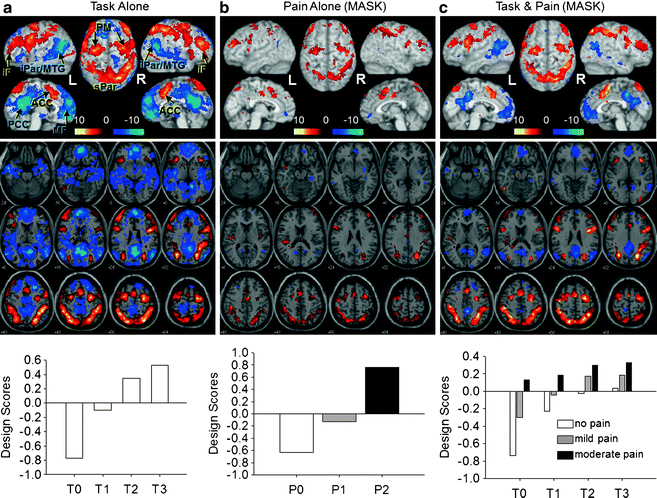
Fig. 27.3
Pain and cognitive load activate an intrinsic task-negative/task-positive network. An attention-specific network was identified in a partial least squares analysis with four levels of cognitive demand in a pain-free state and displayed as a covariance map. (a) Design scores (bottom) indicate how the covariance pattern was represented by each condition. For example, for T0 (minimal cognitive load), the design score value was negative, so blue areas covary positively with this task and red areas covary negatively with the task and with the blue areas; T3 (maximum cognitive load) covaried most strongly with the task-positive network (red) and negatively with the task-negative network (blue). From this spatial pattern of activity, a mask was created, including only those voxels that were significantly associated with the design. (b) Conditions with no, mild, and moderate pain during the control task (T0) were included in the analysis in which the mask was applied to limit the areas to the task-positive and -negative networks. The design scores (bottom) indicate that as pain increases, activity in the task-positive network also increases (with one difference: bilateral SII/posterior insula are included in the task-positive here, whereas those regions were part of the task-negative network identified using only task conditions). Thus pain alone activates the task-positive network. (c) All pain and task conditions. The results indicate that task difficulty is associated with increased activity of the task-positive network and reduced activity in the task-negative network, and pain further activates the task-positive network, and suppresses the task-negative network. iF Middle/inferior frontal; iPar/MTG inferior parietal/middle temporal gyrus; ACC anterior cingulate cortex; PM premotor cortex; MF medial frontal; PCC precuneus/posterior cingulate cortex; sPar superior parietal (from Seminowicz and Davis (2007) (Journal of Neurophysiology), Am. Physiol. Soc., used with permission)
Other studies have reported altered resting state activity in chronic pain conditions, including changes in spatial networks (Cauda et al. 2009, 2010; Napadow et al. 2010; Tagliazucchi et al. 2010), and in the temporal (frequency) aspects (Malinen et al. 2010). One of the great advantages of resting state fMRI is that many different networks – cognitive, salience (Seeley et al. 2007; Taylor et al. 2009), or sensorimotor – can be interrogated from a single dataset. Data sharing projects now let researchers access tens of thousands of resting state scans from different settings, and this will allow for comparisons of resting state networks in many different disorders.
Effect of Cognitive Behavioral Therapy
Although cognitive behavioral therapy (CBT) interventions have been used in the management of persistent pain for some time (see also Donovan et al. 2011), relatively little attention has been given to the mechanisms by which CBT achieves pain relief. To our knowledge, only one published study has examined the effects of a CBT protocol on patterns of brain activation during a painful inducing task (rectal balloon distension) (Lackner et al. 2006). In this study, the responses of six irritable bowel syndrome patients who had undergone CBT were compared to that of five healthy controls. All patients in the study underwent an imaging session before and after completing a 10-week CBT protocol which included training in several coping skills such as self-monitoring, cognitive reappraisal of pain, strategies for controlling worry, and problem solving. Data analyses showed that, after completing CBT, patients had reductions in neural activity in the parahippocampal gyrus and inferior portion of the right cingulate cortex when compared to both their pretreatment responses and the responses of healthy controls. Interestingly, the magnitude of change in these neural responses was found to be significantly correlated with reductions in a number of gastrointestinal symptoms including pain, as well as reductions in anxiety. The authors concluded that changes in the activation of brain structures, involved in attention and emotion regulation, may represent one mechanism by which CBT achieves its effects on pain and other symptoms of irritable bowel syndrome. Naylor et al. (manuscripts submitted for publication) studied the effects of CBT on brain function and structure in patients with chronic musculoskeletal pain. After 11 weeks of CBT patients showed increased activity in the insula, reduced activity in the amygdala and frontal areas associated with emotional processes of pain. This activity was correlated with subjective measures of pain and coping. In this study Naylor et al. also assessed the neuroplasticity of gray matter atrophy previously reported in patients with chronic musculoskeletal pain. CBT was associated with increased gray matter volume (GMV) in DLPFC and aACC. The post-CBT increase in gray matter volume was correlated with a decrease of pain catastrophizing (Naylor et al).
Given the interest in the neural correlates of CBT treatments for emotional disorders (e.g., panic disorder) (de Carvalho et al. 2010), the relative lack of studies of CBT for pain relief is surprising. This is clearly an important direction for future research.
Effects of Placebo
Interest in the neural correlates of placebo analgesia has been high (see also Pollo and Benedetti 2011). A number of studies support the notion that placebo analgesia is related to activation of the brain regions involved in endogenous opioid pain modulation (Bingel et al. 2006; Petrovic et al. 2010; Wager et al. 2004; Zubieta et al. 2005) – the same regions involved in opioid analgesia.
An interesting possibility is that placebo analgesia not only affects pain processing at the level of the brain but also at the level of the spinal cord. Eippert et al. (2009) used fMRI imaging of the cervical spinal cord to test this possibility. Administration of a placebo agent, which reduced ratings of thermal pain by 26%, what posterior parietal cortex, left DLPFC found to be associated with a significant reduction in BOLD responses at the ipsilateral dorsal horn of the spinal cord. These results are consistent with the notion that descending pain control systems responsible for placebo analgesia influence the gating of nociceptive input at the level of the spinal cord.
Recently, researchers have been interested in the effects of placebo conditioning trials on the effects of placebo analgesia. In placebo conditioning trials, patients are provided with repeated exposures to a placebo (e.g., a placebo cream applied to an area of pain stimulation) and this is matched with intentionally lowered level of pain stimulation to increase patients’ expectations of placebo analgesia. Watson et al. (2009) studied the effects of placebo conditioning on brain activity occurring in response to a laser pain stimulus. Participants, 11 healthy individuals, underwent brain imaging before, during, and after the placebo conditioning trials as well as administration of the placebo analgesia. Data were collected during both anticipation of pain stimulation and during pain stimulation. Anticipation of pain stimulation was associated with activation of the left DLPFC, medial frontal cortex, and anterior mid-cingulate cortex for both the placebo conditioning and placebo administration conditions. Delivery of pain stimulation was associated with activation of the anterior MCC and posterior cingulate. The authors suggest that their findings support the notion that placebo conditioning produces activations in prefrontal and mid-cingulate cortices which persist during placebo analgesia.
Investigators have examined the possibility that there may be differences in the mechanisms underpinning opioid analgesia and endogenous opioid analgesia. Petrovic et al. (2010) argued that during administration of opioids, patients’ expectations that they will obtain effective pain relief are met and are matched with corresponding changes in the processing of nociceptive input. This contrasts with a placebo condition during which patients’ expectations for effective pain relief are not necessarily matched by corresponding changes in the processing of nociceptive input. Given prior findings that prefrontal mechanisms are involved in expectation processes and the matching of expectations with sensory input, Petrovic et al. (2010) hypothesized that these regions might be more active in placebo analgesia vs. opioid analgesia. To test this possibility, they reanalyzed data from an fMRI study contrasting opioid analgesia and placebo analgesia. Their findings indicated that, when compared to opioid analgesia, placebo analgesia was associated with substantial increases in activity in prefrontal areas (specifically, the lateral OFC and ventrolateral PFC). A connectivity analysis showed that activation of these prefrontal regions covaried with activation of the right ACC. The authors concluded that the mechanism of placebo analgesia is distinct from opioid analgesia in that it involves greater activation in prefrontal brain areas related to the processing of how expectations fit with sensory input.
Future Directions
We demonstrated in this chapter that there is considerable overlap among brain regions participating in processing of pain, cognition and emotions, including ACC, insula, amygdala, thalamus and PFC. A challenge for future research will be to unravel the mechanisms through which complex mental activities, such as cognitive behaviors (expectations, attention, distraction, catastrophizing) and emotions are able to influence brain functions and structures. As placebo analgesia is embedded in pain treatment, studying placebo effects on pain intensity and brain function would bring better understanding of how placebo analgesia can be used in clinical treatments. There is a clear need for additional studies of neural correlates of CBT treatments for pain and recent studies of the effects of CBT for emotional disorders could have implications for this research. Furthermore, more studies are needed to understand not only cortical processes but also involvement of white matter tracts in development of chronic pain and its response to psychosocial interventions and medications. Finally, we need to address long-term efficacy of psychological interventions for pain and their neural correlates.
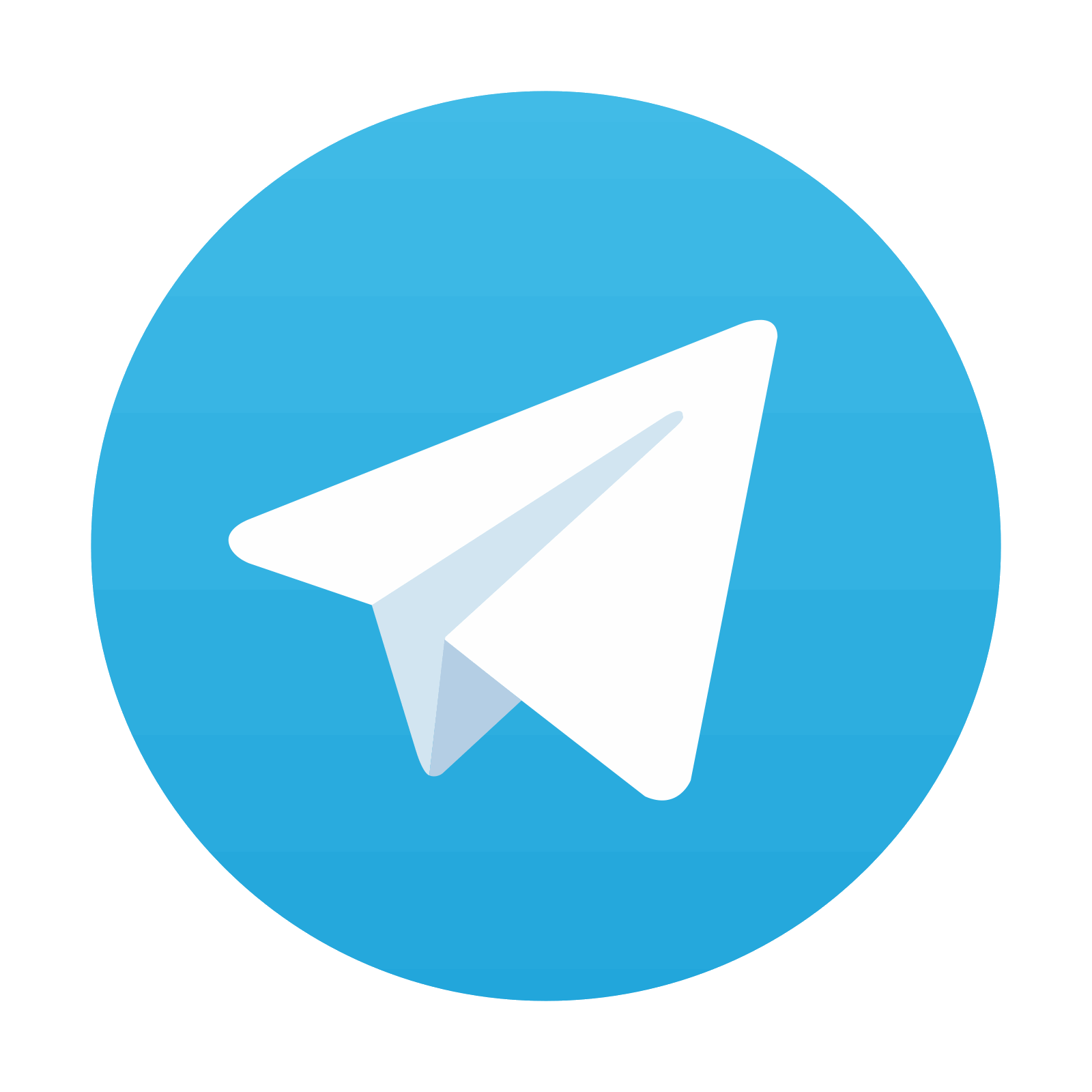
Stay updated, free articles. Join our Telegram channel
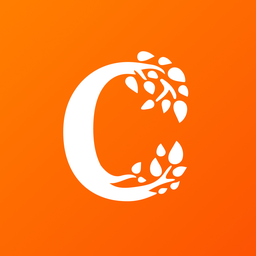
Full access? Get Clinical Tree
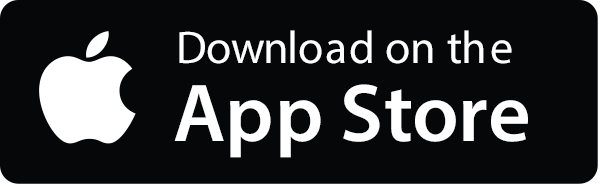
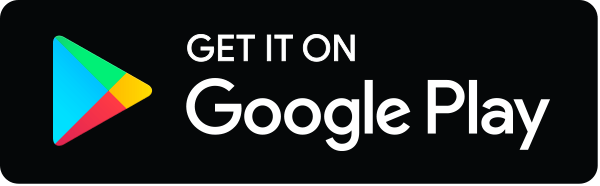