Change, alternatively referred to as reorganization or plasticity, is a fundamental property of the brain that enables it to adapt and thus enhance survival of the organism. It has been documented and studied across all the scales of the brain. The smallest unit of neural processing is the individual channel and its related receptor. Its efficacy can be modulated by various mechanisms, for example, changes in the availability or concentration of various chemicals within the intracellular or extracellular environment. Such a change can lead to an increase either in local resting membrane potential or, when it influences the excitability of the neuron, in an increased or decreased rate of firing. A change in either resting membrane potential or individual neuronal firing rate can in turn modulate individual synapse strength, which can be demonstrated experimentally as phenomena called long-term potentiation and long-term depression. Cumulative changes across these microscopic scales would in turn lead to reorganization of whole territories of the cortex, in which one functional specialization may be abandoned and replaced by another. Several review articles highlight the recent excitement in the topic across multiple brain functional systems, from the single synapse to large-scale reorganization. We now know that all of this happens in the human brain, perhaps more subtly in the adult than in the developing brain, and we also know that such events are fundamental for understanding the brain mechanisms of pain. We will revisit each of these mechanisms specifically in the context of pain perception.
Regarding pain, there has been a fundamental shift in our concept of the functional role of the brain. Until about 15 years ago, most basic research on pain involved charting the “telephone lines” and the “code” for these lines. Basically, most work in the field was an attempt to establish pain as a sensory modality with its unique pathways and presentation within the peripheral nervous system (PNS) and central nervous system (CNS). Chapter 8 , which discusses the anatomy of pain systems, nicely demonstrates this knowledge and shows the distinct PNS and CNS components that participate in coding pain. This work has been highly successful in showing that unique receptors in the skin are involved in responding to mechanical, thermal, and chemical noxious stimuli. This information is transmitted through specialized myelinated and unmyelinated fibers to the spinal cord, where the nociceptive input converges on specific populations of cells, which in turn transmit the signal cephalad through multiple ascending pathways to give rise to the perception of pain. Descending modulatory pathways converge back on nociceptive neurons in the spinal cord and control their level of excitability based on environmental conditions. This is the “telephone line” view of pain perception, also known as the specificity theory of pain, which some researchers criticized for years as being too limited and simplistic in scope. Its basic underlying assumption is a unidirectional flow of information from the environment to the cortex through specific pathways, disruption of any parts of which would break the telephone lines of communication and relieve the pain. Within this viewpoint there is little space to accommodate some of the most debilitating clinical pain conditions, namely, chronic pain, and in fact even the existence of these conditions was highly controversial for large portions of the past century. There remain hardcore scientists who still adhere to this notion ; however, the brunt of scientific evidence generated recently shows that the plasticity of the brain and peripheral nerves is a fundamental component of pain, especially for clinical pain in general and even more specifically for chronic pain. Recent studies have repeatedly uncovered the fact that disruption of any of the PNS or CNS components of the network underlying pain perception results, in most cases, in exacerbation of pain behavior rather than its diminution and that the specific site and extent of injury result in mimicking, at least in part, various clinical pain conditions. Plasticity of the PNS and CNS seems to be a universal consequence of persistent pain, the details of which depend on the type and extent of injury giving rise to the persistent pain. Accordingly, Woolf and Salter concluded, “All living organisms need to be able to react to noxious stimuli, and a major evolutionary drive for the development of a plastic nervous system might have been the acquisition of the capacity to detect and remember pain.” This suggests the possibility that the ability of the brain to change was driven by its need to adapt to coping with pain rather than the other way around.
Acute Nociception in Contrast to Persistent Pain
Activation of the nociceptive system in the absence of changes in the PNS or CNS is supposed to happen only when short-lasting stimuli (on the scale of a few seconds and not persisting for more than minutes) are applied that can evoke pain but do not cause inflammation or injury. Under such restricted conditions, one expects to observe a primarily neuroelectric response in which, depending on the stimulus, nerve endings of unmyelinated and small myelinated nociceptors are excited and, in turn, activate second-order spinal cord cells that project to multiple supraspinal sites. The best known and best characterized of these is the spinothalamic pathway, which has all the characteristics necessary for coding stimulus properties such as its location, intensity, and modality. The spinothalamic pathway has also been classically attributed with conveying the emotional properties of noxious stimuli, although recent brain-imaging studies are beginning to question this notion, at least in humans. As soon as noxious stimuli increase in duration or intensity, they are accompanied by inflammation or injury, which in turn results in a long list of PNS and CNS changes.
Definitions of General Pain Types
- •
Nociceptive pain refers to normal, acute pain perception evoked by short-lasting noxious stimuli in intact tissue in the absence of peripheral or central sensitization.
- •
Inflammatory pain refers to pain following tissue injury but with no neural injury. Some group this under nociceptive, others under pathophysiologic pain. There is ample evidence of peripheral and central reorganization in such conditions; thus, it cannot be regarded as normal pain.
- •
Neuropathic pain refers to pain after neural injury. It is generally accepted as a pathophysiologic state accompanied by peripheral and central reorganization.
Definitions of Abnormal Pain
- •
Allodynia is the perception of pain from a stimulus that in a healthy organism is not painful.
- •
Hyperalgesia is an enhanced perception of pain from a stimulus that does evoke pain in a healthy organism but to a lesser extent.
- •
Primary sensitization is an enhanced sensation and related enhanced neuronal transmission within and around the site of injury, which suggests that the effect is a consequence of reorganization of neuronal signaling directly from the injury.
- •
Secondary sensitization is an enhanced sensation and neural transmission at body sites removed from the site of injury. It is usually attributed to changes in neuronal properties in the spinal cord where the end product of various changes leads to increased excitability of neurons involved in transmitting nociceptive input cephalad. The process is assumed to be a result of reorganization of the spinal cord nociceptive circuitry.
- •
Supraspinal sensitization is the term used to distinguish between spinal and supraspinal changes in nociceptive coding. Such processes are assumed to change the cortical elements involved in pain perception. These in turn would modulate the spinal cord processing of afferent nociceptive input through descending modulatory pathways.
Inflammation and Changes in Response in Free Nerve Endings
Free nerve endings terminating in the skin and viscera are the machinery for signaling local mechanical, thermal, and chemical changes, as well as for modulating this local environment ( Fig. 10.1 ). Thus, even at the level of the afferent receptor, nociceptive afferents are involved in two-way communication. Moreover, these receptors undergo changes in their response properties that affect the trafficking of chemicals, as well as the sensitivity to local events. Free nerve endings possess a long list of receptors (proteins that span the lipid bilayer membrane that separates the extracellular and intracellular spaces) specialized for detecting the presence of specific chemicals or stimuli ( Fig. 10.2 ) that act as agonists and, by binding to the receptor, either activate intracellular second-messenger events or directly open the channel. To provide inflow of Na + ions, for example, the VR1 cation channel is activated at around 43° C and is also sensitive to capsaicin and acidity. This process initiates a current, which then generates an action potential that by propagating centrally into the spinal cord may in turn activate second-order neurons that transmit this information farther centrally. Injury or inflammation results in local release of the “inflammatory soup,” which includes peptides (bradykinin), neurotransmitters (serotonin), and neurotrophins (nerve growth factor). These factors interact with cell surface receptors (see Fig. 10.2 ) to activate the nociceptor. Besides transmitting the information centrally, this process also initiates neurogenic inflammation by locally releasing various neurotransmitters such as substance P and calcitonin gene–related peptide (CGRP). Release of these substances by terminals on the free endings of nociceptors induces local vasodilation and plasma extravasation, as well as activation of non-neuronal cells such as mast cells and neutrophils, all of which contribute to the inflammatory soup ( Fig. 10.3 ) and further sensitize these peripheral nociceptors by binding to their respective receptors, thus enhancing intracellular processes.



Inflammation and Changes in Response in Free Nerve Endings
Free nerve endings terminating in the skin and viscera are the machinery for signaling local mechanical, thermal, and chemical changes, as well as for modulating this local environment ( Fig. 10.1 ). Thus, even at the level of the afferent receptor, nociceptive afferents are involved in two-way communication. Moreover, these receptors undergo changes in their response properties that affect the trafficking of chemicals, as well as the sensitivity to local events. Free nerve endings possess a long list of receptors (proteins that span the lipid bilayer membrane that separates the extracellular and intracellular spaces) specialized for detecting the presence of specific chemicals or stimuli ( Fig. 10.2 ) that act as agonists and, by binding to the receptor, either activate intracellular second-messenger events or directly open the channel. To provide inflow of Na + ions, for example, the VR1 cation channel is activated at around 43° C and is also sensitive to capsaicin and acidity. This process initiates a current, which then generates an action potential that by propagating centrally into the spinal cord may in turn activate second-order neurons that transmit this information farther centrally. Injury or inflammation results in local release of the “inflammatory soup,” which includes peptides (bradykinin), neurotransmitters (serotonin), and neurotrophins (nerve growth factor). These factors interact with cell surface receptors (see Fig. 10.2 ) to activate the nociceptor. Besides transmitting the information centrally, this process also initiates neurogenic inflammation by locally releasing various neurotransmitters such as substance P and calcitonin gene–related peptide (CGRP). Release of these substances by terminals on the free endings of nociceptors induces local vasodilation and plasma extravasation, as well as activation of non-neuronal cells such as mast cells and neutrophils, all of which contribute to the inflammatory soup ( Fig. 10.3 ) and further sensitize these peripheral nociceptors by binding to their respective receptors, thus enhancing intracellular processes.
Neuropathic Pain and Peripheral Changes
Neuropathic pain is distinct from nociceptive and inflammatory pain conditions in that the injury is itself neuronal whereas inflammatory injuries are caused by disease of non-neural tissue. Osteoarthritis is the prime example of a persistent (or chronic) non-neuropathic condition, whereas clinical conditions such as post-herpetic neuralgia (PHN) and diabetic neuropathy are classic examples of neuropathic pain following metabolic disease or infection. When the neuropathic pain is of central origin (e.g., after autoimmune disease, stroke, trauma, or cancer), it has been proposed that it occurs only if the central insult directly involves the nociceptive pathways. The opposite does not seem to be true. That is, an injury to the nociceptive system does not imply the necessity of having pain. A partial lesion of a peripheral nerve commonly induces neuropathic pain. However, severing of dorsal roots seems to have little chance of creating lasting pain. Generally, neuropathic pain conditions are associated with abnormal neuronal activity at the site of the injury, as well as with central sensitization. Thus, neuropathic pain is generally viewed as paradoxical because it involves severing portions of the nociceptive system and thereby usually causing various types of sensory deficits, which rather than decreasing pain transmission results in long sustained increased pain because of reorganization of the peripheral and central neural elements participating in pain perception.
When neuropathic pain is a consequence of injury to the PNS, the extent to which it depends on peripheral as opposed to central sensitization continues to be debated, with strong evidence provided for both views. The mechanisms underlying such conditions have been studied extensively, and peripheral and central changes have been well characterized, primarily because of a number of animal models that have been advanced over the past 15 years. In contrast, pain induced by central neural injury remains less well characterized and is best studied following spinal cord injury (SCI). Neuropathic as well as inflammatory pain conditions are commonly accompanied by tactile allodynia, heat or cold hyperalgesia, and spontaneous pain. The extent of the contribution of each of these abnormalities to specific clinical conditions varies with the type of clinical condition; for example, PHN is associated with a very high incidence of tactile allodynia, whereas chronic back pain (CBP) is dominated by spontaneous pain. Consistent with this clinical picture, animal models of inflammatory pain that persists for different durations show distinct peripheral and central reorganization. Similarly, different animal models of neuropathic pain, in which the differences sometimes seem trivial (for example, different approaches used to induce partial sciatic nerve injury), also show various differences in reorganization.
In intact healthy organisms, nociceptive input is transmitted through Aδ and C nociceptors. However, there is now very good evidence that following central sensitization caused by inflammation or neuropathic injury, peripheral input to the CNS through non-nociceptive, thickly myelinated Aβ touch afferents may evoke pain. The latter is clear evidence that the functional roles of afferent fibers can be disturbed, again providing evidence that notions regarding specificity of the pain pathway are tenuous.
Nerve injury provides a new source of afferent activity as a result of ectopic discharges from the injured axons, in contrast to normal tissue, in which electrogenesis is limited to excitation of free nerve endings through transduction of noxious stimuli. When an axon is severed, its proximal stump seals off and forms a terminal swelling, or end-bulb. In adults, axotomized cells survive and begin to regenerate from the proximal cut end. Many of these cells grow back and reinnervate peripheral target tissue. When growth is blocked, as commonly occurs with most injury conditions, the terminal end-bulbs persist, turn back on themselves, and form a tangled mass termed a neuroma. There is good evidence that neuromas generate spontaneous ectopic activity that directly contributes to the perception of spontaneous pain. Neuromas are also sensitive to mechanical and chemical stimuli; their activity is exacerbated by temperature. Both A fibers and C fibers show ectopic activity, although the latter persist longer after injury, and sympathetic-sensory coupling may occur at the neuroma through noradrenergic sensitization. Overall, these changes, which are a consequence of damage to peripheral nerves, are triggered by metabolic and functional responses of the sensory cell that render it hyperexcitable, thereby contributing to the positive sensory symptoms of neuropathic pain, as well as triggering and maintaining central sensitization. Nerve injury induces alterations at multiple sites along the neural axis. Abnormalities occur in the injured and uninjured afferents, accompanied by central sensitization at the spinal cord level coupled with cell death. Additionally, there are changes in the descending control system, and immune responses in the periphery and the spinal cord are observed. As described later, such changes are also accompanied by reorganization of the cortical-subcortical circuitry involved in pain perception, in which immune responses as well as signs of cell death have also been observed. Generally, the literature on central sensitization simply refers to the fact that spinal cord transmission of nociceptive information is enhanced. Here, I argue that there is a specific cortical-subcortical reorganization that accompanies neuropathic pain and perhaps persistent inflammatory pain states as well. I label the latter “supraspinal sensitization.”
The following is a summary of the evidence regarding the roles of various sources of afferent input in neuropathic pain. (1) Systemic administration of a selective CB2 cannabinoid receptor agonist reverses the mechanical and thermal hyperalgesia–related behavior in an animal model of peripheral neuropathy. Because CB2 is expressed only in the periphery, the result is strong evidence of the role of peripheral afferents. (2) Similarly, reducing the efficacy of specific sodium channel subtypes in the periphery can decrease signs of mechanical hyperalgesia. (3) In addition to the hyperexcitable injured afferents, there is also good evidence that neuropathic pain can develop in the absence of activity from the injured nerve. (4) Spontaneous activity develops in uninjured, unmyelinated nociceptive afferents that innervate the same territory as the injured afferents, similar spontaneous activity develops following inflammatory injury, and in both the rate of this activity relates to spontaneous pain as assessed by the frequency of lifting the injured limb. (5) There is also evidence for sensitization of intact afferents that develop adrenergic sensitivity and increased responsiveness to thermal and mechanical stimuli. (6) Messenger RNA for a variety of receptors is upregulated in the cell bodies of dorsal root ganglion (DRG) neurons of both injured and uninjured afferents, which are regulated by trophic factors transported from the site of injury to these cell bodies. These processes contribute to changes in sensitivity to heat, cold, and mechanical stimuli in intact and injured afferents, as well as their responses to various modulatory input.
Central Sensitization Following Inflammatory, Neuropathic, or Cancer Pain
Glutamate is the dominant excitatory neurotransmitter in all nociceptors, and all primary nociceptors terminate and make synaptic contact with second-order neurons in the dorsal horn gray matter of the spinal cord. Unlike the rest of the CNS, nociceptive afferents also contain a long list of neuropeptides that are co-released from afferents and can modulate neurotransmission at longer distances than just at the synapse. Substance P is a peptide neurotransmitter that binds preferentially to the neurokinin-1 (NK1) receptor in the spinal cord. Changes in NK1 receptor expression and internalization can be used to identify the spatial and temporal effects of peripheral nociceptive input on individual spinal cord neurons ( Fig. 10.4 ). In animal models of both inflammatory and neuropathic injury there is ample evidence that second-order synaptic transmission becomes sensitized, though with distinct properties for each general type of injury. Moreover, newer evidence shows that in animal models of cancer pain there is yet another set of distinct cellular and molecular signs of reorganization regarding the state of second-order neurons within the spinal cord.
Inflammatory pain involves the sensitization of both primary afferent and spinal cord neurons. The neurochemical changes that contribute to inflammatory pain have been examined via expression and internalization of the NK1 receptor in the spinal cord (see Fig. 10.4 ) in acute, short-term, and long-term inflammatory pain states. With acute inflammatory pain there is ongoing release of substance P as measured by NK1 internalization in layer 1 neurons (neurons located at the marginal zone; these neurons send supraspinal projections to a variety of targets). Although there is no tonic release of substance P with short-term inflammatory pain, 3 hours after injury, both noxious and non-noxious somatosensory stimulation induces NK1 internalization in neurons located in layers 1, 3, and 4. This is significant because neurons in layers 3 and 4 are classically responsive only to innocuous stimuli and in intact, healthy organisms do not show NK1 internalization. In longer-term inflammatory pain models (3 weeks after the initial injury), the same pattern of substance P release and NK1 activation occurs as in short-term inflammation, with the addition of significant upregulation of NK1 in layer 1 neurons. These changes suggest that there are unique neurochemical signatures for acute, short-term, and long-term inflammatory pain, and given that NK1 internalization is associated with potentiation of glutamatergic neurotransmission, it is clear that the longer that inflammatory conditions persist, the more spinal cord circuitry is recruited and associated with the behavior.
With inflammation there is significant upregulation of substance P and other neuropeptides, such as CGRP, in the dorsal horn, whereas these same primary afferent neurotransmitters are downregulated with neuropathic pain. In contrast, in a bone cancer pain model, no change in these peptidergic neurotransmitters takes place. Likewise, galanin and neuropeptide Y are markedly upregulated in sensory neurons with neuropathic pain, but no change is observed in these neurotransmitters with cancer pain. Even more marked are the different neurochemical changes induced by each pain state in the spinal cord. Whereas inflammation induces an increase in substance P and CGRP in layers 1 and 2 of the spinal cord, nerve injury induces downregulation of these same markers in this area of the spinal cord with an additional upregulation of galanin and neuropeptide Y. In contrast, with cancer pain, the concentrations of these molecules or markers are not significantly changed. However, the greatest change observed in the spinal cord in response to metastatic bone cancer pain is the activation of astrocytes. These results imply that with inflammatory, neuropathic, or cancer pain, a unique and highly distinct set of neurochemical changes occur in the spinal cord and DRG.
Central sensitization occurs following inflammation or neuropathic injury and involves homosynaptic and heterosynaptic mechanisms. Homosynaptic mechanisms involve changes in the response properties of second-order neurons with direct afferent input from the site of injury, whereas heterosynaptic mechanisms underlie the spread of hyperexcitability to neurons with input from intact afferents. The latter accounts for the mechanisms of allodynia. In this case, nociceptive input alters synaptic efficacy such that Aβ mechanoreceptors acquire the capacity to activate second-order nociceptors. Additionally, uninjured nociceptors can acquire spontaneous activity after neuropathic injury, and this leads to enhanced excitability of the spinal cord nociceptors supplied by this input. Sensitization, both homosynaptic and heterosynaptic, involves the increased release of excitatory neurotransmitters, such as glutamate and substance P, or enhanced synaptic efficacy, all of which result in a reduction in the threshold for activation, increased responsiveness as measured by the number of action potentials generated for a given stimulus, and expansion of the receptive fields of dorsal horn neurons. There is a long list of mechanisms that can contribute to different extents to this outcome, including (1) presynaptic changes, (2) postsynaptic changes, (3) interneuron changes, (4) changes in descending modulation, (5) immune and microglial mechanisms, and (6) cell death. Convincing evidence along all these lines is outlined only briefly here.
- 1.
Regarding presynaptic effects, the best evidence is for opioid receptors, which are downregulated with neuropathic pain and upregulated with inflammatory pain. Because opioid receptors control presynaptic and postsynaptic neural transmission from nociceptive afferents, these changes in expression result in modulation of central nociceptive transmission by both mechanisms.
- 2.
There is ample evidence for involvement of postsynaptic mechanisms in central sensitization. Primarily, release of substance P and other peptides opens the N -methyl- d -aspartate (NMDA) glutamate-gated channel, thereby leading to increased calcium entry, which in turn may also potentiate glutamate transmission through α-amino-3-hydroxy-5-methyl-4-isoxazolepropionate (AMPA) receptors.
- 3.
Inhibitory γ-aminobutyric acid (GABAergic) interneurons control the overall excitability of neuronal transmission throughout the CNS and play an important role in governing the sensitivity of dorsal horn–projecting neurons. The reduced expression of inhibitory receptors observed following nerve injury decreases the inhibitory control on afferent input. Also, nerve injury is accompanied by downregulation of the potassium chloride (KCl) transporter, which reverses the effects of GABA release in that opening of the Cl channel now leads to excitation rather than inhibition.
- 4.
Descending modulatory pathways seem to reorganize with neuropathic pain and play a critical role in associated behavior. The rostral ventromedial (RVM) medulla receives input from the periaqueductal gray (PAG), which in turn receives convergent input from cortical, basal ganglia, and amygdala input. The RVM medulla projects to the spinal cord and has excitatory and inhibitory innervations within the dorsal horn. Many of the RVM cells express opioid receptors, ablation of which either before or after induction of neuropathic behavior eliminates hyperalgesic behavior. This evidence is important from two viewpoints: first, the descending modulation must be substantially reorganized with neuropathic pain, and second, the supraspinal, including cortical, input interacts with the spinal cord processing of nociceptive afferent input and plays an important role in hyperalgesic behavior. Again, this provides the view that pain behavior is the integrated output of ascending and descending signals throughout the CNS and casts grave doubt on the unidirectional, dedicated telephone line model of pain perception.
- 5.
Involvement of the immune system in peripheral inflammation is well established (see Fig. 10.3 ). There is now also good evidence for its role in neuropathic conditions. Peripheral nerve damage evokes a cascade of peripheral immune responses that lead to macrophage infiltration and increased expression of proinflammatory cytokines. Knockout of the proinflammatory interleukin-1 (IL-1) receptor along with overexpression of the IL-1 receptor antagonist decreases hyperalgesia and results in reduced spontaneous activity as recorded from dorsal root fibers. Thus, peripheral IL-1 clearly participates in neuropathic pain. There is recent evidence that central immune mechanisms, mediated through activation of microglia, are also essential in neuropathic pain ( Fig. 10.5 ).
Figure 10.5
Numerous changes in spinal microglia take place after peripheral nerve injury and result in the phenomenon of activation, which leads to enhancement of central sensitization and mechanical allodynia. A critical factor in relation to neuropathic pain is upregulation of expression of the P2X4 receptor. Influx of calcium into microglia through P2X4 receptors results in an intracellular cascade involving phosphorylation (P), the result of which is the release of brain-derived neurotrophic factor (BDNF). BDNF then acts on neurons in the dorsal horn to drive the spinal hyperexcitability underlying neuropathic pain.
(From Trang T, Beggs S, Salter MW. Purinoceptors in microglia and neuropathic pain. Pflugers Arch . 2006;452:645-652, with permission.)
- 6.
Cell death has now been repeatedly documented to occur in the spinal cord following peripheral nerve injury. It seems to have a limited time course, underlies apoptosis, and affects primarily GABAergic inhibitory interneurons ( Fig. 10.6 ).
Figure 10.6
Mechanisms of neurodegeneration in the spinal cord following peripheral nerve injury. (1) Abnormal ectopic activity from injured and neighboring uninjured primary afferents generates increased extracellular glutamate levels in the dorsal horn. (2) Excess activation of N -methyl- d -aspartate (NMDA), glutamate, and α-amino-3-hydroxy-5-methyl-4-isoxazolepropionic acid (AMPA) receptors leads to a rising influx of Ca 2+ into dorsal horn neurons, which over time exhausts the buffering capacity of mitochondria. (3) Apoptosis is induced in γ-aminobutyric acid (GABAergic) interneurons. (4) As a result, the GABA-mediated presynaptic and postsynaptic inhibition of sensory transmission is diminished, and input from nociceptive and non-nociceptive afferents is conveyed without adequate control. The response to noxious stimulation becomes exaggerated (producing hyperalgesia), and normally innocuous stimuli begin to produce pain (allodynia).
(From Apkarian AV, Scholz J. Shared mechanisms between chronic pain and neurodegenerative disease. Drug Disc Today Dis Mech . 2006;3:319-326, with permission.)
This summary of peripheral and spinal cord changes with persistent pain of various types highlights the main components involved. It also ignores or skims over much of the detail. In fact, the literature on the subject is vast and growing rapidly. Here I have attempted to identify cellular, molecular, and structural changes that accompany persistent or chronic pain. This overview also sets the stage so that one can examine the literature regarding human brain activity with respect to pain and its relationship to the expected equivalences between supraspinal sensitization and peripheral and central sensitization.
Nociceptive Supraspinal Projections and Cortical-Subcortical Pain Circuitry
There is a long list of pathways that convey nociceptive information from the spinal cord to higher brain centers (see Chapter 8 ). These pathways can generally be subdivided into two groupings: pathways accessing the cortex through the thalamus, which is the spinothalamic pathway, and pathways that access the cortex more indirectly through synapses at the brainstem, amygdala, and basal ganglia, as well as direct projections from the spinal cord to the prefrontal cortex. The relative size of these projections and the response properties of spinal cord neurons that participate in the different pathways have been studied relatively well in mammals, but such information is mostly lacking in humans. It is quite possible that some of these pathways may be preferentially enhanced in humans as compared with other mammals. The spinothalamic pathway has been studied most extensively, and the supraspinal circuitry regarding pain is usually cast within the anatomic framework of this pathway while ignoring the contribution of the others.
Conscious perception of external stimuli requires encoding by sensory organs, processing within the respective sensory system, and activation of the appropriate sensory cortical areas. Based on a small case series of infratentorial and supratentorial brain lesions in 1911, Head and Holmes postulated that the sensation of pain is an exception to this rule and that its conscious perception occurs in the “essential organ of the thalamus.” Despite evidence to the contrary from clinical reports, single-unit recordings in animals, and neuroanatomic tracings, it was maintained for a long time that the cortical representation of pain was a negligible one.
This situation changed when modern neuroimaging techniques (positron emission tomography [PET] and functional magnetic resonance imaging [fMRI]) demonstrated systematic changes in metabolism and perfusion in a large number of cortical areas following the application of painful stimuli. These findings were supported by invasive and noninvasive electrophysiologic studies in humans using magnetoencephalography (MEG), electroencephalography (EEG), and subdural and depth recordings. Meanwhile, it has been recognized that painful stimuli activate a vast network of cortical (and subcortical) areas, including the primary and secondary somatosensory (SI, SII) cortices, insula, posterior parietal cortex, anterior and midcingulate cortex, and parts of the prefrontal cortex ( Fig. 10.7 ). These areas are now presumed to be involved in the generation of pain perception, as well as in the descending control of pain. A recent meta-analysis examined the incidence of statistically significant activity across all brain-imaging studies of pain in healthy subjects over the past 15 years and indicated that in 68 studies the incidence of activity across these regions was 55% to 94%, with the lowest incidence seen for the prefrontal cortex (55%) and the highest for the insula (94%). Hence the nociceptive system converges with other systems for generation of the conscious percept of pain. In this sense the nociceptive system is not different from, for example, the visual system. However, to what extent any cortical regions can be considered nociceptive specific is still an open question.
Over the past 15 years or so, activation of the cortical and subcortical areas (including the thalamus, basal ganglia, amygdala, and brainstem) by pain in healthy subjects has been examined in terms of the specific dimensions of pain that can be mapped within this network, as well as its modifications with various cognitive and pharmacologic manipulations. Traditionally, pain perception has been conceived to consist of sensory-discriminative, affective-motivational, and cognitive-evaluative dimensions. The sensory-discriminative dimension includes intensity discrimination, pain quality, stimulus localization, and timing discrimination; this dimension is traditionally thought to involve the lateral thalamic nuclei and the SI and SII cortices. The affective-motivational dimension includes perception of the negative hedonic quality of pain, autonomic nervous system manifestations of emotions, and motivated behavioral responses; this dimension is traditionally thought to involve the medial thalamic nuclei and the limbic anterior and medial cingulate cortices (ACC and MCC, respectively). The insula has an intermediate position in this concept and receives input from the lateral thalamus but projects into the limbic system. The cognitive-evaluative dimension includes interaction with previous experience, cognitive influence on perceived pain intensity, and an overall evaluation of its salience; this dimension is traditionally thought to involve the prefrontal cortex. However, numerous neuroimaging studies have assessed various experimental paradigms derived from several psychological concepts that do not easily fit into the traditional three dimensions of pain. Therefore, I discuss the evidence for involvement of cortical areas in specific functions instead of the dimensions of pain.
Location and Quality of Phasic Pain
Neuroimaging studies have examined brain regions activated by many types of painful stimulation, including noxious heat and cold, muscle stimulation, topical and intradermal capsaicin, colonic distention, rectal distention, gastric distention, esophageal distention, ischemia, cutaneous electric shock, ascorbic acid, laser heat, and the illusion of pain evoked by combinations of innocuous temperatures. Despite the differences in sensation, emotion, and behavioral responses provoked by these different types of pain, individuals can easily identify each as being painful. Thus, there appears to be a common construct of “pain” with an underlying network of brain activity in the areas described. Nevertheless, despite the similarities in pain experiences and similarities in neural activation patterns, each pain experience is unique. Subjects can usually differentiate noxious heat from noxious cold and from noxious pressure. Given that there is ubiquitous convergence of information from cutaneous, visceral, and muscle tissue throughout the afferent nociceptive system, differentiation of types of pain must be achieved by feature extraction via interactions between neurons in the cortical-subcortical circuitry ( Fig. 10.8 ). Although this has not yet been demonstrated, somatotopic organization for noxious stimuli has been shown in human brain imaging, at least for the SI and SII cortices and the basal ganglia, and electrophysiologically in nonhuman primates in the thalamus. Thus these regions can all play a part in localization of pain. Strigo and colleagues directly compared the brain activation produced by esophageal distention and by cutaneous heat on the chest that were matched for pain intensity. They found that the two qualitatively different pain types produced different primary loci of activation within the insula, SI cortex, and motor and prefrontal cortices. Such local differences in response within the “nociceptive network” might subserve our ability to distinguish visceral and cutaneous pain, as well as the differential emotional, autonomic, and motor responses associated with these different sensations.
Representation in the Time Domain
The dual pain sensation elicited by a single brief painful stimulus is caused by the different conduction times in nociceptive A and C fibers (about 1-sec difference) and is reflected in two sequential brain activations on EEG and MEG recordings from the SI and SII cortices and the MCC. Intracranial recordings show that the earliest pain-induced brain activity originates in the vicinity of the SII cortex. These observations support the suggestion derived from anatomic studies that the SII region and adjacent insula are primary receiving areas for nociceptive input to the brain.
Attention and Distraction Effects
Early human brain-imaging studies examining the effects of attention and distraction showed modulation of pain-evoked activity in a number of cortical regions, including the sensory and limbic structures, as well as the prefrontal areas. Other studies extended these notions by showing that during distraction there is a functional interaction between the pregenual ACC and the frontal cortex that exerts a top-down modulation on the PAG and thalamus to reduce activity in cortical sensory regions and correspondingly decrease the perception of pain.
Anticipation and Expectation
Anticipation or expectation of pain can activate many of the cortical areas related to perception of pain in the absence of a physical pain stimulus. Two studies have attempted to identify the circuitry for modulation of pain expectation. The results indicated that the expectation of pain intensity is necessary for maximal activation of the afferent pain circuitry and maximal perceived pain intensity, thus suggesting that cortical modulation by expectancy changes the gain for afferent input to the cortex. Generally, there remains a strong need for systematic studies to identify brain elements that modulate pain responses as a result of expectation.
Empathy
A provocative study in which the authors defined empathy as the ability to have an experience of another’s pain opened the field regarding the interaction between pain and empathy. Using this definition and comparing brain activity when experiencing pain or knowing that a loved one, present in the same room, was experiencing the same pain, the authors showed that many cortical regions were similarly activated for both conditions. These results were interpreted as evidence that the affective component of pain is active in both empathy and pain, and they thus concluded that empathy for pain involves the affective component but not the sensory component of pain. The study induced a flurry of activity in attempting to understand the relationship between empathy and pain. Multiple groups have replicated the main finding and proposed different underlying mechanisms. Even though these results are internally consistent, their interpretation remains problematic. Simple introspection casts doubt on the notion that empathy means actually experiencing another person’s pain. Instead, what is called empathy may be assessment of the magnitude of negative emotion that the other person may be experiencing, that is, a cognitive function of interpersonal communication. According to this concept, empathy may be conceived of as a psychological process involving cognitive-evaluative and affective mechanisms that allows us to understand the personal experience of another person. A study in patients with congenital insensitivity to pain reported a deficit in rating pain-inducing events, but normal inference of pain from facial expressions (“empathy”), thus indicating that empathy for pain does not require an intact pain percept.
Mood and Emotional States
Studies show that experimental procedures that improve mood generally reduce pain whereas those that have a negative effect on mood increase pain. One study showed that looking at fearful faces increases the level of anxiety and discomfort, which also resulted in enhanced esophageal stimulation and evoked activity in limbic regions such as the ACC and insula.
Placebo
Placebo is a potent modulator of pain; it affects all clinical studies of pain pharmacology. Placebo effects have also been seen in depression and Parkinson’s disease, and recent brain-imaging studies have shown robust involvement of the brain and subcortical reward circuitry in these conditions. The first neurochemical evidence for opioid involvement in placebo was demonstrated about 35 years ago by showing that placebo analgesia can be blocked by naloxone. Consistent with this notion, changes in endogenous opioid release have been shown to be involved in placebo-induced analgesia, in which the prefrontal cortex (medial and lateral), as well as the insula and ventral striatum, seem to be involved, and in high placebo responders, increased opioid release in the ventral striatum is positively correlated with pain ratings. Results generally consistent with this brain response pattern have been demonstrated by a number of other groups ; the medial prefrontal/rostral ACC responses to placebo seem to recruit the PAG and amygdala, and involvement of the PAG in placebo-induced analgesia is experimentally observed as well, which links opioid descending modulation with prefrontal cortical control of placebo analgesia. The correspondence between placebo analgesia and reward was studied directly, and the results showed a strong correspondence between the brain regions involved in each.
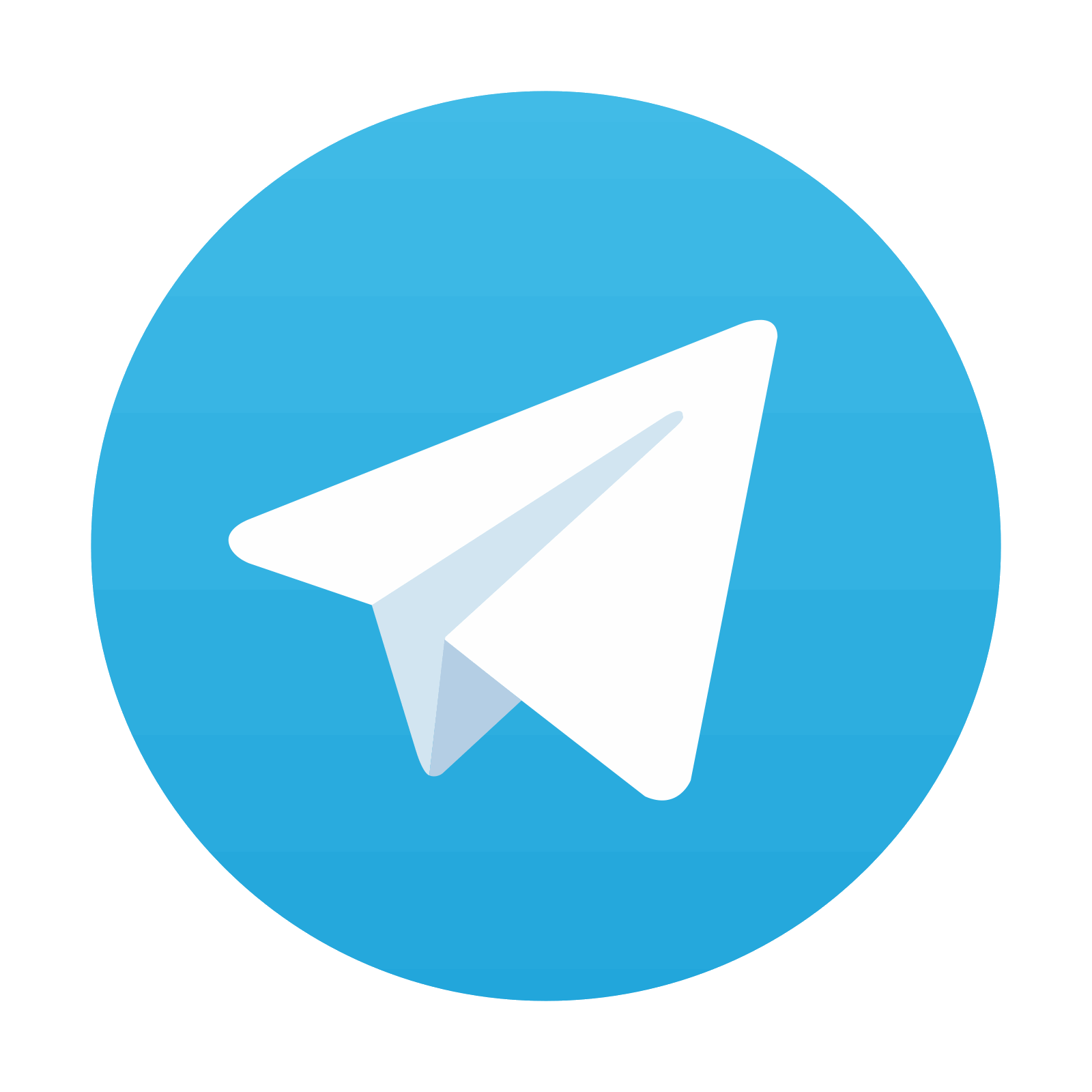
Stay updated, free articles. Join our Telegram channel
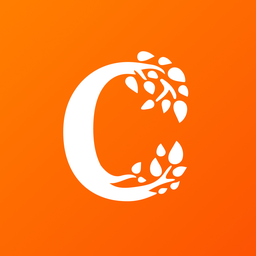
Full access? Get Clinical Tree
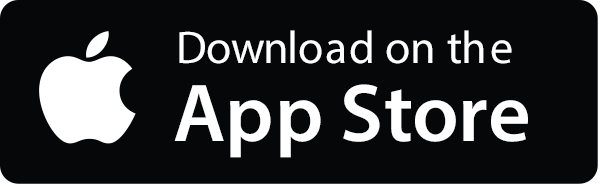
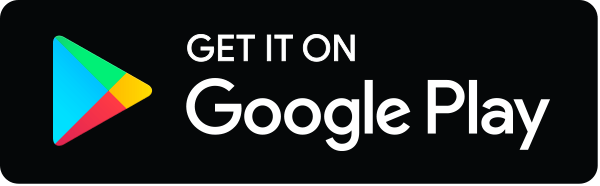