Opioids play an indispensable role in the practice of anesthesiology, critical care, and pain management. A sound understanding of opioid pharmacology, including both basic science and clinical aspects, is critical for the safe and effective use of these important drugs. This chapter will focus almost exclusively on intravenous opioid receptor agonists used perioperatively.
Basic Pharmacology
Structure-Activity
The opioids of clinical interest in anesthesiology share many structural features. Morphine is a benzylisoquinoline alkaloid ( Fig. 9.1 ). Many commonly used semisynthetic opioids are created by simple modification of the morphine molecule. Codeine, for example, is the 3-methyl derivative of morphine. Similarly, hydromorphone, hydrocodone, and oxycodone are also synthesized by relatively simple modifications of morphine. More complex alterations of the morphine molecular skeleton result in mixed agonist-antagonists such as nalbuphine and even complete antagonists such as naloxone.

The fentanyl series of opioids are chemically related to meperidine. Meperidine is the first completely synthetic opioid and can be regarded as the prototype clinical phenylpiperidine (see Fig 9.1 ). Fentanyl is a simple modification of the basic phenylpiperidine structure. Other commonly used fentanyl congeners such as alfentanil and sufentanil are somewhat more complex versions of the same phenylpiperidine skeleton.
Opioids share many physicochemical features in common, although some individual drugs have unique features ( Table 9.1 ). In general, opioids are highly soluble weak bases that are highly protein bound and largely ionized at physiologic pH. Opioid physicochemical properties influence their clinical behavior. For example, relatively unbound, un-ionized molecules such as alfentanil and remifentanil have a shorter latency to peak effect after bolus injection.
Parameter | Morphine | Fentanyl | Sufentanil | Alfentanil | Remifentanil |
---|---|---|---|---|---|
pKa | 8.0 | 8.4 | 8.0 | 6.5 | 7.1 |
% un-ionized at pH 7.4 | 23 | <10 | 20 | 90 | 67 |
Octanol-H 2 O partition coefficient | 1.4 | 813 | 1778 | 145 | 17.9 |
% bound to plasma protein | 20-40 | 84 | 93 | 92 | 80 |
Diffusible fraction (%) | 16.8 | 1.5 | 1.6 | 8.0 | 13.3 |
Vdc (L/kg) | 0.1-0.4 | 0.4-1.0 | 0.2 | 0.1-0.3 | 0.06-0.08 |
Vdss (L/kg) | 3-5 | 3-5 | 2.5-3.0 | 0.4-1.0 | 0.2-0.3 |
Clearance (mL/min/kg) | 15-30 | 10-20 | 10-15 | 4-9 | 30-40 |
Hepatic extraction ratio | 0.6-0.8 | 0.8-1.0 | 0.7-0.9 | 0.3-0.5 | NA |
Mechanism
Opioids produce their main pharmacologic effects by interacting with opioid receptors, which are typical of the G protein–coupled family of receptors widely found in biology (e.g., β-adrenergic, dopaminergic, among others). Expression of cloned opioid receptors in cultured cells has facilitated analysis of the intracellular signal transduction mechanisms activated by the opioid receptors. Binding of opioid agonists with the receptors leads to activation of the G protein, producing effects that are primarily inhibitory ( Fig. 9.2 ); these effects ultimately culminate in hyperpolarization of the cell and reduction of neuronal excitability.

Three classical opioid receptors have been identified using molecular biology techniques: μ, κ, and δ. More recently, a fourth opioid receptor, ORL1 (also known as NOP), has also been identified, although its function is quite different from that of the classical opioid receptors. Each of these opioid receptors has a commonly employed experimental bioassay, associated endogenous ligand(s), a set of agonists and antagonists, and a spectrum of physiologic effects when the receptor is agonized ( Table 9.2 ). Although the existence of opioid receptor subtypes (e.g., μ 1 μ 2 ) has been proposed, it is not clear from molecular biology techniques that distinct genes exist for them. Posttranslational modification of opioid receptors certainly occurs and may be responsible for conflicting data regarding opioid receptor subtypes.
Feature | Mu (μ) | Delta (δ) | Kappa (κ) |
---|---|---|---|
Tissue bioassay a | Guinea pig ileum | Mouse vas deferens | Rabbit vas deferens |
Endogenous ligand | β-Endorphin | Leu-enkephalin | Dynorphin |
Endomorphin | Met-enkephalin | ||
Agonist prototype | Morphine | Deltorphin | Buprenorphine |
Fentanyl | Pentazocine | ||
Antagonist prototype | Naloxone | Naloxone | Naloxone |
Supraspinal analgesia | Yes | Yes | Yes |
Spinal analgesia | Yes | Yes | Yes |
Ventilatory depression | Yes | No | No |
Gastrointestinal effects | Yes | No | Yes |
Sedation | Yes | No | Yes |
a Traditional experimental method to assess opioid receptor activity in vivo.
Opioids exert their therapeutic effects at multiple sites. They inhibit the release of substance P from primary sensory neurons in the dorsal horn of the spinal cord, mitigating the transfer of painful sensations to the brain. Opioid actions in the brainstem modulate nociceptive transmission in the dorsal horn of the spinal cord through descending inhibitory pathways. Opioids are thought to change the affective response to pain through actions in the forebrain; decerebration prevents opioid analgesic efficacy in rats. Furthermore, morphine induces signal changes in “reward structures” in the human brain.
Studies in genetically altered mice have yielded important information about opioid receptor function. In μ opioid receptor knockout mice, morphine-induced analgesia, reward effect, and withdrawal effect are absent. Importantly, μ receptor knockout mice also fail to exhibit respiratory depression in response to morphine.
Metabolism
The intravenously administered opioids in routine perioperative clinical use are transformed and excreted by many metabolic pathways. In general, opioids are metabolized by the hepatic microsomal system, although hepatic conjugation and subsequent excretion by the kidney are important for some opioids. For certain opioids, the specific metabolic pathway involved has important clinical implications in terms of active metabolites (e.g., morphine, meperidine) or an ultra short duration of action (e.g., remifentanil). For other opioids, genetic variation in the metabolic pathway can drastically alter the clinical effects (e.g., codeine). These nuances are addressed in a subsequent section focused on individual drugs.
Clinical Pharmacology
Pharmacokinetics
Pharmacokinetic differences are the primary basis for the rational selection and administration of opioids in perioperative anesthesia practice. Key pharmacokinetic behaviors are (1) the latency to peak effect-site concentration after bolus injection (i.e., bolus front-end kinetics), (2) the time to clinically relevant decay of concentration after bolus injection (i.e., bolus back-end kinetics), (3) the time to steady-state concentration after starting a continuous infusion (i.e., infusion front-end kinetics), and (4) the time to clinically relevant decay in concentration after stopping a continuous infusion (i.e., infusion back-end kinetics).
Applying opioid pharmacokinetic concepts to clinical anesthesiology requires recognition of several fundamental principles. First, a table of pharmacokinetic variables has limited clinical value (see Table 9.1 ). Understanding pharmacokinetic behavior is best achieved through computer simulation. Second, opioids administered by bolus injection or continuous infusion must be considered separately. Third, pharmacokinetic information must be integrated with knowledge about the concentration-effect relationship and drug interactions (i.e., pharmacodynamics) in order to be clinically useful (also see Chapter 4 ).
The latency to peak effect and the offset of effect after bolus injection (i.e., bolus front-end kinetics and bolus back-end kinetics) of various intravenous opioids can be defined by predicting the time course of effect-site concentrations after a bolus is administered. Because the opioids differ in terms of potency (and thus the required dosages), for comparison purposes, the effect-site concentrations must be normalized to the percent of peak concentration for each drug. Considering morphine, fentanyl, sufentanil, alfentanil, and remifentanil as among the opioids most commonly used intraoperatively, pharmacokinetic simulation illustrates how opioids differ in terms of latency to peak effect after a bolus is administered ( Fig. 9.3 , top panel).

The simulation of a bolus injection (see Fig. 9.3 , top panel) has clinical implications. For example, when a rapid onset of opioid effect is desirable, morphine may not be a good choice. Similarly, when the clinical goal is a brief duration of opioid effect followed by rapid dissipation, remifentanil or alfentanil might be preferred. Note how remifentanil’s concentration has declined very substantially before fentanyl’s peak concentration has even been achieved. The simulation illustrates why the front-end kinetics of fentanyl make it a drug well suited for patient-controlled analgesia (PCA) (also see Chapter 39, Chapter 40 ). In contrast to morphine, the peak effect of a fentanyl bolus is manifest before a typical PCA lockout period has elapsed, thus mitigating a “dose stacking” problem (also see Chapter 40 ).
The latency to peak effect is governed by the speed with which the plasma and effect site come to equilibrium (i.e., the ke0 parameter). Drugs with a more rapid equilibration have a higher “diffusible” fraction (i.e., the proportion of drug that is un-ionized and unbound) and high lipid solubility (see Table 9.1 ). However, a very large dose of even a slow onset opioid can produce an apparent rapid onset (because a supratherapeutic drug level in the effect site is reached even though the peak concentration comes later).
The time to steady-state after beginning a continuous infusion is also best examined by pharmacokinetic simulation. Using the same prototypes as with bolus administration, pharmacokinetic simulation ( Fig. 9.3 , middle panel) shows the time required to achieve steady-state effect-site concentrations (i.e., infusion front-end kinetics).
This simulation of simple, constant rate infusions has obvious clinical implications. First, the time required to reach a substantial fraction of the ultimate steady-state concentration is very long in the context of intraoperative use. To reach a near steady-state more quickly requires that a bolus be administered before the infusion is commenced (or increased). Remifentanil perhaps represents a partial exception to this general rule. Also, opioid concentrations will increase for many hours after an infusion is commenced; in other words, concentrations are typically increasing even though the infusion rate may have been the same for hours! That remifentanil achieves a near steady-state relatively quickly is certainly part of why it has emerged as a popular drug for total intravenous anesthesia (TIVA).
The time to offset of effect after stopping a steady-state infusion is best expressed by the context-sensitive half-time (CSHT) simulation. Defined as the time required to achieve a 50% decrease in concentration after stopping a continuous, steady-state infusion, the CSHT is a means of normalizing the pharmacokinetic behavior of drugs so that rational comparisons can be made regarding the predicted offset of drug effect. The CSHT is thus focused on “infusion back-end” kinetics.
The bottom panel of Fig. 9.3 is a CSHT simulation for commonly used opioids. For most drugs, the CSHT changes with time. Thus, for brief infusions, the predicted back-end kinetics for the various drugs do not differ much (remifentanil is a notable exception to this general rule). As the infusion time lengthens, the CSHTs begin to differentiate, providing a rational basis for drug selection. Second, depending on the desired duration of opioid effect, either shorter-acting or longer-acting drugs can be chosen. Finally, the shapes of these curves differ depending on the degree of concentration decline required. In other words, the curves representing the time required to achieve a 20% or an 80% decrease in concentration (e.g., the 20% or 80% decrement time simulations) are quite different. Thus, depending on the anesthesia technique applied, the CSHT simulations are not necessarily the clinically relevant simulations (i.e., a 50% decrease may not be the clinical goal). Also, CSHT simulation for morphine does not account for active metabolites (see later discussion of individual drugs under “Unique Features of Individual Opioids”).
Pharmacodynamics
In most respects, the μ-agonist opioids can be considered pharmacodynamic equals with important pharmacokinetic differences; that is, both the therapeutic and adverse effects are essentially the same. Their efficacy as analgesics and their propensity to produce ventilatory depression are indistinguishable from each other. Pharmacodynamic differences do exist with nonopioid receptor mechanisms such as histamine release.
Because the nervous system profoundly influences the function of the entire body, opioid μ-agonist pharmacodynamic effects are observed in many organ systems. Fig. 9.4 summarizes the major pharmacodynamic effects of the fentanyl congeners. Depending on the clinical circumstances and clinical goals of treatment, some of these widespread effects can be viewed as therapeutic or adverse. For example, in some clinical settings the sedation produced by μ-agonists might be viewed as a goal of therapy. In others, drowsiness would clearly be thought of as an adverse effect.

Therapeutic Effects
The relief of pain is the primary therapeutic effect of opioid analgesics. Acting at spinal and brain μ-receptors, opioids provide analgesia both by attenuating the nociceptive traffic from the periphery and also by altering the affective response to painful stimulation centrally. μ-Agonists are most effective in treating “second pain” sensations carried by slowly conducting, unmyelinated C fibers; they are less effective in treating “first pain” sensations (carried by small, myelinated A-delta fibers) and neuropathic pain. A unique aspect of opioid-induced analgesia (in contrast to drugs like local anesthetics) is that other sensory modalities are not affected (e.g., touch, temperature, among others).
Perioperatively (certainly intraoperatively), the drowsiness produced by μ-agonists is also one of the targeted effects. The brain is the anatomic substrate for the sedative action of μ-agonists. With increasing doses, μ-agonists eventually produce drowsiness and sleep (the relief of pain no doubt contributes to the promotion of sleep in uncomfortable patients both pre- and postoperatively). With sufficient doses, the μ-agonists produce pronounced delta wave activity on the electroencephalogram, which resembles the pattern observed during natural sleep.
μ-Agonists can of course produce significant relief of pain by doses that do not produce sleep. This is the clinical basis for their use in the treatment of pain in ambulatory patients. Yet, the administration of additional doses eventually produces drowsiness (and, as a consequence, the inability to request additional doses) and is the essential scientific foundation for the safety of PCA devices (also see Chapter 40 ). However, even large doses of opioids do not reliably produce unresponsiveness and amnesia and thus opioids cannot be viewed as complete anesthetics when used alone.
Opioids also suppress the cough reflex via the cough centers in the medulla. Attenuation of the cough reflex presumably makes coughing and “bucking” against the indwelling endotracheal tube less likely.
Adverse Effects
Depression of ventilation is the primary adverse effect associated with μ-agonist drugs. When the airway is secured and ventilation is controlled intraoperatively, opioid-induced depression of ventilation is of little consequence. However, opioid-induced respiratory depression in the postoperative period can lead to brain injury and death (also see Chapter 39 ).
μ-Agonists alter the ventilatory response to arterial carbon dioxide concentrations at the ventilatory control center in the medulla. The depression of ventilation is mediated by the μ-receptor; μ-receptor knockout mice do not exhibit respiratory depression from morphine.
In unmedicated humans, increases in arterial carbon dioxide partial pressure markedly increase minute volume ( Fig. 9.5 ). Under the influence of opioid analgesics, the curve is flattened and shifted to the right for a given carbon dioxide partial pressure and reflecting that the minute volume is smaller. More importantly, the “hockey stick” shape of the normal curve is lost; that is, there may be a partial pressure of carbon dioxide below which the patient will not breathe (i.e., the “apneic threshold”) in the presence of opioids.

The clinical signs of depressed ventilation are quite subtle with moderate opioid doses. Postoperative patients receiving opioid analgesic therapy can be awake and alert and yet have a significantly decreased minute volume. Respiratory rate (often associated with a slightly increased tidal volume) also decreases. As the opioid concentration is increased, the respiratory rate and tidal volume progressively decrease, eventually culminating in an irregular ventilatory rhythm and then complete apnea.
Many factors can increase the risk of opioid-induced ventilatory depression. Clear risk factors include large opioid dose, advanced age, concomitant use of other central nervous system (CNS) depressants, and renal insufficiency (for morphine). Natural sleep also increases the ventilatory depressant effect of opioids.
Opioids can alter cardiovascular physiology by a variety of different mechanisms. Compared to many other anesthetic drugs (e.g., propofol, volatile anesthetics), however, the cardiovascular effects of opioids, particularly the fentanyl congeners, are relatively minimal (morphine and meperidine are exceptions—see the following section on individual drugs).
The fentanyl congeners cause bradycardia by directly increasing vagal nerve tone in the brainstem, which experimentally can be blocked by microinjection of naloxone into the vagal nerve nucleus or by peripheral vagotomy.
Opioids also produce vasodilation by depressing vasomotor centers in the brainstem and to a lesser extent by a direct effect on vessels. This action decreases both preload and afterload. Decreases in arterial blood pressure are more pronounced in patients with increased sympathetic tone such as patients with congestive heart failure or hypertension. Clinical doses of opioids do not appreciably alter myocardial contractility.
Opioids can induce muscle rigidity, usually from the rapid administration of large bolus doses of the fentanyl congeners. This rigidity can even make ventilation via a bag and mask during induction of anesthesia nearly impossible because of vocal cord rigidity and closure. The appearance of rigidity tends to coincide with the onset of unresponsiveness. Although the mechanism of opioid-induced muscle rigidity is unknown, it is not a direct action on muscle because it can be eliminated by the administration of neuromuscular blocking drugs.
Opioids also cause nausea and vomiting. Opioids stimulate the chemoreceptor trigger zone in the area postrema on the floor of the fourth ventricle in the brain. This can lead to nausea and vomiting, which are exacerbated by movement (this is perhaps why ambulatory surgery patients are more likely to be troubled by postoperative nausea and vomiting, PONV) (also see Chapter 37 ).
Pupillary constriction induced by μ-agonists can be a useful diagnostic sign indicating some ongoing opioid effect. Opioids stimulate the Edinger-Westphal nucleus of the oculomotor nerve to produce miosis. Even small doses of opioid elicit this response and very little tolerance to the effect develops. Thus, miosis is a useful, albeit nonspecific indicator of opioid exposure even in opioid-tolerant patients. Opioid-induced pupillary constriction is naloxone reversible.
Opioids have important effects on gastrointestinal physiology. Opioid receptors are located throughout the enteric plexus of the bowel. Stimulation of these receptors by opioids causes tonic contraction of gastrointestinal smooth muscle, thereby decreasing coordinated, peristaltic contractions. Clinically, this contraction results in delayed gastric emptying and presumably larger gastric volumes in patients receiving opioid therapy preoperatively. Postoperatively, patients can develop opioid-induced ileus that can potentially delay the resumption of proper nutrition and discharge from the hospital. An extension of this acute problem is the chronic constipation associated with long-term opioid therapy.
Similar effects are observed in the biliary system, which also has an abundance of μ-receptors. μ-Agonists can produce contraction of the gallbladder smooth muscle and spasm of the sphincter of Oddi, potentially causing a falsely positive cholangiogram during gallbladder and bile duct surgery. These effects are completely naloxone reversible and can be partially reversed by glucagon treatment.
Although the urologic effects are minimal, opioids can sometimes cause urinary retention by decreasing bladder detrusor tone and by increasing the tone of the urinary sphincter. These effects are in part centrally mediated, although peripheral effects are also likely given the widespread presence of opioid receptors in the genitourinary tract. Although the urinary retention associated with opioid therapy is not typically pronounced, it can be troublesome in males, particularly when the opioid is administered intrathecally or epidurally.
Opioids depress cellular immunity. Morphine and the endogenous opioid β-endorphin, for example, inhibit the transcription of interleukin 2 in activated T cells, among other immunologic effects. Individual opioids (and perhaps classes of opioids) may differ in terms of the exact nature and extent of their immunomodulatory effects. Although opioid-induced impairment of cellular immunity is not well understood, impaired wound healing, perioperative infections, and cancer recurrence are possible adverse outcomes.
Drug Interactions
Drug interactions can be based on two mechanisms: pharmacokinetic (i.e., when one drug influences the concentration of the other) or pharmacodynamic (i.e., when one drug influences the effect of the other). In anesthesia practice, although unintended pharmacokinetic interactions sometimes occur, pharmacodynamic interactions occur with virtually every anesthetic and are often produced by design.
The most common pharmacokinetic interaction in opioid clinical pharmacology is observed when intravenous opioids are combined with propofol. Perhaps because of the hemodynamic changes induced by propofol and their impact on pharmacokinetic processes, opioid concentrations may be larger when given in combination with a continuous propofol infusion.
The most important pharmacodynamic drug interaction involving opioids is the synergistic interaction that occurs when opioids are combined with sedatives. When combined with volatile anesthetics, opioids reduce the minimum alveolar concentration (MAC) of a volatile anesthetic ( Fig. 9.6 ). Careful examination of “opioid-MAC reduction” data reveals several clinically critical concepts (see Fig. 9.6 ). First, opioids synergistically reduce MAC. Second, the MAC reduction is substantial (as much as 75% or more). Third, most of the MAC reduction occurs at moderate opioid levels (i.e., even modest opioid doses substantially reduce MAC). Fourth, reduction of MAC is not complete (i.e., opioids are not complete anesthetics). The addition of the opioid cannot completely eliminate the need for the other anesthetic. And fifth, there are an infinite number of hypnotic-opioid combinations that will achieve MAC (this implies that clinicians must choose the optimal combination based on the goals of the anesthetic and operation). All of these concepts also apply when opioids are used in combination with propofol for TIVA.
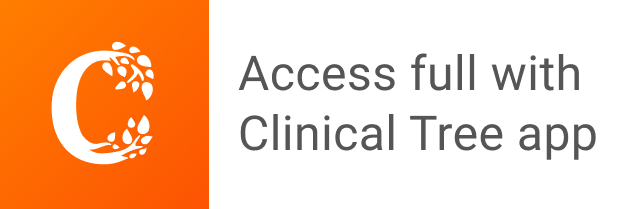