“Among the remedies which it has pleased almighty God to give to man to relieve his sufferings, none is so universal and efficacious as opium.”
—Sir Thomas Sydenham, 1680Analgesics are drugs that relieve pain without significant loss of other sensations. The word analgesic is from the Greek an, without + algesis, sensation of pain. Opioid analgesics are the most effective and commonly used pharmacotherapy for moderate to severe pain.1 Not all pain patients benefit from opioid therapy; however, more patients benefit from strong analgesics when the drugs are used as an element of multimodal therapy.
Opioids include all compounds that bind to opioid receptors; these include exogenous opioid receptor agonists and antagonists as well as the endogenous opioid peptides. The term opiate originally was used to describe a drug derived from opium but now includes the natural opium products (e.g., morphine), the semisynthetic derivatives (e.g., hydromorphone), and completely synthetic congeners (e.g., methadone).
The differences between exogenous opioids, such as morphine, and endogenous opioids, such as β-endorphin, do not justify differing terminology. Opioid accurately describes both types of compounds and is generally considered the preferred term in both clinical and scientific dialogue.
Narcotic, from the Greek narkotikos, benumbing, was originally used to describe opium derivatives. Today, narcotic has become a legal term that includes a broad range of sedating and potentially abused drugs, many of which are not related to opium. Because the word narcotic has negative connotations, physicians should not use it when talking with patients.
The last 30 years have seen a marked increase in our knowledge of the sites and mechanisms of action of opioids.1 New analytical methods reveal pharmacokinetic (PK) data on the disposition and fate of opioids in humans. Recently elucidated opioid receptor-related genetic polymorphisms provide a greater understanding of how genetics can help predict responses to opioids.2
The word opium is derived from the Greek opion, poppy juice. Opium is the milky exudation derived from the unripe capsules of the opium poppy, Papaver somniferum. Opium contains over 20 different alkaloids and has been used to control human discomfort for over five millennia. Opium was used clinically in the early days of European medicine, but it fell into disfavor because of toxic outcomes from nonstandardized forms that were not used with necessary care. Paracelsus repopularized the use of opium in the 16th century, and by the second half of that century, clinical use of opium was understood and adopted by physicians throughout the continent.
The French pharmacist Jean-François Derosne isolated a crystalline precipitate from opium in 1803, a mixture of morphine and narcotine. It was not until 3 years later that the German pharmacist Friedrich Wilhelm Sertürner isolated morphine, the pure alkaloid, and named it after Morpheus, the Greek god of dreams. Other opium alkaloids including papaverine and codeine were soon isolated, and within a few decades, the pure alkaloids replaced crude opium in clinical practice.
By the mid-19th century, many of the patent medicines sold as panaceas contained opioids. Steadily increasing federal and state control of opioids in the United States began with the passage of the Harrison Narcotic Act in 1914. The federal Bureau of Narcotics was created in the 20th century to address use of potentially abused drugs. In recent decades, political and law enforcement demands, rather than scientific or clinical findings, have defined the control system for opioid use in the United States. Creation of the Drug Enforcement Administration (DEA) by the Controlled Substances Act of 1970 assured that control of opioids and other controlled substances would be driven by law enforcement, not a patient care focus. Numerous initiatives, both within and outside of government, have worked to emphasize that opioids are important and frequently underused medications. These include the 1979 Report to the White House of the federal Interagency Committee on New Therapies for Pain and Discomfort, the 1985 report of the federal Interagency Committee on Pain and Analgesia,3 and the 1986 National Institutes of Health Consensus Development Conference Report titled “The Integrated Approach to the Management of Pain.”4 It may be that serious opioid underuse in the latter decades of the 20th century has been replaced by overuse in the early 21st century.5
It is not logical that the human body should contain specific receptors for alkaloids derived from a plant. The presence of endogenous opioids was postulated to explain the presence of opioid receptors before the endogenous substances were isolated. Cloning studies have identified three groups of endogenous opioid peptides; the enkephalins, endorphins, and dynorphins appear to function as neurotransmitters, neuromodulators, and neurohormones. These peptides are found within the central nervous system (CNS), adrenal medulla, nerve plexi, gastric exocrine glands, and intestines.
Opioid drugs manifest analgesic effects primarily by binding to and activating (agonizing) opioid receptors. Opioid receptors are found in the CNS and gastrointestinal (GI) tract and, to a lesser degree, in peripheral tissues. The interaction of exogenous opioid medications and opioid receptors mimics the interaction when endogenous opioid peptides (dynorphins, endorphins, enkephalins) bind with these same receptors.6 The three major classes of opioid receptors are the mu (μ), delta (δ), and kappa (κ) receptors.7 Sigma (σ) and epsilon (∊) receptors were formerly classified as opioid receptors because opioids can bind to them. However, neither is currently considered an opioid receptor because activation does not necessarily result in analgesia and neither of these receptor types is opioid-specific.
Opioid receptors are composed of glycoproteins found in cellular membranes. These receptors are coupled to G proteins that modulate potassium and calcium ion conduction8 (Fig. 68-1). At μ– and δ-opioid receptors, opioids open a potassium ion channel, increasing potassium conductance. Hyperpolarization inhibits neuronal activity. In contrast, κ-receptor activation inhibits calcium entry via a calcium ion channel. Activation of opioid receptors decreases transmission of signals from primary peripheral afferent sensory neurons to higher CNS centers, as well as the processing of the pain stimulus.
FIGURE 68-1.
Illustration of synapse A δ and C fibers with second-order neurons in the dorsal horn of the spinal cord and proposed opioid receptor demonstrating the G protein subunits and close approximation to an ion channel. (Adapted with permission from Sabbe and Yaksh.17)

Administration of an opioid agonist activates opioid receptors, producing both analgesia and adverse effects. The three opioid receptor types have known subtypes (Table 68-1). Two μ subtypes have been elucidated. Activation of μ1 leads to supraspinal analgesia, whereas μ2 activation is associated with the adverse sequelae of opioid administration. Activation of κ and δ receptors produces spinal analgesia. However, κ3 receptors are thought to mediate supraspinal analgesia.9 Activation of δ receptors may potentiate μ-receptor-induced analgesia, but no δ agonists are available for clinical use. Clinical implications of the δ– and κ-receptor subtypes are not fully understood.
Opioid Receptors, Subtypes, and Physiologic Effects
Receptor | Subtypes | Effects |
µ | µ 1 | Supraspinal analgesia |
µ 2 | Physical dependence | |
Euphoria | ||
Sedation | ||
Respiratory depression | ||
Constipation | ||
Orthostatic hypotension | ||
Arteriolar/venous vessel dilation | ||
Delta | Delta 1, 2 | Spinal analgesia |
Euphoria | ||
Potentiates µ receptor analgesia | ||
κ | κ 1, 2, 3 | Spinal analgesia |
Sedation | ||
Miosis | ||
Supraspinal analgesia (K3) |
Peripheral μ and κ opioid receptors in peripheral tissues can effect inflammation and exert antihyperalgesic activity.10 Topical and intraarticular opioids have been used to treat pain in soft tissue and joints. The clinical significance of the peripheral effects of opioids is not yet well understood.
Most opioids used as analgesics are µ agonists which bind primarily at µ opioid receptors (MORs) and also may bind to a lesser degree at κ receptors. Kappa opioid receptor ligands provide a few drugs; however, their clinical pharmacology is complicated by concurrent µ opioid receptor antagonist activity. Delta opioid receptor ligands have been in clinical investigation for years, but none was available for clinical use at the time of this writing. Most analgesics which are predominantly µ agonists also have some ability to bind at κ receptors. Some recent literature has suggested that µ agonists with some preferential κ binding capacity may be advantageous in visceral pain. Oxycodone displays that characteristic.11
Opioid receptors generate nerve signals through a second messenger, cyclic adenosine monophosphate (cAMP), or an ion channel.1 Alterations in the levels of cAMP and the transcription factor CREB (cAMP response element binding protein) during chronic morphine treatment are associated with numerous cellular changes, some of which can produce tolerance and physical dependence.12 Molecular genetics approaches have used gene-targeting (knockout) technology to disrupt the gene that codes for each of the three types of opioid receptors. Mice given morphine that lack the µ opioid receptor (MOR-deficient mice) do not experience analgesia, respiratory depression, constipation, physical dependence, reward behaviors, or immunosuppression.13 µ opioid receptors are evident in the periphery following inflammation, in presynaptic and postsynaptic spinal cord dorsal horn sites, and in the brainstem, thalamus, and cortex. These locations constitute the ascending pain transmission system. These receptors also reside in midbrain periaqueductal gray, the nucleus raphe magnus, and the rostral ventral medulla. Those sites constitute the descending inhibitory system that modulates spinal cord pain transmission.14 µ agonists bind at MORs in the colon, causing opioid-induced constipation.15 Opioids also decrease presynaptic neurotransmitter release through calcium channel inhibition and increase potassium ion efflux, causing hyperpolarization of postsynaptic neurons and decreasing synaptic transmission. An additional mechanism of action of opioids is inhibition of GABAergic transmission within the CNS. The net effect is descending inhibitory circuit excitation.
Animal studies suggest that the reinforcing and rewarding properties of opioids (e.g., euphoria) that are associated with opioid abuse involve the mesolimbic dopamine system, not the supraspinal systems most prominently involved in the production of analgesia and physical dependence.16
Pain relief from µ agonist opioids is relatively selective; other senses are not affected. After opioid administration, some patients still perceive pain, but they generally report it as being less uncomfortable than it was before receiving the drug. Pain includes both sensory-discriminative aspects (e.g., perception of the location, type, and intensity) and an emotional dimension sometimes described as unpleasantness. Sensory-discriminative aspects are processed in the somatosensory cortex (SSC), while the affective component is processed in the anterior cingulate cortex (ACC). µ opioid receptors (MORs) are found in both of these areas of the brain. Non-opioid analgesics have dose-dependent analgesic effect ceilings; morphine-like opioids do not. However, dose-related adverse events produce a functional ceiling. These include sedation, obtundation, nausea, vomiting, and respiratory depression. The clinical dose needed for analgesia varies with the type of pain and among patients. As result, the most important clinical principle is titration of the opioid dose to response. Some patients will prefer less analgesia to retain a more intact sensorium; others prefer mental clouding if it is accompanied by pain relief.
µ agonists can alter mood, including the relief of anxiety, euphoria, and—more commonly dysphoria. Chronic pain patients often report initial relief of depression, at least some of which may be due to decreased pain, which frequently proceeds to worsened depression in days to weeks. Reinforcing and rewarding properties of opioids in opioid abusers involve the mesolimbic dopamine system and differ from the systems which produce analgesia and physical dependence.
µ agonists produce nausea and vomiting by stimulating the chemoreceptor trigger zone (CTZ) in the medullary area postrema. These adverse effects occur more frequently in ambulatory patients due to motion exacerbating vestibular sensitivity. Opioid-induced nausea and vomiting varies among drugs and among patients. Patients who cannot tolerate GI adverse effects from one opioid may tolerate another and find tapentadol better tolerated than pure µ agonists. Central antiemetics such as phenothiazines (e.g., prochlorperazine), a gastrokinetic drug (e.g., metoclopramide), or a 5-HT receptor antagonist (e.g., ondansetron) can be helpful.
µ agonists are sedating and these effects are additive to those of other CNS depressants. Opioids are additive to respiratory depressants including alcohol, benzodiazepines, and other sedative-hypnotics. A central stimulant (e.g., methylphcnidate, modafinil) helps to counteract opioid sedative effects. Tolerance to opioid-induced sedation typically occurs to some degree within a few days.
Respiratory depression with initiation of opioid pharmacotherapy for acute pain is potentially the most serious adverse opioid effect. The µ agonists act at brainstem respiratory centers. The effect is dose-related and sensitivity varies among individuals. Therapeutic morphine doses can depress respiratory rate, minute volume, and tidal exchange. As CO2 accumulates, it stimulates central chemoreceptors, causing a compensatory increase in respiratory rate that masks respiratory depression. Respiratory depression and CO2 retention produce cerebral vasodilation and increased cerebrospinal fluid pressure unless the PCO2 is normalized by artificial ventilation. Respiratory depression occurs most commonly in opioid-naive patients after acute opioid administration and is associated with other signs of CNS depression, including sedation and obtundation. Tolerance to these effects develops rapidly, and the risk of the effects is relatively low with chronic opioid use in chronic pain management. Obstructive sleep apnea is common in obese patients and presents risk for opioid-induced respiratory depression, especially when the patients are sleeping. Opioids, and perhaps more so methadone, may induce potentially life-threatening central sleep apnea Respiratory depression can be reversed by prompt administration of the opioid antagonist naloxone. In patients chronically receiving opioids who develop respiratory depression, naloxone diluted 1:10 should be titrated carefully to prevent the precipitation of severe withdrawal symptoms while reversing the respiratory depression.
Three mixed agonist-antagonists, pentazocine, nalbuphine, butorphanol, and the partial agonist buprenorphine have different dose-response characteristics for their respiratory depression curves from the morphine-like opioids. While therapeutic doses of pentazocine produce respiratory depression equivalent to that of morphine, increasing the dose does not ordinarily produce a proportional increase in respiratory depression. Whether this apparent ceiling to respiratory depression offers any significant clinical advantage has not been determined. Naloxone reversal of the respiratory depression produced by buprenorphine requires relatively large doses (5-10 mg) and is delayed in onset.
Opioids produce miosis through stimulation of the parasympathetic nucleus of the oculomotor nerve. Pinpoint pupils, respiratory depression, and loss of consciousness are the three signs of opioid overdose. All of these effects can be reversed with naloxone. Severe anoxia causes mydriasis.
Opioids depress the cough centers in the medulla, depressing the cough reflex. Different mechanisms depress cough than produce analgesia. As a result, the dextrorotatory isomers of opioids (e.g., dextromethorphan), which do not bind to opioid receptors, are antitussive.
Morphine alters hypothalamic heat regulation, lowering body temperature. Corticotrophin-releasing hormone (CRH) and gonadotropin-releasing hormone (GnRH) are inhibited, resulting in lowered luteinizing hormone (LH), follicle stimulating hormone (PSH), and adrenocorticotropic hormone (ACTH). Prolactin and antidiuretic hormone release are increased. Tolerance to these effects appears to occur.
Opioid-induced androgen deficiency (OPIAD) describes clinically meaningful decrease in testosterone levels that occurs in the majority of men receiving ongoing morphine or other potent µ agonist opioid pharmacotherapy. Worsening of pain, depressed mood, and refractoriness to treatment are common in such patients. Trials of androgen supplements have been helpful and this phenomenon also appears to occur in women, presumably due to progesterone suppression.
In addition to being CNS depressants, opioids can be excitatory. Normeperidine, a major metabolite of meperidine produces anxiety, tremors, myoclonus, and generalized seizures when it accumulates with repeated dosing. Naloxone does not reverse, and may even exacerbate, this hyperexcitability. Other high-dose µ agonists can produce multifocal myoclonus in opioid-tolerant patients.18
Opioids remain the most effective analgesics available; as such, they are essential in clinical medicine for the management of moderate to severe pain in many cases. However, the misuse of prescription opioids resulting in morbidity and mortality has produced controversy over opioid availability and control.19 Opioids are clinically useful in treating diarrhea and GI hypermotility because activation of opioid receptors in the intestines slows peristalsis. Opioids decrease respiratory activity which can be useful in anesthetized patients and to help manage air hunger, especially in end-of-life care. Opioids also can lessen anxiety and excitation and induce a feeling of well-being.
Much opioid pharmacology learned by health care professional students is based on studies conducted in lower animals or isolated tissues. Those rarely address the profound cognitive effects of pain and analgesia that greatly influence outcomes of opioid therapy in humans. Serious misunderstanding among health care providers results from failure to recognize the great differences between acute opioid toxicity and that seen with long-term therapy. Incorrect assumptions and beliefs about opioid toxicity, addiction potential, and tolerance to analgesic effects have led to poor use of these effective drugs in many clinical settings. This has led to widespread opiophobia, the irrational and undocumented fear that appropriate use of opioids causes addiction.20
Balance in opioid availability and use is essential for good patient care outcomes and the public health. Well-designed guidelines have helped to define that balance.21–23
Opioid pharmacotherapy for chronic nonmalignant pain was considered controversial by many physicians well into the 1990s despite numerous published studies that documented the safety and efficacy of opioids in the management of a variety of chronic nonmalignant pain states, such as neuropathic, myofascial, arthritic, and osteoporosis pain.24 In 2002, the Federation of State Medical Boards of the United States published “Model Policy for the Use of Controlled Substances in the Treatment of Pain.”25 This authoritative publication clearly documents that opioids have a place in the management of many patients’ chronic nonmalignant pain.
Many physicians are more willing to use opioids in patients with cancer pain than noncancer pain. The fact that opioids are seriously underused was underscored by a study of opioid prescribing for cancer pain patients that was published in the Journal of the American Medical Association in 1998.26 Researchers evaluated the records of 13,625 cancer patients discharged from hospitals to Medicare or Medicaid certified nursing homes during the 4-year period 1992 to 1995 in five states. A total of 4003 patients reported and had multiple factors independently associated with daily pain. Only 26% of patients received morphine or another opioid considered to be on level 3 of the World Health Organization (WHO) analgesic ladder27 (Fig. 68-2). Only 32% of patients received a step 2 analgesic, and 26% received only acetaminophen or a nonsteroidal anti-inflammatory drug (NSAID) for analgesia.
The WHO analgesic ladder that was developed to guide analgesic therapy for cancer patients in developing countries has been shown to be applicable in most pain management in most societies. This approach describes three levels of analgesia. It suggests that pharmacologic management of mild to moderate pain should include a NSAID or acetaminophen unless there is a contraindication as a first step. When pain persists or increases, add an opioid as the second step. The third step consists of increasing the opioid dose to treat persistent moderate to severe pain. This is not a stepped approach in which prior steps must be tried before initiating more aggressive therapy. Analgesia should be started at the level appropriate for the patient’s pain. For cancer pain, a fourth step including palliative radiation and chemotherapy, nerve blocks, and various other modalities exists in developed countries.
Equianalgesic dose tables often use parenteral morphine 10 mg every 4 hours as the standard for comparison. Equianalgesic doses are listed in Table 68-2. The dose tables will not apply to all patients because of interpatient variances in response to opioids. The tables do provide an approximation of equally effective doses, but patients must often be retitrated to response when the opioids they are taking are changed. It is important to differentiate among the pure µ-opioid receptor agonists, mixed agonist-antagonists (which are κ-opioid receptor agonists and either antagonistic or neutral at µ receptors), and partial agonists. There is no proven difference in analgesic activity among the pure µ agonists. Greater potency does not imply higher activity. Potency differences simply mean that different amounts of drug must be used to obtain the same activity. PK differences can be clinically important.
Dosing Comparison for Common Opioids
Drug | Approximate Equianalgesic Oral Dose (mg) | Approximate Equianalgesic IM Dose (mg) | Approximate Time to Onset PO-parenteral (minutes) | Dosing Interval (hours) | |
Morphine | 30 Regular schedule 60 PRN dosing | 10 | 20 | 15 | 4-6 |
Methadone | 20 | 10 | 30 | 20 | 6-8 |
Levorphanol | 4 | 2 | 30 | 20 | 6-8 |
Hydromorphone | 4–6 | 1.5–2 | 20 | 15 | 4 |
Meperidine | 150–250* | 75–100 | 15 | 10 | 2.5-3.5 |
Codeine | 75 | 20 | 15 | 4-6 | |
Buprenorphine | not available | 0.3–0.4** | — | 25 | 6-8 |
Butorphanol | not available | 2** | — | 20 | 3-4 |
Nalbuphine | not available | 10** | — | 20 | 3-6 |
Pentazocine | 150** | 60** | 20 | 15 | 3-6 |
Oxycodone | 10 mg is clinically equivalent to 10–20 mg of oral morphine. The maximum safe daily dose of oxycodone 5 mg/acetaminophen 325 mg is 12 tablets (~4 g of acetaminophen) due to potential for hepatotoxicity. Plain oxycodone can be titrated to higher doses much like morphine. OxyContin labeling recommends a dose ratio of oxycodone to morphine of 1:2. A ratio of 3:4 may be more accurate | ||||
Fentanyl, transdermal | a 50 μg/hr patch provides similar analgesia to 10 mg of oral morphine on a regular q 4-hr schedule which is equal to 30 mg of sustained acting morphine administered q 12 hr. Patients with fever (e.g., tumor fever) may get only about 48 hr of analgesia from a patch; fever or exogenous heat, 9 (e.g., heat lamps, electric blankets) may accelerate drug release. The patch requires a mean of 17 hr to reach peak blood levels and effects remain for 12-24 hr after removing a patch. Therefore, patches cannot be used effectively to titrate doses. |
Pharmacokinetic parameters to consider include peak serum level (Cmax), time to peak serum level (Tmax), and elimination half-life (T1/2). Oral-to-parenteral dose ratios also vary among opioids (Table 68-3). Also, differences in metabolism (biotransformation) may sometimes make one opioid preferable to another, especially in a patient with impaired metabolism or elimination. Opioids may be long acting due to inherent pharmacologic factors, for example, methadone, levorphanol, or a pharmaceutical formulation. Each type has advantages and disadvantages. The PKs of commonly used opioids have been well described at all phases of human life.28–30
Opioid Metabolites*
Parent Drug (% excreted unchanged) | Analgesic Duration (hours) | Metabolites (% if known) | Metabolite Half-lives (hours) | Metabolite Route Elimination | Comment |
Morphine (~7.2% – IV) (~3.7% – PO) | 4-6 |
|
|
| Elimination of morphine is not affected by renal failure, however, volume of distribution may be smaller, resulting in increased plasma concentrations; enterohepatic circulation of morphine and glucuronides occurs |
|
| Morphine-3-glucuronide (57-74) | 2.8-4 | Renal | Half-life is 41-141 hor in renal failure; 5-20 times more potent than morphine in causing hyperalgesia, EEG spiking, agitation, seizures in animals; possibly by a non-opioid receptor mechanism |
|
| Morphine-6-glucuronide (4.7-12) | Duration of action 2 times longer than parent | Renal | Half-life is 89-136 hr in renal failure; may be responsible for narcosis in patients with renal failure; by IT administration is 100 times more potent than morphine; accumulates with chronic dosing |
|
| Morphine-3-ethereal sulfate (5-10) |
|
|
|
|
| Normorphine (3.5) |
|
| May have toxic effects (myoclonus, allodynia) |
|
| morphine-N-oxide |
|
|
|
Codeine 11.1% | 4-6 |
|
|
|
|
|
| Codeine-6-glucuronide |
|
| Primary elimination pathway; profound narcosis has occurred in chronic renal failure |
|
| Norcodeine |
| Renal | Equipotent to codeine in analgesic activity |
|
| Morphine (10) |
|
| May account for analgesic activity of codeine |
Fentanyl (<10) | 1-2 |
|
|
| May be extensively liver metabolized |
|
| Norfentanyl |
| Renal, hepatic | Metabolized to despropionyl fentanyl |
|
|
|
|
| May cause neurotoxic adverse effects |
|
|
|
|
| Structurally similar to normeperidine |
|
| 4-N-anilinopiperidine |
|
|
|
Hydromorphone (5.6%) |
|
|
|
| Clearance dependent on hepatic blood flow |
|
| Hydromorphone-3-glucuronide |
|
| Shown to accumulate in renal failure in one patient |
|
| Hydromorphone-6-glucuronide |
| Renal | Formed from intermediate metabolites, dihydroisomorphine and dihydromorphine |
|
| Nor-metabolites |
|
| Significance not known |
Levorphanol | 6-8 | Levorphanol glucuronide |
| Renal | Liver metabolized by glucuronide conjugation |
Meperidine | 2.5-3.5 |
|
|
| Bioavailability increases from 50%- 80% in cirrhosis |
5% (uncontrolled urine pH) |
|
|
|
| Half-life of meperidine and normeperidine prolonged in cirrhosis |
|
|
|
|
| Urinary pH effects elimination of unchanged meperidine in urine 25% in acidic urine vs. 1%-2% in alkaline urine |
|
| Normeperidine (5-30) | 15-30 | Renal, hepatic | Half-life prolonged significantly in renal failure (>30 hr); twice as potent in CNS stimulatory effects as meperidine; one-half the analgesic effect of meperidine; urinary excretion pH dependent: uncontrolled pH fe = 0.05-0.06, acidic urine fe = 0.30, alkaline urine fe <0.031 |
|
| Meperidinic acid |
|
| Inactive metabolite |
Methadone 21% (acidic urine increases the fraction of elimination [fe]) | 4-6 initially; |
|
|
| In one anephric patient, 98% of methadone was found in feces as metabolite, suggesting a shift in metabolism from renal to fecal urinary excretion of methadone and metabolites is dose dependent and is the major route of elimination in doses >55 mg/d; 10%-45% of methadone is eliminated in feces as metabolites |
| 6-12 after steady state (1-2 d) |
|
|
|
|
|
| 1,5-demethyl-2-ethyl-3,3-iphenyl-1-pyrroline |
| Renal, biliary | Unpredictable half-life with chronic dosing long-term analgesia is 10 times that of morphine; major metabolite fe = 0.30 |
|
| 2-ethyl-5-methyl-3,3-diphenyl-1-pyrroline |
| Renal, biliary | Minor metabolite |
|
| Methadone-N-oxide |
|
| Minor metabolite |
Oxycodone | 3-6 |
|
|
| Renally excreted, primarily as metabolites |
|
| Noroxymorphone |
|
|
|
|
| Oxymorphone |
| Hepatic, renal | Active metabolite; renally excreted as oxymorphone-glucuronide |
|
| Noroxycodone |
|
|
|
Propoxyphene 1.5% | 4-6 |
|
|
| May depress cardiac conduction secondary to anesthetic properties; half-life not altered in renal failure |
|
| Norproproxyphene (25) | 22.9-36.6 | Renal | Local anesthetic properties; cardiac conduction abnormalities can result with accumulation (not reversed by naloxone); not hemodialyzable |
Buprenorphine | 6-8 |
|
|
| One source says it is almost completely metabolized in the liver; another says the majority is excreted unchanged in feces Undergoes enterohepatic circulation with metabolites |
|
| Glucuronidation products |
| Renal |
|
|
| Norbuprenorphine |
| Renal | May have weak analgesic properties. |
|
|
|
|
| Primary route of elimination is renal; hepatic, biliary, and fecal routes also involved |
Butorphanol 5% | 3-4 |
|
|
| Extensively liver metabolized; ClCr <30 mL/min half-life increased from 5.75-10.5 hr in single-dose, intranasal administration |
|
| Norbutorphanol |
| Biliary | No analgesic activity |
|
| Hydroxybutorphanol |
| Renal | Analgesic activity; major metabolite; 60%-80% renally excreted |
Nalbuphine | 3-6 |
|
|
| Hepatic metabolism; metabolites and parent compound excreted in urine and feces |
7% |
|
|
|
| Major route of elimination is biliary secretion |
Pentazocine 4.9% | 3-6 |
|
|
| (l) isomer responsible for analgesic activity; large interpatient variability in metabolism and oral bioavailability |
|
|
|
|
| Bioavailability in cirrhotic patients increased to 60-70% |
|
| Alcoholic and carboxylic acid metabolites |
| Renal | Inactive metabolites |
|
| Pentazocine glucuronide |
| Renal | Inactive metabolite |
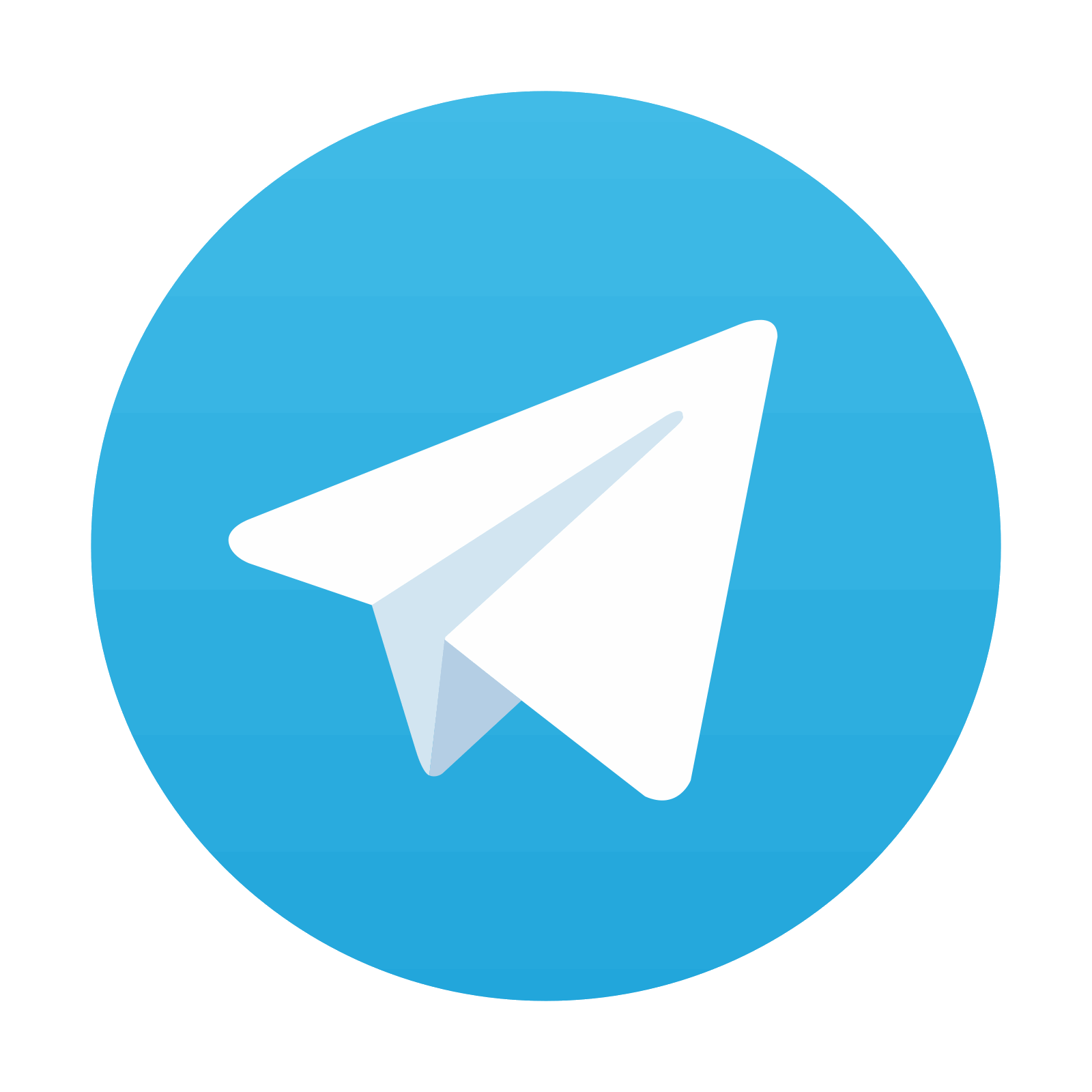
Stay updated, free articles. Join our Telegram channel
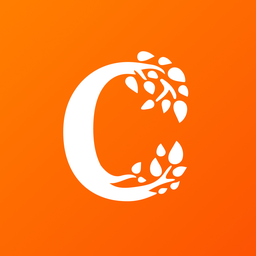
Full access? Get Clinical Tree
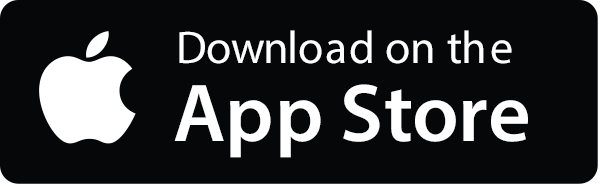
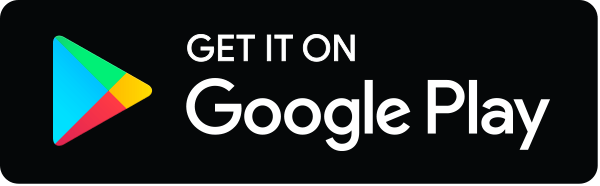
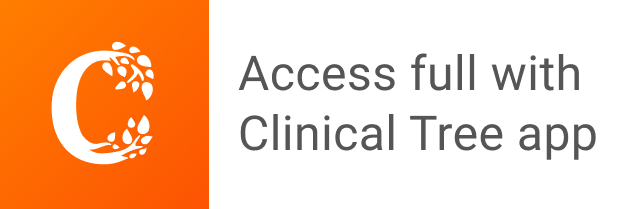