KEY POINTS
Mechanical ventilators are support devices, not therapeutic devices. The clinical goal is thus to support gas exchange without causing harm.
A number of challenges face clinicians in providing safe and effective mechanical ventilatory support. Two of the most important are (1) supporting gas exchange without causing injury from applied pressure or FiO2; (2) providing comfortable interactive support as the lung recovers.
Innovations need to focus on addressing clinical challenges. Moreover, to be accepted as “standard of care,” an innovation must be shown to improve an important clinical outcome.
Recent innovations focusing on supporting gas exchange in a “lung protective” fashion include airway pressure release ventilation, high frequency ventilation, and adaptive support ventilation.
Recent innovations focusing on improving patient-ventilator synchrony include various feedback controls on variable flow-pressure-targeted breaths, proportional assist ventilation, and neutrally adjusted ventilatory assistance.
While all of these innovations have conceptual appeal and supporting observational data, none as yet have convincing randomized control trial data demonstrating improved clinical outcomes.
INTRODUCTION
The overarching goal of positive pressure mechanical ventilation is to provide adequate gas exchange support while not causing harm. Indeed, positive pressure mechanical ventilators are only support technologies, not therapeutic technologies. As such they cannot be expected to “cure” disease; they can only “buy time” for other therapies (including the patient’s own defenses) to work.
Conventional approaches to positive pressure ventilation involve applying ventilatory patterns mimicking normal through either masks or artificial airways. This is usually done with modes of support incorporating assist/control breath triggering mechanisms, gas delivery patterns governed by either a set flow or pressure, and breath cycling based on either a set volume, a set inspiratory time or a set flow. Often this support includes positive end expiratory pressure (PEEP) and supplemental oxygen. In recent decades a number of novel or unconventional approaches to providing mechanical ventilatory support have been introduced. For these to be considered of value, however, it would seem reasonable that they address important clinical challenges and be shown to improve important clinical outcomes (eg, mortality, duration of ventilation, sedation needs, complications). The remainder of this chapter will focus on challenges facing clinicians in providing mechanical ventilatory support and assess several novel approaches introduced over the last two decades in the context of these challenges.
CLINICAL CHALLENGES FACING CLINICIANS PROVIDING MECHANICAL VENTILATORY SUPPORT
Probably the most important challenge facing clinicians providing mechanical ventilatory support today is managing the balance between providing adequate gas exchange and avoiding lung injury associated with positive airway pressure and oxygen exposure. On the one hand, patients in respiratory failure need adequate tissue oxygenation and acid/base balance; on the other hand, the lungs are fragile structures easily injured by excessive stretch, alveolar collapse-reopening and high oxygen exposure. This challenge is made more difficult by the fact that lung injury is usually heterogeneous and thus what may benefit gas exchange in one region (eg, higher pressure) may cause worse injury in another.1
Lung injury from mechanical ventilatory support is often termed ventilator associated lung injury, or more commonly, ventilator-induced lung injury (VILI).2-7 Pathologically, VILI resembles in many ways the inflammatory response seen in other forms of acute lung injury and the acute respiratory distress syndrome (ALI/ARDS).2,3 The principal cause of VILI is alveolar injury induced by alveolar overstretch at end inspiration (overdistension), extended periods of tidal breath delivery above normal physiologic values, and cyclic atelectasis-recruitment that occur during positive pressure ventilation (Fig. 50-1).2-7 In general, the risk for VILI increases as end inspiratory transpulmonary pressures exceed 30 to 35 cm H2O, as tidal volumes exceed 8 to 10 mL/kg (ideal body weight), and as regions of repetitive alveolar opening-closing develop.2-12 Other ventilatory pattern factors may also be involved in the development of VILI. These include frequency of stretch13 and the acceleration/velocity of stretch.14
FIGURE 50-1
Ventilator-induced lung injury during positive pressure ventilation comes from several factors. Depicted is the sigmoidal-shaped pressure-volume relationship seen in the acutely injured lung. Injury can occur from end inspiratory overdistention (upper right region), repetitive excessive tidal breath delivery (middle region), and repetitive collapse-reopening of alveolar units (lower left region).
Importantly, VILI is associated with cytokine release4-6 and bacterial translocation.15 These are often implicated as important contributors to the systemic inflammatory response with multiorgan dysfunction that results in VILI associated mortality. The incidence of VILI has been reported to be as high as 24% of patients who are receiving mechanical ventilation for reasons other than ALI/ARDS although estimates widely vary.5,7,16
Another conceptual source of injury during mechanical ventilatory support is oxygen toxicity. Oxygen concentrations approaching 100% are known to cause oxidant injuries in airways and lung parenchyma.17 A “safe” oxygen concentration or duration of exposure is not clear in sick humans, however, since most of the data supporting the concept of oxygen toxicity comes from animals. Most consensus groups have argued that FiO2 values less than 0.4 are safe for prolonged periods of time and that FiO2 values of greater than 0.80 should be avoided if at all possible.18
A second major challenge facing clinicians, providing mechanical ventilatory, is to ensure that the duration of mechanical ventilation is kept to a minimum. The shorter the duration of mechanical support, the less is the risk for VILI, infections, airway injury, delirium, and respiratory muscle atrophy.19-23 This challenge involves both vigilance in assessing the need for continued support every day as well as in providing comfortable support that promotes normal muscle function and minimizes the need for sedation.19
The available evidence strongly supports the routine (daily) assessment of the need for continued ventilatory support through the use of spontaneous breathing trials (SBTs) in patients recovering from acute respiratory failure.19 In those patients deemed to still require continued support after the SBT assessment, the available evidence would further suggest that this support be provided as patient triggered interactive support aimed at promoting comfortable respiratory muscle activity that avoids both fatigue and disuse atrophy.19-23
Comfortable interactive support requires clinician optimizing all three phases of breath delivery: breath triggering, flow delivery, and cycling. In general, patient ventilator synchrony is best assessed by clinical observations and by analyzing the airway pressure graphic over time. Clinical signs of dyssynchrony are tachypnea, dyspnea, diaphoresis, and tachycardia and the patient is often described as “fighting” the ventilator. Graphically, trigger dyssynchrony is a manifestation of excessive negative airway pressure signals preceding breath triggering or absence of any flow delivery in response to observed effort. Flow dyssynchrony is manifest by the airway pressure graphic during flow delivery being pulled (or “sucked”) downward during inspiration (Fig. 50-2; left panel). Cycle dyssynchrony is manifest by continued patient effort and sometimes double triggering if the cycle is too early. Cycle dyssynchrony can also manifest as rises in airway pressure from expiratory muscle activity if the cycle is too long.
FIGURE 50-2
Graphical depictions of flow synchrony and dyssynchrony. Plotted are flow (upper panel), volume (middle panel), and pressure (lower panel). In the left example, set flow is inadequate for patient demand and the airway pressure graphic is literally “sucked” downward by the flow-starved patient (solid arrow). In the right example, a pressure-targeted, variable flow breath is provided delivering the same tidal volume. Because the flow adjusts to demand (broken arrow), synchrony is improved and the airway pressure graphic retains its upright shape.
Conventional strategies to optimize synchrony during breath triggering, flow delivery and cycling include a number of options. Optimal breath triggering involves assisted breath trigger sensitivity be as sensitive and responsive as possible without autocycling.24 In patients with flow limited airways and resulting intrinsic PEEP, judicious amounts of applied PEEP can reduce the imposed trigger load (PEEPi).25 Optimizing flow synchrony when using set flow modes (eg, volume assist control or volume-targeted SIMV) involves careful selection of flow magnitude and pattern. Indeed, flow synchrony is often easier to achieve with pressure-targeted modes (eg, pressure assist control, pressure-targeted SIMV, or pressure support) because of the adjustable flow features of these modes (Fig. 50-2; right panel).26,27 Finally, cycle synchrony requires proper setting of the target volume and inspiratory time.
NOVEL STRATEGIES ADDRESSING THE CHALLENGE OF BALANCING GAS EXCHANGE VERSUS VILI
Airway pressure release ventilation (APRV, also known as “Bi-level,” “Bi-phasic,” and “BiPAP” among other trade names) is a time-cycled, pressure-targeted form of ventilatory support.28-31 APRV is actually a variation of pressure-targeted SIMV that allows spontaneous breathing (with or without pressure support) to occur during both the inflation and deflation phases. APRV differs from conventional pressure-targeted SIMV in the inspiratory:expiratory (I:E) timing. Specifically, conventional pressure-targeted SIMV uses a “physiologic” inspiratory time with I:E ratio less than 1:1. Spontaneous breaths thus occur during the expiratory phase. In contrast, APRV uses a prolonged inspiratory time producing so called inverse ratio ventilation (IRV with I:E ratios of up to 4 or 5:1). Spontaneous breaths thus now occur during this prolonged inflation period.
The putative advantages of this approach are similar to those of other long inspiratory time (IRV) strategies.28-33 Specifically, the long inflation phase recruits the more slowly filling alveoli and raises mean airway pressure without increasing tidal volume or applied PEEP (although intrinsic PEEP can develop with short expiratory or deflation periods). Unlike older IRV strategies that required paralysis, however, the additional spontaneous efforts during lung inflation may enhance both recruitment and cardiac filling as compared to other controlled forms of support.28 Although IRV strategies are usually reserved for very severe forms of respiratory failure in which airway pressures and FiO2 levels are approaching potentially injurious levels, the recruitment potential associated with APRV may prompt consideration of its use in less severe forms of lung injury.
APRV is generally set up to provide tidal breaths (inflations) of 6 to 8 mL/kg (ideal body weight) and set breathing rates to control PCO2 and pH. The expiratory (deflation) time setting is controversial. Although the IRV pattern requires a short expiratory time, whether consequent intrinsic PEEP is desirable (and if so, how much) is often debated.31
Good gas exchange, often with lower maximal airway pressures than control ventilation, has been demonstrated with APRV in several small observational clinical trials.28-31,34 However, the end inspiratory lung distention in APRV may not be necessarily less than that provided during other forms of support (and, indeed, it could be substantially higher) since spontaneous tidal volumes added to lung volume occur while the lung is inflated with the APRV set pressure.
Several randomized controlled trials have been done with APRV. The first of these appeared to show an outcome benefit to APRV but is difficult to interpret as the control strategy required three days of paralysis and it seemed to markedly worsen gas exchange.29 A later trial compared APRV to a more conventional SIMV strategy and showed no difference in outcome.30 The most recent trial compared APRV to ARDS Network low tidal volume ventilation in 64 patients with trauma induced ALI ARDS.34 In this study, there were no significant differences in any of the clinical outcomes. Specifically ventilator days, ICU length of stay, and mortality were all comparable regardless of mode. Finally, an interesting reanalysis of the data base of a very large ventilator usage survey was recently published.35 In this data base, 234 subjects were identified who were receiving APRV. A case control group of matched patients based on a propensity score and who were receiving assist-control ventilation were also identified. Comparing the APRV group with this matched assist control group found no differences in mortality, ventilator free days, or length of stay. Taken together these studies would suggest that APRV, while a physiologically interesting mode, has not been shown as yet to improve meaningful clinical outcomes in patients with severe ARDS.
High-frequency oscillatory ventilation (HFOV) uses very high breathing frequencies (120-900 breaths per minute [bpm] in the adult) coupled with very small tidal volumes (usually less than anatomic dead space and often <1 mL/kg at the alveolar level) to provide gas exchange in the lungs.36,37 Gas transport up and down the tracheobronchial tree under these seemingly unphysiologic conditions involves such mechanisms as Taylor dispersion, coaxial flows and augmented diffusion.37 The actual device to deliver HFOV in adults uses a to-and-fro piston mechanism to literally vibrate a fresh bias flow of gas delivered at or near the tip of the endotracheal tube. Indeed, because HFOV supplies substantial mean airway pressures but applies very little pressure or volume fluctuations in the alveolus, it is sometimes termed “CPAP with a wiggle.”
The putative advantages to HFOV are twofold. First, the very small alveolar tidal pressure swings minimize cyclical overdistension and derecruitment.36 Second, a high mean airway pressure can also prevent derecruitment. Interestingly, mean pressures used during HFOV are often reported to exceed the 30 to 35 cm H2O threshold employed during conventional ventilation.38-40 The reason this is possible may be explained by alveolar membrane expansion, which could occur when a slowly applied constant pressure is applied rather than the cyclical brief tidal pressures of conventional ventilation.41
In the adult, common initial settings are a frequency of 300 bpm and a mean pressure of 5 cm H2O above the previous conventional ventilation settings.39,40 Oxygenation is largely controlled by the mean pressure setting and the FiO2. CO2 clearance is largely controlled by the “power setting” which controls the oscillatory pressure amplitude. Ironically, lower frequencies favor enhanced CO2 clearance, largely because slower frequencies allow larger volume changes with the applied oscillatory power setting.
Clinical experience with various high-frequency ventilation techniques has been most extensive in the neonatal and pediatric populations.42,43 From these studies, a general consensus has arisen that high-frequency ventilation appears to improve long-term clinical outcomes in these patients.
Adult experience is less with high-frequency techniques as only recently have HFOV devices been available to adequately support gas exchange in this setting. In 2010, the McMaster University Evidence Based Medicine Group updated a meta-analysis of HFOV in ARDS.40 They analyzed eight clinical trials of HFOV in patients with ARDS. This population included some pediatric patients who met the criteria for ARDS. In this analysis, six of the eight studies applied HFOV within 48 hours of intubation and in five of the eight studies the ARDS Network low tidal volume strategy was used as the control group. Four hundred nineteen patients were included in these studies. The resulting meta-analysis showed that HFOV produced a significant reduction in mortality with a risk ratio of 0.77 and a 95% confidence interval range from 0.61 to 0.98. This certainly suggests that there may be a role for HFOV in severe respiratory failure from ARDS. While these results were encouraging, both Canadian and British trials were recently completed and reported. These trials had randomized patients with ARDS to either HFOV or a standard approach. Neither trial showed a benefit with HFOV and one was terminated early because of harm related to HFOV (see Chap. 52).
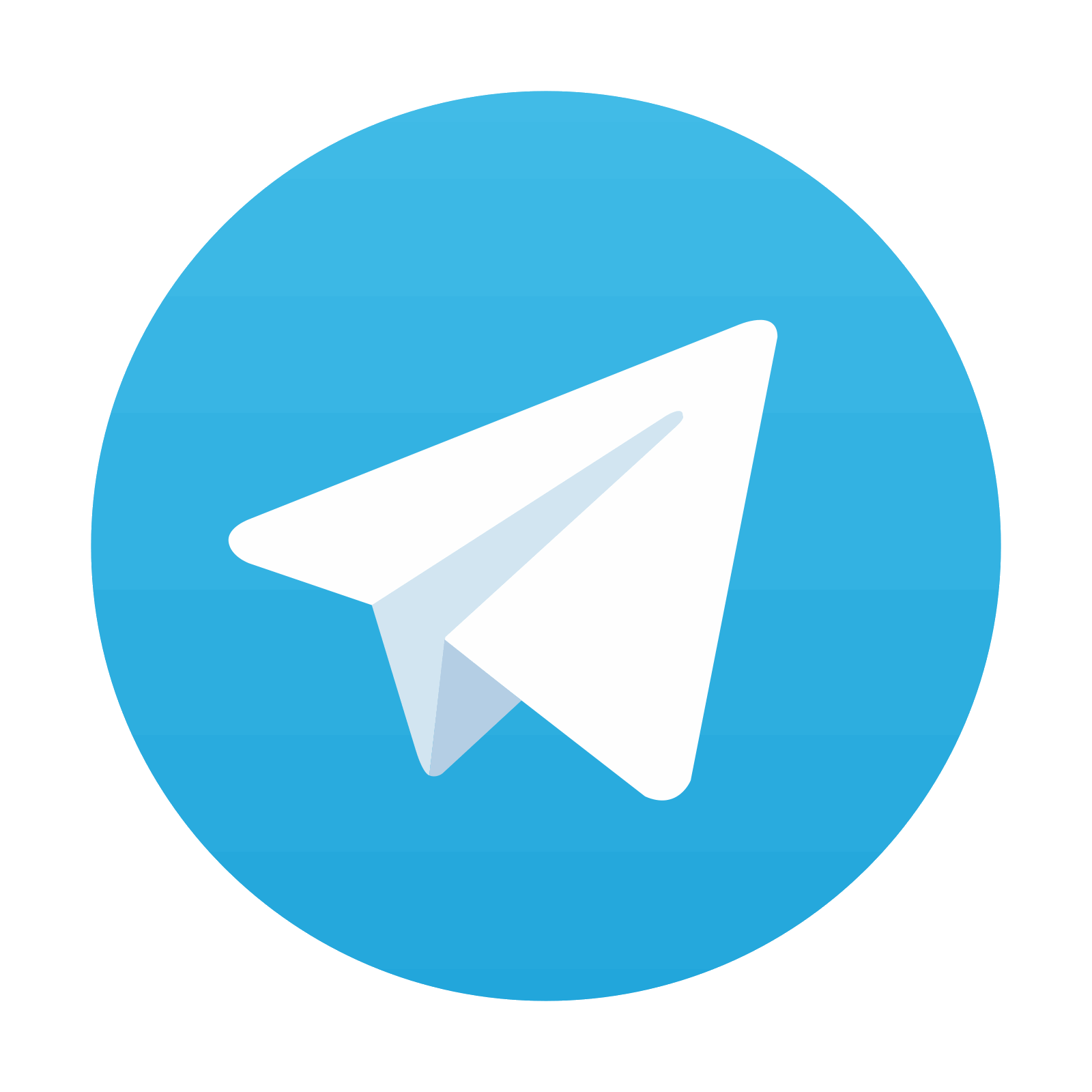
Stay updated, free articles. Join our Telegram channel
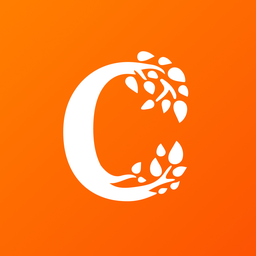
Full access? Get Clinical Tree
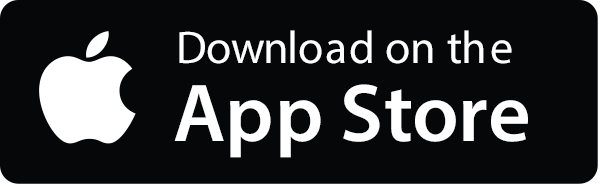
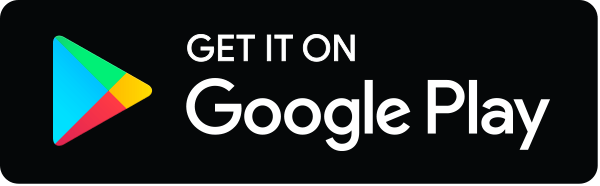