Nonthyroidal Illness Syndrome (Sick Euthyroid Syndrome) in the Intensive Care Unit
Shirin Haddady
Alan P. Farwell
Introduction
Critical illness causes multiple alterations in thyroid hormone concentrations in patients who have no previously diagnosed intrinsic thyroid disease [1,2,3,4,5,6]. These effects are nonspecific and relate to the severity of the illness. Despite abnormalities in serum thyroid hormone parameters, there is little evidence that these patients have clinically significant thyroid dysfunction. Because a wide variety of illnesses tend to result in the same changes in serum thyroid hormones, such alterations in thyroid hormone indexes have been termed the sick euthyroid syndrome or, more recently, the nonthyroidal illness syndrome. These changes are rarely isolated and often are associated with alterations in other endocrine systems, such as reductions in serum gonadotropin and sex hormone concentrations [7] and increases in serum corticotropin and cortisol levels [8]. Similar changes in endocrine function have been shown experimentally by the administration of cytokines from the interleukin (IL) and interferon families as well as tumor necrosis factor-α (TNF-α) [9]. Thus, the sick euthyroid syndrome should not be viewed as an isolated pathologic event but as part of a coordinated systemic reaction to illness that involves both the immune and endocrine systems.
The differentiation between patients with the sick euthyroid syndrome and those with intrinsic thyroid disease is a frequent diagnostic problem in the intensive care unit (ICU). This chapter will first review normal thyroid physiology and discuss the changes in thyroid hormone metabolism seen with critical illness. Management of these patients and the identification of those with intrinsic thyroid disease will then be discussed. Finally, the use of thyroid hormone replacement in the sick euthyroid syndrome will be reviewed.
Normal Thyroid Hormone Economy
Regulation
The synthesis and secretion of thyroid hormone is under the control of the anterior pituitary hormone, thyrotropin (TSH). In a classic negative feedback system, TSH secretion increases when serum thyroid hormone levels fall and decreases when they rise (Fig. 107.1). TSH secretion is also under the regulation of the hypothalamic hormone, thyrotropin-releasing hormone (TRH). The negative feedback of thyroid hormone is targeted mainly at the pituitary level but likely affects TRH release from the hypothalamus as well. In addition, input from higher cortical centers can affect hypothalamic TRH secretion.
Under the influence of TSH, the thyroid gland synthesizes and releases thyroid hormone. Thyroxine (T4, 65% iodine by weight) is the principal secretory product of the thyroid gland, comprising ∼ 90% of secreted thyroid hormone under normal conditions [10]. While T4 may have direct actions in some tissues, T4 primarily functions as a hormone precursor that is metabolized in peripheral tissues to the transcriptionally active 3,5,3′-triiodothyronine (T3, 59% iodine by weight).
Metabolic Pathways
The major pathway of metabolism of T4 is by sequential monodeiodination [11] (Fig. 107.2). At least three deiodinases, each with its unique expression in different organs, catalyze the deiodination reactions involved in the metabolism of T4. Removal of the 5′-, or outer ring, iodine by type 1 iodothyronine 5′deiodinase (type 1 deiodinase, D1) is the “activating” metabolic pathway, leading to the formation of T3. Removal of the inner ring, or 5-, iodine by type 3 iodothyronine deiodinase (type 3 deiodinase, D3) is the “inactivating” pathway, producing the metabolically inactive hormone, 3,3′,5′-triiodothyronine (reverse T3, rT3). Type 1 deiodinase (D1) is found most abundantly in the liver, kidneys, and thyroid. It is upregulated in hyperthyroidism and downregulated in hypothyroidism. Type 3 deiodinase is expressed primarily in the brain, in skin, and in placental and chorionic membranes. The actions of D3 also include the inactivation of T3 to form T2, another inactive metabolite. Under normal conditions, ∼ 41% of T4 is converted to T3, ∼ 38% is converted to rT3, and ∼ 21% is metabolized via other pathways, such as conjugation in the liver and excretion in the bile [4,5].
T3 is the metabolically active thyroid hormone and exerts its actions via binding to chromatin-bound nuclear receptors and regulating gene transcription in responsive tissues [12]. Important in the understanding of the alterations in circulating thyroid hormone levels seen in critical illness is the fact that only ∼ 10% of circulating T3 is secreted directly by the thyroid gland while > 80% of T3 is derived from conversion of T4 in peripheral tissues [10,11]. Thus, factors that affect peripheral T4 to T3 conversion will have significant effects on circulating T3 levels. Serum levels of T3 are approximately 100-fold less than those of T4, and, like T4, T3 is metabolized by deiodination to form diiodothyronine (T2) and by conjugation in the liver. The half-lives of circulating T4 and T3 are 5 to 8 days and 1.3 to 3 days, respectively [13].
Serum-Binding Proteins
Both T4 and T3 circulate in the serum as bound hormones to several proteins synthesized by the liver [14]. thyroxine-binding globulin (TBG) is the predominant transport protein and binds
∼ 80% of the circulating serum thyroid hormones. The affinity of T4 for TBG is approximately 10-fold greater than that of T3 and is part of the reason that circulating T4 levels are higher than T3 levels. Other serum-binding proteins include transthyretin [15], which binds ∼ 15% of T4 but little, if any T3, and albumin, which has a low affinity but a very large binding capacity for T4 and T3. Overall, 99.97% of circulating T4 and 99.7% of circulating T3 is bound to plasma proteins.
∼ 80% of the circulating serum thyroid hormones. The affinity of T4 for TBG is approximately 10-fold greater than that of T3 and is part of the reason that circulating T4 levels are higher than T3 levels. Other serum-binding proteins include transthyretin [15], which binds ∼ 15% of T4 but little, if any T3, and albumin, which has a low affinity but a very large binding capacity for T4 and T3. Overall, 99.97% of circulating T4 and 99.7% of circulating T3 is bound to plasma proteins.
Free Hormone Concept
Essential to the understanding of the regulation of thyroid function and the alterations of circulating thyroid hormones seen in critical illness is the “free hormone” concept, which is that only the unbound hormone has any metabolic activity. Under the regulation by the pituitary, overall thyroid function is affected when there are any changes in free hormone concentrations. Changes in either the concentrations of binding proteins or the binding affinity of thyroid hormone to the serum-binding proteins have significant effects on the total serum hormone levels due to the high degree of binding of T4 and T3 to these proteins. Despite these changes, this does not necessarily translate into thyroid dysfunction.
Thyroid Hormone Economy in Critical Illness
The widespread changes in thyroid hormone economy in the critically ill patient occur as a result of: (a) alterations in the peripheral metabolism of the thyroid hormones, (b) alterations in TSH regulation, and (c) alterations in the binding of thyroid hormone to TBG.
Peripheral Metabolic Pathways
One of the initial alterations in thyroid hormone metabolism in acute illness is the acute inhibition of type 1 deiodinase, resulting in the impairment of T4 to T3 conversion in peripheral tissues [16]. D1 is inhibited by a wide variety of factors, including acute illness (Table 107.1) [11], resulting in the acute decrease in T3 production in critically ill patients. In contrast, inner ring deiodination by D3 may be increased by acute illness, resulting in increased levels of rT3 [17,18]. Additionally, since rT3 is subsequently deiodinated by D1, degradation of rT3 decreases and levels of this inactive hormone rise in proportion to the fall in T3 levels. Recent studies on postmortem tissues have confirmed that alterations in deiodinase enzymes occur in patients who died during an acute critical illness, with decrease in type 1 and increase in type 3 deiodinases and, surprisingly, an increase in skeletal muscle type 2 deiodinase [18,19]. Finally, there is impaired transport of T4 into peripheral tissues, such as the liver and kidney where much of the circulating T3 is produced, further contributing to the decrease in production of T3 [20].
Table 107.1 Factors That Inhibit Type 1 5″-Deiodinase Activity | |
---|---|
|
TSH Regulation
Serum TSH levels are usually normal early in acute illness. However, TSH levels often fall as the illness progresses due to the effects of a variety of inhibitory factors that are common in the treatment of the critically ill patient (Table 107.2). Many medications used in the treatment of the critically ill patient may also have inhibitory effects on serum TSH levels. Van den Berghe et al. [21] reported that intravenous administration of dopamine for as short a time as 15 to 21 hours is able to acutely decrease TSH levels and its withdrawal results in a tenfold increase in serum TSH levels. In one study, children who received dopamine infusions during a pediatric ICU admission for meningococcal sepsis had lower TSH levels than those who did not [22,23]. Increased levels of glucocorticoids, whether from endogenous or exogenous sources, also have direct inhibitory effects on TSH secretion.
TSH secretion also occurs as a result of altered TRH secretion. Decreased TRH secretion due to inhibitory signals from higher cortical centers, impaired TRH metabolism [24], the alteration of pulsatile TSH [25], and the decrease or absence of a nocturnal TSH surge [25,26] may all decrease TSH levels. Serum levels of Leptin, the ob gene product that has been shown to vary directly with thyroid hormone levels [27], also fall as illness progresses [28] and hypothalamic TRH secretion falls, which in turn leads to lowered TSH levels [29]. The decrease of hypothalamic TRH gene expression in animal models is, however, not associated with increased serum T4 and T3
levels [30]. Finally, certain thyroid hormone metabolites that are increased during acute nonthyroidal illness may play a role in the inhibition of TSH and TRH secretion [1].
levels [30]. Finally, certain thyroid hormone metabolites that are increased during acute nonthyroidal illness may play a role in the inhibition of TSH and TRH secretion [1].
Table 107.2 Factors That Alter TSH Secretion | ||||
---|---|---|---|---|
|
Table 107.3 Factors That Alter Binding of T4 to TBG | ||||||
---|---|---|---|---|---|---|
|
Serum-Binding Proteins
The affinity of thyroid hormones binding to transport proteins and the concentrations of serum-binding proteins are altered with acute illness (Table 107.3). Serum levels of transthyretin and albumin decrease, especially during prolonged illness, malnutrition, and in high catabolic states. TBG levels may be increased, as seen with liver dysfunction and HIV infection, or decreased, as seen with severe or prolonged illness [14]. TBG may also be rapidly degraded by protease cleavage during cardiac bypass, thereby partially explaining the rapid fall of serum T3 levels in patients undergoing cardiac surgery [31].
An acquired binding defect of T4 to TBG is commonly seen in patients with critical illness. This is believed to result from the release of some as yet unidentified factor from injured tissues that has the characteristics of unsaturated nonesterified fatty acids (NEFA) [32], which also inhibit T4 to T3 conversion [33]. In systemically ill patients, NEFA levels rise in parallel with the severity of the illness [34], and drugs such as heparin stimulate the generation of NEFA [35]. Many drugs, including high-dose furosemide, antiseizure medications, and salicylates also alter binding of T4 to TBG. The alterations in serum-binding proteins in critical illness make the estimation of the free hormone concentrations difficult (see later).
Role of Cytokines in the Pathogenesis of the Sick Euthyroid Syndrome
Cytokines are medium-sized polypeptide hormones secreted by mononuclear cells of the lymphoid system in response to a variety of stimuli, including infection by foreign organisms, invasion by foreign cells, metabolic derangements, and organ system dysfunction [36]. Cytokines have an array of systemic and local actions characteristic of illness, such as fever, prostration, inflammation, and the initiation of wound repair. Cytokine production by lymphoid cells is essential for the development and maintenance of immunity. The actions of cytokines include both autocrine and paracrine effects on cell proliferation and differentiation and on induction of other cytokines. Classes of cytokines include the interleukins (1 to 12), interferons (α, β, and γ), tumor necrosis factors (α and β), and other assorted growth factors. Of these cytokines, TNF-α and several interleukins (IL-1, 6, and 10) have been extensively studied for their role in the pathogenesis of the sick euthyroid syndrome [9,37].
TNF-α, IL-1, and IL-6 concentrations are increased in systemic illness and are implicated as mediators of endotoxemia-induced shock, fever, and metabolic acidosis. TNF-α and IL-1 both induce the production of IL-6 and TNF-α also induces IL-1 production [38] and activates nuclear factor-kappa B (NF-κ B), which has been shown to inhibit hepatic type 1 5′-deiodinase activity [39]. Serum concentrations of these cytokines have shown to be inversely proportional to serum T3 concentrations in children (IL-6 and TNF-α [40]); postoperative patients (IL-6 [41]); hospitalized patients, including those with acute myocardial infarction (IL-6 [42,43,44]) and after bone marrow transplant (IL-6 and TNF-α [45]); and nursing home patients (TNF-α [46]). However, there have been reports of a lack of correlation between IL-6 and TNF-α after abdominal surgery [47] and in rheumatoid arthritis [48].
The administration of cytokines to animals produces an acute fall in serum T3 concentrations in rats (TNF-α [49], IL-1 [50,51], IL-6 [52]) and mice (IL-1 [53,54], IL-6 [55]) and shows a direct inhibitory effect on thyroid cells in culture (TNF-α [49,56,57] and IL-1 [57,58,59]). Variable effects on deiodinase enzymes have been reported, including an inhibition of type 1 5′-deiodinase (IL-1β [60], NF-κ B [39]) and inhibition (TNF-α, IL-1, and IL-6 [61]) and induction of type 2 5′-deiodinase (response to lipopolysaccharide [62]).
The administration of TNF-α [63] or IL-6 [64] to healthy human volunteers as well as isolated limb perfusion of cancer patients with TNF-α [65] also produced an acute decrease in both serum T3 and TSH concentrations and a rise in serum rT3 concentrations, while the administration of an IL-1α receptor antagonist failed to alter the decrease in serum thyroid hormone concentrations observed after infusion of endotoxin in healthy males [66]. In addition, recovery from these changes in thyroid hormone parameters was associated with a rise in TSH [65], similar to the recovery phase of the sick euthyroid syndrome (see later).
Other cytokines have been variably investigated as to their role in the pathogenesis of the sick euthyroid syndrome. Interferon α causes a decrease in serum T3 and TSH and a rise in serum rT3 concentrations in both humans [67] and mice [58] and has been shown to directly inhibit thyroidal type 1 5′-deiodinase activity [56,57] while interferon γ appears to have no effect on thyroid hormone parameters [68]. An increase in TSH has been observed in patients treated with IL-2 [69]. Soluble cytokine receptors may play a regulatory role in the cytokine cascade by functioning either as carrier proteins or cytokine inhibitors [36,38], and serum T3 levels were found to be inversely proportional to soluble receptors for TNF-α, IL-1, and IL-2 in hospitalized patients [58].
From the data discussed earlier, it is likely that cytokines play a role in the alterations in thyroid hormone metabolism that occurs with systemic illness. While all cytokines examined to date can produce the sick euthyroid syndrome in either man or rodents when administered in pharmacologic doses, no one cytokine can be singled out as the primary mediator of the syndrome. This is not unexpected, given the diverse interrelationships and cascade nature of the cytokine network. Whether the sick euthyroid syndrome results from activation of the cytokine network or simply represents an endocrine response to systemic illness resulting from the same mediators that trigger the cytokine cascade remains to be determined.
Stages of the Sick Euthyroid Syndrome
As discussed earlier, critical illness causes multiple nonspecific alterations in thyroid hormone concentrations in patients without intrinsic thyroid dysfunction that relate to the severity of the illness [3,70,71]. One author has postulated that sick euthyroid syndrome may be a compensatory mechanism in response to the oxidative stress of acute illness [72]. Whatever the underlying cause, these alterations in thyroid hormone parameters represent a continuum of changes that depends on the severity of the illness and that can be categorized into several distinct stages (Fig. 107.3) [1]. The wide spectrum of changes observed often results from the differing points in the course of the illness that the thyroid function tests were obtained. Importantly, these changes are rarely isolated and often associated with alterations of other endocrine systems, such as decreases in serum gonadotropin and sex hormone concentrations [73] and increases in serum adrenocorticotropic hormone and cortisol levels [74]. Thus, the sick euthyroid syndrome should not be viewed as an isolated pathologic event but as part of a coordinated systemic reaction to illness involving both the immune and endocrine systems.
Low T3 State
Common to all of the abnormalities in thyroid hormone concentrations seen in critically ill patients is a substantial depression of serum T3 levels, which can occur as early as 24 hours after the onset of illness. Over half of the patients admitted to the medical service will demonstrate depressed serum T3 concentrations [75,76]. The development of the low T3 state arises from the impairment of peripheral T4 to T3 conversion through the inhibition of type 1 deiodinase (discussed earlier). This results in the marked reduction of T3 production and rT3 degradation [77], thereby leading to the reciprocal changes in serum T3 and serum rT3 concentrations. Low T3 levels are also found in the peripheral tissues [18]. Previously, it was thought that the inhibition of type 1 deiodinase was the sole cause of the low T3 syndrome by decreasing T3 production. Recent studies suggest that increased type 3 deiodinase in critical illness increases T3 disposal, adding to the decrease in serum T3 levels [17,18]. Thyroid hormone receptor expression is also decreased in acute nonthyroidal illness [78], possibly in response to the decrease in tissue T3 levels.
High T4 State
Serum T4 levels may be elevated early in acute illness due to either the acute inhibition of type 1 deiodinase or increased TBG levels. This is seen most often in the elderly and in patients with psychiatric disorders. As the duration of illness increases, nondeiodinative pathways of T4 degradation increase serum T4 levels to the normal range [75].
Low T4 State
As the severity and the duration of the illness increases, serum total T4 levels decrease into the subnormal range. Contributors to this decrease in serum T4 levels are (a) a decrease in the binding of T4 to serum carrier proteins, (b) a decrease in serum TSH levels leading to decreased thyroidal production of T4, and (c) an increase in nondeiodinative pathways of T4 metabolism. The decline in serum T4 levels correlates with prognosis in the ICU, with mortality increasing as serum T4 levels drop below 4 μg per dL and approaching 80% in patients with serum T4 levels below 2 μg per dL [79,80,81]. Despite marked decreases in serum total T4 and T3 levels in the critically ill patient, the free hormone levels have been reported to be normal or even elevated [82,83], providing a possible explanation for why most patients appear euthyroid despite thyroid hormone levels in the hypothyroid range. Thus, the low T4 state is unlikely to be a result of a hormone-deficient state and is probably more of a marker of multisystem failure in these critically ill patients.
Recovery State
As acute illness resolves, so do the alterations in thyroid hormone concentrations. This stage may be prolonged and is characterized by modest increases in serum TSH levels [84]. Full recovery, with restoration of thyroid hormone levels to the normal range, may require several weeks [85] or months after hospital discharge [76]. One study reported that 35 of 40 patients with nonthyroidal illness after coronary artery bypass grafting were able to regain normal thyroid function within 6 months after surgery [86].
Alterations in Thyroid Function in Specific Critical Illnesses
Caloric Deprivation
Most, if not all, nonthyroidal illness is associated with decreased caloric intake, catabolism, and/or malnutrition. Caloric deprivation is the most common inhibitory factor of type 1 5′-deiodinase [11,87,88]. Serum T3 levels decrease and rT3 levels increase within 24 hours of the onset of a fast. The decrease in serum T3 levels may possibly be an adaptive
response in order to preserve the total body protein stores. Indeed, restoring the serum T3 to normal during starvation results in a marked increase in urinary nitrogen excretion [89]. Thus, the inhibition of T4 to T3 conversion in starvation can be viewed as a condition of adaptive hypothyroidism. Further support for the role of caloric deprivation in the development of the sick euthyroid syndrome is the demonstration that serum thyroid hormone levels in critically ill patients receiving nutritional support return to normal levels [90].
response in order to preserve the total body protein stores. Indeed, restoring the serum T3 to normal during starvation results in a marked increase in urinary nitrogen excretion [89]. Thus, the inhibition of T4 to T3 conversion in starvation can be viewed as a condition of adaptive hypothyroidism. Further support for the role of caloric deprivation in the development of the sick euthyroid syndrome is the demonstration that serum thyroid hormone levels in critically ill patients receiving nutritional support return to normal levels [90].
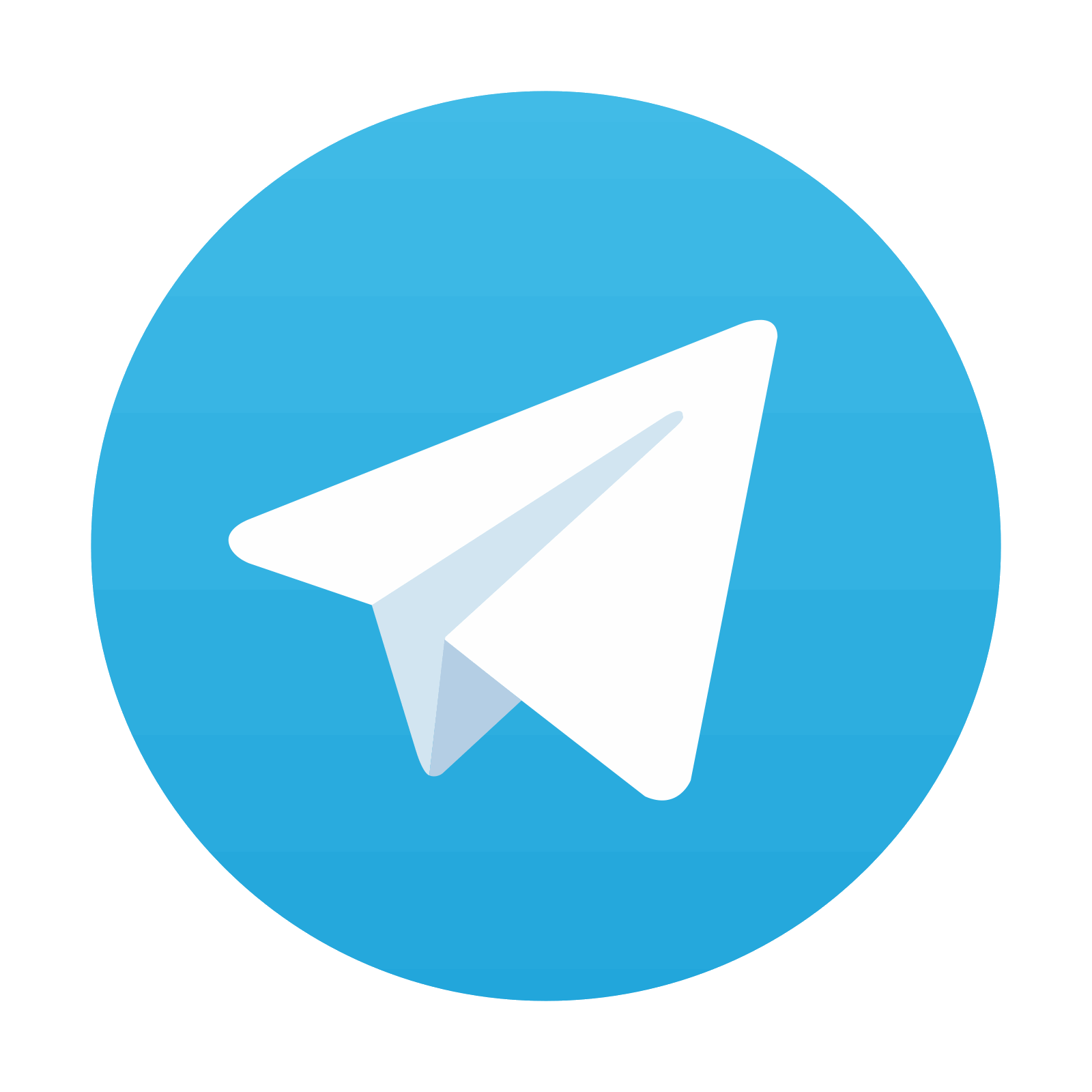
Stay updated, free articles. Join our Telegram channel
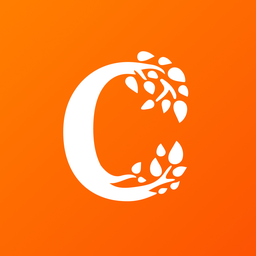
Full access? Get Clinical Tree
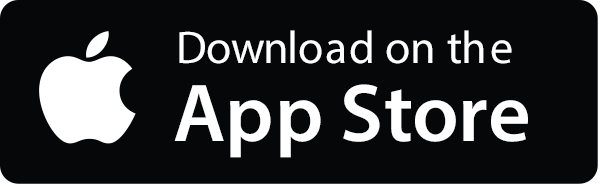
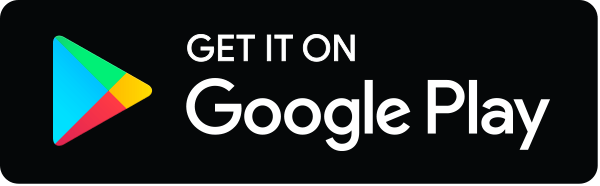