Chapter Outline
Introduction 127
Monitors Connected to the Patient 128
Cardiovascular System 128
Respiratory System 128
Miscellaneous Monitors 136
Neurological System Monitors 142
Monitors Connected to the Anesthesia Machine 144
Oxygen Concentration Analyzers 144
Volatile Agent and Nitrous Oxide Analyzers 145
Respirometer and Pneumotachograph 145
Conclusion/Future Directions in Noninvasive Monitoring 146
Introduction
The hallmark of anesthesiology is providing patients with pain relief, comfort, and safety during unpleasant diagnostic studies, treatments, and surgery. Imparting safety to anesthetized patients requires vigilance, and continuous observation and monitoring of the patient. This forms the basis of the American Society of Anesthesiologists (ASA) seal, which depicts a watchtower and the word “vigilance” ( Figure 9-1 ). Historically, and at present, stress of medical interventions and from anesthetics themselves causes physiological derangements in anesthetized patients, manifesting as erratic and unstable vital signs sensed by monitors. Monitors are the topic of this chapter.

The work of Joseph T. Clover, MD (1825–1882), renowned British anesthetist, was paramount in the development of monitoring for anesthetized patients. In Clover’s time chloroform and nitrous oxide formed the basis of surgical anesthesia. He was a strong advocate of palpating the patient’s pulse during anesthesia, and can be seen doing so in photographs ( Figure 9-2 ) and depictions of him providing anesthesia care.

The American Society of Anesthesiologists (ASA) House of Delegates approved on October 21, 1986, and last amended on October 25, 2005, a set of standards outlining basic anesthesia monitoring (otherwise known as the “ASA Standard Monitors”), to be followed whenever general, regional, or monitored anesthesia care (MAC) anesthesia is provided. These vital monitors and their associated equipment/technology are noninvasive and will be discussed in this chapter.
The ASA Standard Monitors include measures of patient oxygenation, ventilation, circulation, and, if necessary, the ability to monitor body temperature. Why did the ASA choose these patient variables and not others? The reason is because it is the responsibility of the anesthetist to provide adequate oxygenation and respiration to the organs of the patient at the tissue level to prevent cellular damage or cellular death. The ideal monitor would provide information regarding cell oxygenation and respiration safely, noninvasively, accurately, reliably, consistently, and in real time. This monitor should also require little to no maintenance, no calibration and should be monetarily inexpensive. Unfortunately, there is no single monitor in existence that can provide this information to the anesthetist. Therefore, surrogate measures must be obtained: inspired oxygen analysis, end-tidal CO 2 analysis, electrocardiography, blood pressure, and pulse oximetry. It is the task of the anesthetist to synthesize and interpret the data from these indirect monitors in order to infer adequacy of tissue oxygenation and respiration.
Assessment of oxygenation must include an oxygen analyzer to measure the amount of inspired oxygen within a breathing circuit, and a measurement of blood oxygenation (usually via pulse oximetry). Ventilation, in addition to clinical observation, may be detected by measuring carbon dioxide in the patient’s exhaled gas. Capnography, capnometry, or mass or Raman spectrometry are used to quantify expired carbon dioxide. Appropriate monitoring of circulation requires a continuous electrocardiogram and perfusion assessment, in addition to measurement of blood pressure and heart rate at least every 5 minutes. Lastly, temperature, if deemed necessary by the anesthesiologist, can be measured with a thermistor or thermocouple probe. Each monitor is prone to error, so all of the above monitors are best used when the data are pooled together, in conjunction with clinical data from direct patient observation, physical examination, and assessment, such as chest auscultation or direct palpation of pulses, to fully gauge the well-being of the patient under anesthesia.
The anesthetized patient often needs additional noninvasive monitors, such as inhalational agent concentration analyzers, peripheral nerve stimulation for neuromuscular blockade, or central nervous system monitoring. These adjunct monitors will also be discussed.
Monitors Connected to the Patient
Cardiovascular System
Electrocardiogram (ECG)
The ECG monitors the conduction of electrical impulses through the heart. These yield potentials of 0.5 to 2.0 mV at the patient’s skin, to produce PQRST waveforms. This monitor is used to determine the heart rate and to detect and diagnose arrhythmias, myocardial ischemia, structural defects, pacemaker function, and electrolyte abnormalities. Note that the presence of an ECG signal does not guarantee adequate cardiac contraction or output (e.g., pulseless electrical activity).
Mechanism of Monitoring
Electrode pads containing a silver/silver chloride electrode bound to adhesive material and a conductive gel, which decreases the electrical resistance of the skin surface, are applied to the patient’s skin ( Figure 9-3 ). ECG electrodes measure a small electrical signal (about 1 mV), which is amplified and broadcasted over a 0.01- to 250-Hz bandwidth. This allows for the filtration of “noise. ” The ECG is prone to electrical interference from outside sources, and requires proper electrode application to clean, dry skin, ideally free of hair. Severely burned patients or those with certain dermatological conditions may require sterile subcutaneous needle electrodes rather than electrode pads. In normal patients, the injury risk from ECG monitor application is essentially zero. However, ECG skin electrodes can cause skin irritation if left on for a prolonged duration of time without being changed, as is often the case for intensive care unit (ICU) patients ( Table 9-1 ).

|
|
|
|
|
|
ECG skin electrodes, and peripheral nerve electrode pads, should be placed upon skin which is cleaned to remove dead skin, decrease signal impedance and allow for more accurate signal measurement.
Appropriate electrode placement and location is an important component of proper ECG monitoring. To effectively detect arrhythmias and ischemia, the pads must be placed in consistent locations on the patient ( Figure 9-4 ). Limb leads must be placed on, or near, their appropriate limbs and the precordial lead (V 5 ) at the fifth intercostal space, anterior axillary line. Note that lead RL is a “ground” lead, or earth reference. Therefore, placement of RL anywhere on the patient is acceptable ( Figure 9-5 ).


Modes and Options for ECG Monitoring
The ECG monitor has several choices for the filtering of noise, most commonly called “diagnostic” and “monitor” modes. The monitor mode filters out noise by using a narrowed band pass (0.5 to 40 Hz), while the diagnostic mode filters less signal and noise by using a wider band pass (0.05 to 100 Hz).
Rate Detection
The ECG is an excellent measure of heart rate ( Figure 9-6 ). Visual or audible alarms alert the anesthetist to bradycardia and tachycardia.

Rhythm Detection
It is important to monitor and ensure the anesthetized patient maintains a normal sinus rhythm—a P wave before each QRS complex. P waves are best seen in lead II.
Ischemia Detection
This diagnostic mode should be used when monitoring for ischemia. Automatic trending of ST segment changes is often available and useful for monitoring the development of ischemia over time. Continuous monitoring of leads II and V 5 simultaneously allows for detection of ischemia anywhere in a large area of the myocardium. Lead II monitors the inferior portion of the heart, supplied by the right coronary artery. Lead V 5 monitors the bulk of the left ventricle, supplied by the left anterior descending artery. Lead I may be monitored for patients in whom the left circumflex artery is at risk. Visual and/or audible alarms may be configured to provide the anesthetist a warning of possible ischemic changes.
Impedance Pneumography and Apnea Detection
Many ECG monitors have the ability to measure a patient’s respiratory rate through impedance pneumography ( Figure 9-7 ). Impedance pneumography works by measuring the electrical impedance of the thoracic cavity. The baseline thoracic impedance is often represented as “Z.” As air enters the thoracic cavity, lung volume increases, thus increasing the thoracic impedance. During expiration, lung volume decreases, and the impedance of the thoracic cavity goes down. These changes in Z are sometimes called ΔZ. These data are processed and displayed in waveforms representing inspiration and expiration, allowing the anesthetist to observe respiratory rate and depth of breathing. ECG lead I allows for monitoring of the upper chest breaths, while ECG lead II gives data from the lower chest and diaphragmatic area. Impedance pneumography is most commonly used by the anesthetist within the postanesthesia care unit (PACU) or intensive care unit in spontaneously ventilating patients. Cessation in impedance, or when ΔZ becomes equal to Z, will activate an apnea alarm, alerting the anesthetist that the patient may have stopped making respiratory effort.

Implantable Cardiac Rhythm Management Devices
The ECG is capable of monitoring cardiac pacemakers by displaying pacing spikes while the device is functioning ( Figure 9-8 ). If the patient’s heart rate is higher than the device’s programmed rate, no pacer spikes will be seen because the device is not firing (or not needed). Pacer spikes occur at a constant rate. Patients who are atrial-chamber paced will reveal pacer spikes before each P wave, while patients who are ventricular-chamber paced will have pacer spikes before each QRS complex and QRS widening. Lastly, pacers that are dual atrial and ventricular paced will display pacer spikes before each P wave and each QRS complex ( Figure 9-9 ). If the patient has an implanted cardioverter-defibrillator (ICD), an ECG (and the capability to deliver an external defibrillating shock) should be monitored during surgery and transport ( Figure 9-10 ). This is because ICDs are often programmed perioperatively to “no response,” and to respond to external magnet placement.



Noninvasive Blood Pressure Monitoring
The circulatory system is responsible for perfusing organs with blood. Oxygen delivery to, and removal of waste products from, organs must be maintained at all times to sustain life, including during anesthesia.
Blood flow to organs is directly related to pressure gradients and inversely related to vascular resistance. Flow = Pressure/Resistance. Therefore, even if pressure is high, flow may be reduced in the face of elevated resistance. Pressure gradients can be estimated by the difference between mean arterial pressure (MAP) and venous pressure, or, in the case of increased intracerebral pressure (ICP), the difference between MAP and ICP.
The arterial blood pressure is used as a surrogate measure of blood flow and organ perfusion and is comprised of resistance and flow. Thus blood supply to an organ may be low despite adequate blood pressure because of high resistance. Individual organs manifest various degrees of autoregulation, which allows for local changes in resistance to maintain a constant blood flow.
Systolic and diastolic blood pressures are measured by a variety of methods. The pressure created by a contracting heart corresponds to the systolic blood pressure, while the pressure during relaxation corresponds to the diastolic blood pressure. Mean arterial blood pressure is the average arterial blood pressure during systole and diastole; MAP is approximately equal to (2DBP + SBP)/3. It may be either directly measured or calculated from the systolic and diastolic values.
Manual Blood Pressure
Manual blood pressure directly measures the systolic and diastolic blood pressure by auscultation of Korotkoff sounds. The occlusive cuff contains a bladder attached to a tube through which air is entrained with an inflation bulb until a desired pressure is produced. The pressure is often measured with an aneroid manometer. A stethoscope is placed over the occluded artery. The occlusive cuff is inflated above the systolic blood pressure and is slowly deflated (3 to 5 mm Hg/sec) while auscultating for blood flow. The first sound of blood flow corresponds to the systolic blood pressure, while the point at which the sounds diminish reveals the diastolic blood pressure. Auscultation using a stethoscope will detect these Korotkoff sounds. Right- and left-sided, and upper and lower extremity blood pressures should be approximately the same. Both single and twin hose cuffs are available (see below under automated noninvasive blood pressure monitoring) ( Table 9-2 ).
|
|
|
|
Automated Noninvasive Blood Pressure (NIBP)
Automated noninvasive blood pressure is the most common method of noninvasive blood pressure monitoring during anesthesia ( Table 9-3 ). The blood pressure cuff is inflated initially to a preset point (150 mm Hg, or 40 mm Hg above prior measurement) and then incrementally decreased while the transducer senses the pressure oscillation in the cuff with an accuracy of +/− 2%. This method directly measures the mean arterial blood pressure, which correlates to the point of maximum oscillation amplitude, and the systolic and diastolic pressures are estimated by an algorithm (roughly: MAP = (2DBP + SPB)/3. More specifically, the systolic blood pressure is interpreted as the initial detection of rising oscillation, while the diastolic blood pressure is interpreted as the initial detection of falling oscillations ( Table 9-4 ). The automated cuff allows passive participation by the operator and limits operator interpretation by programming the cuff to measure the blood pressure at preset increments of time (e.g., every 5 minutes). NIBP cuffs and/or monitors may contain a single or twin hose system. These systems can be successfully mixed in the case of a mismatched cuff and monitor, as long as a Y-piece adapter is used. Table 9-5 denotes some common problems with automated NIBP monitors. Commonly, the cuff will keep cycling to try and obtain a blood pressure under some of the circumstances listed in Table 9-5 ( Figure 9-11 ). If a blood pressure cannot be determined, error messages may be displayed, such as “pump time-out.”
|
|
|
|
|
|
|
|
|
|
|
|
|
|
|
|
|
|
|
|
|
|
|

Doppler Ultrasound and Direct Palpation to Estimate Blood Pressure
Doppler or palpation uses ultrasound or touch, respectively, in conjunction with an inflatable cuff respectively, to estimate the blood pressure. The systolic pressure correlates to the point during cuff deflation at which first pulse is palpated or Korotkoff sounds are heard. Therefore, these methods entail placing a blood pressure cuff proximal to the artery of interest, and inflating the cuff until the pulse is occluded. The cuff is then gradually deflated until the pulse is appreciated once again. Palpation may also be used to determine approximate systolic blood pressure based on whether the pulse may be palpated at key points: radial artery (~80 mm Hg), femoral artery (~60 mm Hg), or carotid artery (~50 mm Hg). This method provides estimates when the blood pressure is very low. Note that these methods cannot determine diastolic or mean blood pressure, and has the same limitations as the auscultation method.
The Doppler probe works by transmitting ultrasonic waves through the underlying tissue into the flowing blood within the artery, which is then reflected back to the probe. The movement of blood creates a frequency shift, which is also transduced by the probe and can be aurally appreciated. This change between the transmitted and reflected frequencies produces the audible sounds of pulsation. Coupling gel must be placed between the probe and skin to minimize interference from air.
Arterial Tonometry
Arterial tonometry measures beat-to-beat blood pressure in a noninvasive fashion and produces a waveform similar to that of an invasive arterial blood pressure monitor ( Table 9-6 ). Arterial tonometry works by placing numerous independent pressure transducers on the skin over a superficial artery, which overlays bony support, such as the temporal, radial, or dorsalis pedis arteries. The tonometer flattens the artery against the bone without occluding flow ( Figure 9-12 ). Wall tension from the tonometer then acts perpendicular to the blood pressure, such that force measured by transducers at the skin surface reflect the patient’s blood pressure within that artery.
|
|
|
|

Respiratory System
The respiratory system is responsible for oxygen uptake and carbon dioxide removal, and provides a conduit for delivery of anesthetic agents. Mandatory respiratory monitors during general anesthesia include pulse oximetry, capnography, an inspired oxygen analyzer, and a disconnect alarm. In addition, direct visualization of the chest and a precordial or esophageal stethoscope may provide additional clinical information, such as detection of airway obstruction. During regional anesthesia, respiration may be monitored with direct visualization, oximetry, or capnography.
Oxygenation is most easily measured by pulse oximetry. Other methods include qualitative assessment of skin color, transcutaneous oximetry, and arterial blood gas sampling.
Pulse Oximetry
The advent of pulse oximetry in medicine has revolutionized the monitoring of patients, especially that of the anesthetized and critically ill patient ( Figure 9-13 ). It is an extremely important monitor that allows for the continuous measurement of arterial hemoglobin oxygenation ( Table 9-7 ). Oxygenated and deoxygenated hemoglobin absorb light differently at most wavelengths, including the wavelengths of 660 nm (red light) and 960 nm (infrared light) examined by most devices. The Beer-Lambert law allows the concentration of each species to be calculated from the absorption of light at those wavelengths. The ratio of absorption is processed to give the percentage of hemoglobin saturated by oxygen, or the fractional saturation. The sensor has at least two light emitting diodes (960 nm and 660 nm) and a light detector. This sensor may be applied to fingers, toes, earlobes, tongue, or, with a special probe, the nose.

|
|
|
|
|
|
|
|
|
|
|
|
|
|
|
|
|
Normal arterial oxygen saturation, or pulse oximetry (Spo 2 ) ranges in a healthy adult is 96% to 99%, while values above 88% may be acceptable in patients with lung disease. The percentages are associated with audible pitches, which allow the anesthetist to know the patient’s relative level of oxygenation without seeing the monitor. The monitor also provides alarms when saturation falls below a level selected by the user. A high Spo 2 generally indicates that oxygen is available in the lungs from the environment or breathing circuit, taken up in the blood, and delivered to distal tissues. A low Spo 2 implies a problem along the above pathway, or an error in monitoring.
Certain hemoglobinopathies, most notably the presence of carboxyhemoglobin or methemoglobin, can falsely raise or lower the Spo 2 , respectively. This is because carboxyhemoglobin appears like oxyhemoglobin at 660 nm, thus raising the apparent percent of total hemoglobin which is oxygenated. By extension, methemoglobin appears like deoxyhemoglobin at 660 nm, thereby lowering the apparent fraction of deoxyhemoglobin. Co-oximeters exist with a sensor using four or more wavelengths, which can separate a variety of hemoglobin species to calculate a more accurate fractional saturation.
The pulse oximeter detects a pulse plethysmographic waveform ( Figure 9-14 ). Without a clear waveform, the accuracy of the detected Spo 2 should be questioned. The characteristics of this waveform itself may reveal important clinical information, especially when used in conjunction with other monitors. For instance, electrocautery may cause ECG interference, which could be confused with a dangerous cardiac arrhythmia. However, a consistent plethysmographic waveform can easily rule this out. There have been case reports of the plethysmograph diagnosing the degree of airway trapping in obstructive airway disease with pulsus paradoxus, and degree of waveform respiratory oscillations predicting fluid responsiveness in the operating room and ICU.

Some pulse oximetry systems include a signal strength indicator, which can be used to help analyze waveform quality, and therefore assess the validity of the signal and Spo 2 value. For example, the General Electric (GE)/Masimo SET system displays either one, two, or three asterisks, or three dashes, in the display screen to convey the quality/validity of the Spo 2 signal.
End-Tidal CO 2 Monitors
Ventilation is assessed by end-tidal carbon dioxide (Petco 2 ) measurements and spirometry. Capnometry and capnography are often used as synonyms, as both analyze and record carbon dioxide (CO 2 ), with the latter including a waveform. Capnography not only evaluates respiration, but also confirms endotracheal intubation, and is diagnostic for certain pathological conditions. Lastly, mass spectrometry or Raman spectrometry may be employed as a technology to quantify Petco 2 , assuming a sidestream capnometer, rather than a mainstream capnometer, is used.
Method
The measurement of CO 2 , due to its molecular structure, is frequently based on infrared light absorption. Petco 2 can be measured continuously, in real time, to produce waveforms, which will be discussed below. With absorption infrared CO 2 analyzers (a commonly used analyzer), Petco 2 is measured by diverting a gas sample from the patient into a sample chamber. Infrared light is shined through two chambers (a reference/control chamber and a testing chamber), and is sensed by a photodetector. CO 2 absorbs infrared light optimally of a wavelength between roughly 4.2 μm and 4.3 μm. Thus radiation of this wavelength is used. As CO 2 enters the sample chamber, it absorbs the infrared light. With higher concentrations of CO 2 , absorption increases and less infrared radiation reaches the photodetector. With this information, and that from the control chamber, Petco 2 can be calculated by the CO 2 analyzer.
Other than absorption infrared based CO 2 analyzers, photo-acoustic spectroscopy may be used. This device uses a microphone to detect sound produced by the contraction and expansion of the gas sample when it is exposed to pulsatile infrared radiation of an appropriate wavelength. Photo-acoustic spectroscopy allows for less frequent calibration and is faster to detect Petco 2 changes when compared with absorption infrared CO 2 analyzers.
Carbon dioxide may be measured either at the breathing circuit (mainstream capnograph), or via aspiration of gas samples by the capnograph (sidestream capnograph) at a constant rate (from 50 to 250 mL/min), facilitated by a pump, by the above listed CO 2 analyzers. See Tables 9-10 and 9-11 for advantages and disadvantages of each sampling method.
Mass spectroscopy is considered the gold standard expired gas monitor. It determines the amount of a large variety of gases within a sample by firing electrons through it. This imparts a charge (usually positive) onto the sampled gases, and the charged gas moves toward a negatively charged acceleration plate. As the gases travel, they exit through a small hole in the system called a molecular leak, after which the gases are exposed to a magnetic field. This field deflects the path of the gases, whereby gases of lower molecular weight become separated from gases of higher molecular weight. The gases soon reach a photovoltaic detector at different locations, depending on the particle mass, allowing for the identification and calculation of the partial pressure of each gas in the sample. The limitation of this technology is that it must be used with a sidestream sample line, it must be within close proximity of the patient for real-time analysis, and water vapor can collect in their sample line. These machines are also rather expensive, which also limits its practical use.
Raman spectroscopy uses an argon laser with a wavelength of 485 nm, which is fired into the gas sample of the patient. As the gases are exposed to this radiation, they undergo an energy change and frequency shift, which is unique for each gas. Based upon the frequency shift wave number, the spectroscope can calculate the concentration of each gas in the sample in real-time, including volatile anesthetic agents, nitrous oxide, and molecular oxygen. This device requires a sidestream sample line, and consumes a large amount of electrical power. This machine is also very noisy.
Time-Based Versus Volume-Based Capnography
Time-based capnograms are the most commonly used waveforms in the operating room and intensive care unit ( Table 9-8 ). They plot Petco 2 on the y -axis and time on the x -axis ( Figure 9-15 ). A detailed discussion of their interpretation will follow below. It is important to note that volume-based curves may be used and differ from time-based capnograms in several key ways, which allows for the calculation of cardiac output, anatomic dead space volume, physiological dead space fraction, the exhaled CO 2 volume, and even the patient’s metabolic rate if CO 2 is known. Volume-based capnography plots Pco 2 on the y -axis and volume on the x -axis.
|
|
|
|
|
|
|
|
|

Range and Analysis
Normally, Petco 2 is approximately 2 to 5 mm Hg lower than Paco 2 due to dead space in the patient and breathing system. Therefore the ideal range for end-tidal carbon dioxide during general anesthesia is 30 to 40 mm Hg. Due to swallowed gas, esophageal intubation may result in carbon dioxide return similar to that of endotracheal intubation, except that Petco 2 diminishes to zero within a few breaths.
An early sign of malignant hyperthermia is a rapidly rising end-tidal carbon dioxide, especially if it is unresponsive to hyperventilation.
During a period of apnea or prolonged expiration, the measured Petco 2 becomes zero, even though Paco 2 is likely elevated. Providing a breath to the patient will produce another waveform. Sometimes cardiogenic oscillations may be seen on the Petco 2 waveform in this context ( Figure 9-16 ). These are the result of the heart beating and displacing gas from the lungs, which leads to very small amounts of CO 2 being expelled into the sample line. In pressure support ventilation, these oscillations may be mistaken for an attempted patient breath, thus unintentionally triggering the ventilator to provide a breath. These unintentional triggers may be avoided by increasing the threshold for breath delivery, for example, by increasing the degree of negative inspiratory flow required to allow for the delivery of a breath by the ventilator (e.g., from 3 L/min to 4 L/min).

Widening of the β-angle with an elevation of both phase 0/I and III is a sign of inspiratory valve failure. Elevation of both phase 0/I and phase III is a sign of expiratory valve failure or absorbent malfunction. Inspired CO 2 will also rise in the face of absorbent exhaustion.
Alarms may be configured to warn the anesthetist of high or low Petco 2 measurements during inspiration or expiration ( Table 9-9 ). The apnea alarm can also be based on capnography ( Tables 9-10 and 9-11 ) ( Figure 9-17 ).
HIGH PET co 2 |
Increased CO 2 delivery/production |
Malignant hyperthermia, fever, sepsis, seizures, increased metabolic rate or skeletal muscle activity, bicarbonate administration/medication side effect, laparoscopic surgery, clamp/tourniquet release |
Hypoventilation |
COPD, neuromuscular paralysis or dysfunction, CNS depression, metabolic alkalosis (if spontaneously breathing), medication side effect |
Equipment problems |
CO 2 absorbent exhaustion, ventilator leak, rebreathing, malfunctioning inspiratory or expiratory valve |
LOW PET co 2 |
Decreased CO 2 delivery/production |
Hypothermia, hypometabolism, pulmonary hypoperfusion, low cardiac output or arrest, pulmonary artery embolism, hemorrhage, hypotension, hypovolemia, V/Q mismatch or shunt, auto-PEEP, medication side effect |
Hyperventilation |
Pain/anxiety, awareness/”light” anesthesia, metabolic acidosis (if spontaneously breathing), medication side effect |
Equipment problems |
Ventilator disconnection, esophageal intubation, bronchial intubation, complete airway obstruction or apnea, sample line problems (kinks), endotracheal tube or laryngeal mask airway leaks |
MAINSTREAM |
Sensor location is close to the airway |
|
|
|
SIDESTREAM |
|
|
|
|
|
|
MAINSTREAM |
|
|
|
|
|
|
|
|
SIDESTREAM |
|
|
|
|
|
|
|
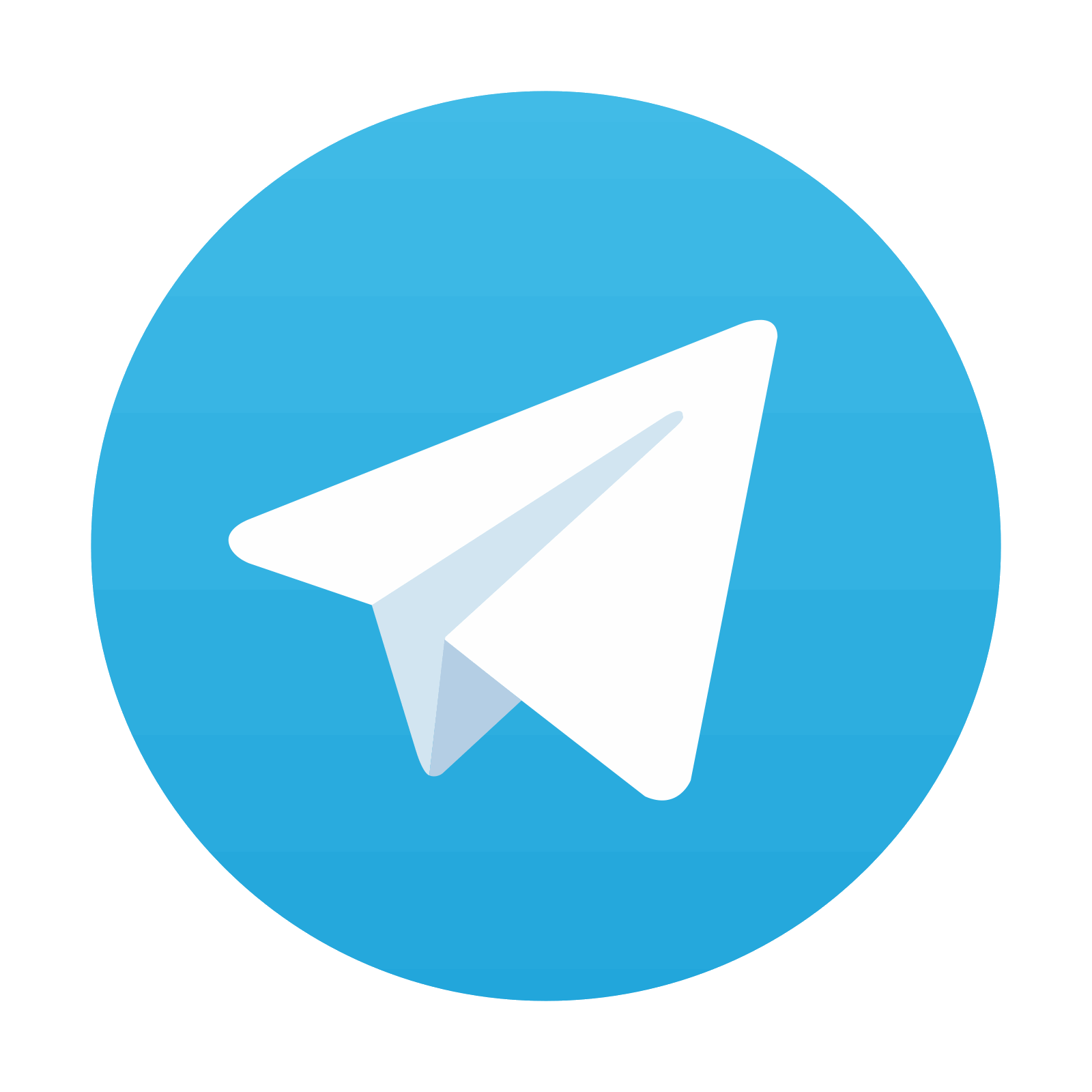
Stay updated, free articles. Join our Telegram channel
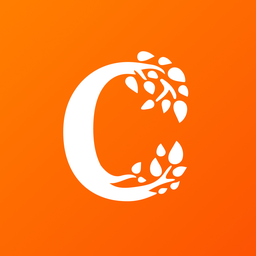
Full access? Get Clinical Tree
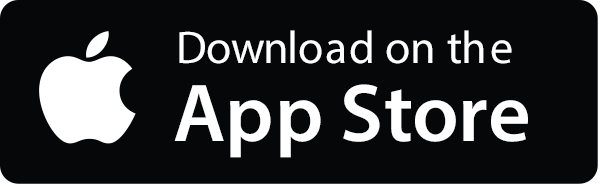
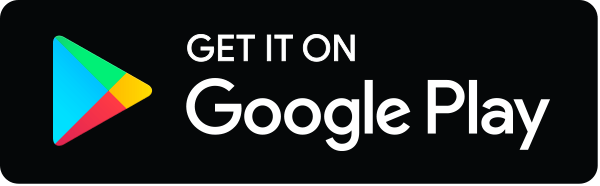
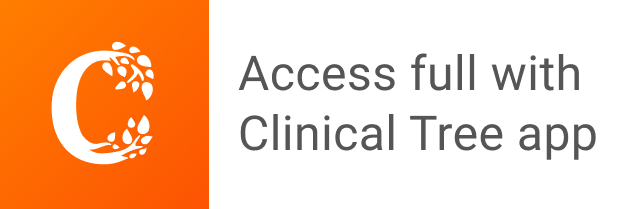